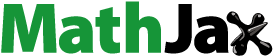
ABSTRACT
Epitaxial graphene on semiconductor films has potential for various applications due to its thermoelectric properties. We investigated factors affecting its thermo power using a solid-state physics approach, considering the interaction between the substrate and graphene, and exploring the effects of chemical potential, temperature, anharmonic vibrations of atoms, phonon-drag, and film thickness. Our results show that anharmonic effects significantly enhance the thermopower caused by electrons, especially at higher temperatures. Additionally, we observed an increase in total thermopower due to phonon-drag, although it has negligible effects at or above room temperature. We found that the thermopower on size-quantized semiconductor films is significantly higher than on metal conductor films and bulk semiconductor substrates. Decreasing the film thickness further increases the thermo power, providing an effective way to enhance the thermo electric properties of epitaxial graphene. Our findings contribute to a better understanding of the thermoelectric properties of epitaxial graphene on semiconductor films and offer valuable insights for future applications.
Introduction
Graphene, a two-dimensional material with unique electronic and mechanical properties, has attracted extensive attention [Citation1]. in recent years due to its potential for various practical applications. One of the most remarkable properties of graphene is its excellent thermoelectric performance, with a thermopower of 30 μV·K−1 at room temperature, which is 3000 times higher than that of metal conductors [Citation1]. As a result, graphene has become a research hotspot in the field of thermoelectric materials, with promising applications in thermoelectric power generation, aerospace probes and industrial waste heat recovery [Citation2].
Previous studies have explored the thermoelectric properties of graphene with different nanostructures and stacking configurations. For instance, Ganguly investigated the thermoelectric properties of graphene nanoribbon with various nanopore structures [Citation3]. Wagner used the semiclassical Boltzmann formalism to compute the transport properties of multilayer graphene, including the electrical conductivity, thermal conductivity and thermopower for Bernal-stacked multilayers with an even number of layers [Citation4]. Moreover, Alisultanov studied the thermoelectric effect in epitaxial graphene formed on semiconductor and metal surfaces, demonstrating that the thermopower of epitaxial graphene is three times higher near the Dirac point near the semiconductor band gap edge and explaining the abnormal increase of thermopower [Citation5,Citation6]. Moreover, previous studies have investigated the contribution of phonon-drag in graphene to thermopower at low temperatures [Citation7,Citation8]. Kubakaddi has also investigated the phonon-drag thermopower, diffusion thermopower and power factor as functions of twist angle theta, temperature and electron density in a twisted bilayer graphene [Citation9]. The theoretical limit of the thermopower contributed by the intrinsic phonons of graphene by dragging electrons has been established, indicating that phonon-drag can reach a thermopower of up to 215 μV·K−1 at 5 K [Citation10]. However, these studies did not specifically calculate the thermopower of epitaxial graphene formed on semiconductor films, nor did they obtain the variation law of thermopower with temperature. Additionally, the anharmonic effect of atomic vibration was not thoroughly considered in most of these studies.
Given the theoretical and practical significance of the thermoelectric transport properties of epitaxial graphene formed on semiconductor films, this study aims to consider the anharmonic vibration of atoms and employ solid-state physics methods to investigate epitaxial graphene formed on silicon films. The investigation will cover the effects of chemical potential, temperature and semiconductor film thickness on thermopower, as well as the influence of atomic anomalous vibration and phonon-drag. By studying the thermoelectric transport properties of epitaxial graphene formed on semiconductor films, this study seeks to contribute to the advancement of thermoelectric materials research.
Methods
Physical model
A monolayer of hexagonally arranged carbon atoms is adsorbed onto the surface of a planar semiconductor film to form a graphene–semiconductor interface [Citation11], as illustrated in . The bond length between adjacent carbon atoms is denoted as d, and the graphene plane is defined as the OXY plane, with the positive vertical direction along the OZ axis.
Figure 1. Top (a) and side (b) views of a semiconductor film-based epitaxial monolayer graphene structure.

Electrons in the substrate of the semiconductor film experience parabolic potential wells in the plane direction but are confined to an infinitely deep potential well in the vertical plane direction. As a result, the electron energy spectrum can be expressed as
where ρ is the density of electronic states of semiconductor substrates, εi is the electron energy of in the Z-direction, which can be determined using the infinitely deep well potential as εi = π2ħ2i2/(2mL2), i is the energy level number, m is the mass of the electron, and L is the thickness of the semiconductor film.
In addition, the interactions between carbon atoms in graphene, as well as between carbon atoms in graphene and the substrate should be taken into account. After considering the short-range interactions between graphene carbon atoms, the atomic interaction energy [Citation11] of a graphene atom can be expressed as
where V1 is the metallization energy, V2 is the covalent energy of the sp2 orbital σ bond of the two atoms for V2 = 3.26×ħ2/(md2). R = 0.154 × 104(ħ2/2m)r010, where r0 is the Bohr radius. β2 is the structural parameter for β2 = 2/3.
Due to thermal vibration, the carbon atoms vibrate anharmonic near the equilibrium position, expand φ(d) near the equilibrium position d0 and deviate from δ = d–d0 by a very small amount, we have
where a0, a1, a2 are the harmonic coefficient, the first and second anharmonic coefficients [Citation12], respectively, which can be obtained by Eq. (1).
Thermopower of semiconductor film-based epitaxial monolayer graphene
The thermopower of semiconductor film-based epitaxial monolayer graphene consists of two components, the thermopower βe resulting from electron transport and the thermopower βph resulting from phonon-drag, with a total thermopower β
The density of electronic states ρ of semiconductor substrates in Eq. (1) can be obtained by
where ρ0 = mS1L1/πħ2. L1 is the distance between carbon atom and the substrate atom in the Z-direction, which is approximately equal to the sum of the radii of the carbon atom and substrate atom, i.e. L1 = rSi + rc. S1 is the amount corresponding to the area of semiconductor film occupied by one carbon atom, which can be obtained by S1 = 3√3d2/4. Δ is the band gap half-width of the semiconductor film substrate, χ is the residual scattering in the substrate, here χ → 0.
The energy level broadening Γc and the movement function Λ(ε) of graphene atoms are caused by the action of the semiconductor film substrate. The relationships between Γc and Λ(ε) and the density of states ρ(ε) of the substrate are determined by
where V is the hybridization potential of graphene.
Considering the anharmonic vibrations of the atoms, the hybridization potential versus temperature [Citation13] is
where V0 is the hybridization potential at equilibrium as V0 = ηħ2/md02, η is a coefficient, αl is the linear expansion coefficient, when 100 K ≤ T < 1500 K, determined by
Thermopower generated by electron transport
The thermopower generated by the electron transport on semiconductor film-based epitaxial graphene is given in Ref [Citation6].
where f is the electron Fermi distribution function, related to the electron chemical potential μ as
where A is the relaxation time, expressed by
The contribution of electrons to the thermopower as a function of chemical potential and temperature as
where (2μ), Γ(2μ) and F(2μ) are the
, Γ and F when the electron energy ε = 2μ.
Thermopower generated by phonon-drag
The existence of the temperature gradient not only changes the electron distribution but also changes the phonon distribution. The electron–phonon interaction affects the relaxation time of the phonon, resulting in a change in the electron distribution and hence in the thermopower. The contribution of this electron–phonon interaction is called the phonon-drag thermopower βph. In Ref [Citation10], using a parabolic quantum well model with quasi-elastic conditions, the phonon-drag thermopower is obtained as
where e and m are the electron charge and effective mass, respectively, s is the phonon sound speed, β0 is the dielectric induction coefficient as β0 = √0.8e14/X, e14 is the piezoelectric constant, X is the electrostatic permittivity. R’ is a parameter determined by the specific form of the electron potential, called the oscillator length, and is related to parabolic potential covariates ω0 by R’ = (ħ/mω0)1/2, and R’ ≈ L/2 with respect to the semiconductor film thickness. l is the mean free path of the phonon and is used as a constant in Ref [Citation10]. σ is the electrical conductivity [Citation14]. ρm is the mass areal density of graphene. I is a mathematical expression as
where , q is the phonon wave vector as q = (4R’k2x2+t’2)1/2, x = q/2k, t’ is the integral variable, k = (kx, ky) is the mode of the electron wave vector, and E1 is the deformation potential. It can be seen from Eq. (19) that
,
at low temperature,
, I ~ constant at high temperature.
The relationship between βph and temperature is
Results and discussion
According to the Refs [Citation13,Citation15,Citation16] and [Citation17,Citation18], the values of physical quantity used in this paper are shown in .
Table 1. Values of physical quantity.
Substituting the above data into Eqs. (6) and (7), the energy level half-width Γc(ε) and the movement function Λ(ε) of adatoms vary with energy ε for different semiconductor film thicknesses are obtained as shown in . Curves a and b show the semiconductor film thicknesses of 6 nm and 10 nm, respectively.
Assuming that there are four energy levels of electrons in the Z-direction, i.e. i = 1, 2, 3 and 4. From Eqs. (13) and (14), taking the temperature T = 300 K, the variation of the thermopower βe with chemical potential and temperature is obtained, as well as the results considering anharmonic effects, as shown in . Curves 0 and 1 represent the result of the harmonic approximation and the result of considering both the first and second anharmonic terms, respectively.
Substituting Eqs. (13) and (15) into Eq. (4) and taking the chemical potential μ = 0.5 eV, the total thermopower β on silicon film-based epitaxial monolayer graphene as a function of temperature and semiconductor film thickness are obtained as shown in . For comparison, also shows βe with temperature when the semiconductor film thickness of 6 nm after considering the anharmonic effect.
Figure 4. Variation of βph with temperature (a), variation of β with temperature (b) and semiconductor film thickness (c).

It can be seen that βe varies nonlinearly with the chemical potential μ, with a peak of 147.4 μV·K−1 near μ = 0.5 eV, indicating that the thermopower of epitaxial monolayer graphene may be abnormally large near the bandgap edge of the semiconductor. βe decreases nonlinearly with increasing temperature. After taking into account the anharmonic effect of atomic vibration, it is larger than that of the harmonic approximation, and the higher the temperature, the larger the difference between them, i.e. the higher the temperature, the more significant the anharmonic effects. For example, the difference of βe between the anharmonic and harmonic approximation is 0.1 μV·K−1 at T = 300 K and 0.18 μV·K−1 at T = 1200 K.
When 100 K ≤ T < 1500 K, βph first decays exponentially and then tends to 0 with increasing temperature, indicating that phonon-drag has little effect on the thermopower at these temperatures. β decreases nonlinearly with increasing temperature and tends to be constant as the temperature continues to increase and decreases nonlinearly with increasing silicon film thickness. For example, when T = 100 K, the thickness L of the silicon film changes from 3 nm to 6 nm and β decreases from 409.6 μV·K−1 to 365.4 μV·K−1, a decrease of 12.84%. When L changes from 7 nm to 10 nm, it decreases from 348.9 μV·K−1 to 335.2 μV·K−1, which is a decrease of 3.93%.
The thermopower β on 3 nm silicon film-based epitaxial monolayer graphene is 409.6 μV·K−1, which is close to the maximum value 400 μV·K−1 at T = 100 K. It is also greater than the thermopower of metal conductor film-based epitaxial graphene and 5.5 times greater than that of semiconductor bulk-based epitaxial graphene seen in . These results suggest that the thermoelectric effect is more significant for epitaxial graphene grown on semiconductor films, and that the effect becomes more pronounced as the thickness of the semiconductor film decreases.
Table 2. Thermopower β of epitaxial monolayer graphene on different substrates.
Conclusions
In this paper, we have studied the thermoelectric transport properties of epitaxial graphene formed on semiconductor films. We obtained the energy-level half-widths of adatoms and the variation of movement function with energy on different semiconductor film thicknesses. By considering the anharmonic effect of atomic vibration, we obtained the variation law of thermopower formed on semiconductor films with temperature, chemical potential and semiconductor film thickness. Our results show that, for the size-quantized semiconductor film substrate, the thermopower of epitaxial graphene is much larger than those on metal conductor film and bulk semiconductor substrates. Furthermore, we found that decreasing the semiconductor film thickness further increases the thermopower, providing an effective way to enhance the thermoelectric properties of epitaxial graphene. These findings provide theoretical guidance for the application of epitaxial graphene in novel thermoelectric nanoelectronic devices.
Supplemental Material
Download MS Word (27.5 KB)Acknowledgments
This work was mainly supported by the Chongqing Natural Science Foundation (No. cstc2020jcyj- msxmX0920), Science and Technology Research Program of Chongqing Municipal Education Commission (No. KJQN202101304), Yongchuan District Natural Science Foundation (No. 2021yc-jckx20045), Science and Technology Research Program of Chongqing Municipal Education Commission (No. HZ2021013).
Disclosure statement
The authors declare that they have no known competing financial interests or personal relationships that could have appeared to influence the work reported in this paper.
Supplemental data
Supplemental data for this article can be accessed online at https://doi.org/10.1080/10667857.2023.2214776
Correction Statement
This article has been republished with minor changes. These changes do not impact the academic content of the article.
Additional information
Funding
References
- Chang PH, Mohammad Saeed Bahramy MS, Nagaosa, N. Giant Thermoelectric Effect in Graphene-Based Topological Insulators with Heavy Adatoms and Nanopores. Nano Letters. 2007;14(7):3779.
- Sharapov SG, Varlamov AA. Anomalous growth of thermoelectric power in gapped graphene. Phys Rev B. 2012;86(3):035430.
- Ganguly S, Maiti SK. Thermoelectricity in graphene nanoribbons: structural effects of nanopores. Micro Nano. 2019;136:106264.
- Wagner G, Nguyen DX, Simon SH. Transport properties of multilayer graphene. Phys Rev B. 2020;101(24):245438.
- Alisultanov ZZ, Mirzegasanova NA. Anomalous increase of thermopower in epitaxial graphene. Tech Phys. 2014;59(10):1562.
- Alisultanov ZZ, Mirzegasanova NA. The thermodynamics of electrons and the thermoelectric transport in epitaxial graphene on the size-quantized films. Physica E Low Dimens Syst Nanostruct. 2015;69:89.
- Zheng RL, Xia JH, Yang WY. Study on thermal and electrical properties of graphene materials. Chengdu: Southwest Jiaotong University Press; 2019.
- Koniakhin SV, Eidelman ED. Phonon drag thermopower in graphene in equipartition regime. Europhys Lett. 2013;103(3):37006.
- Kubakaddi SS. Giant thermopower and power factor in magic angle twisted bilayer graphene at low temperature. J Phys Condens mat. 2021;33(24):245704.
- Hasanov KA, Huseynov JI, Dadashova VV. Effect of Phonon Drag on the Thermopower in a Parabolic Quantum Well. Semiconductors. 2016;50(3):295.
- Davydov SY. Energy of substitution of atoms in the epitaxial graphene-buffer layer-SiC substrate system. Physics of the Solid Stat. 2012;54(4):875.
- Cheng ZF, Zheng RL. Influence of the anharmonic vibration on the Young modulus and the phonon frequency of the graphene. Acta Phys Sin. 2016;65(10):181.
- Gao JH, Zheng RL. Effects of non-harmonic vibration on the thermoelectric properties of semiconductor-based epitaxial graphene. J Sichuan Univ (Nat Sci Ed). 2020;57:1157.
- Alisultanov ZZ. Static conductance of electron gas in epitaxial graphene. Tech Phys Lett. 2013;39(9):758.
- Kittle C. Introduction to Solid State Physics. New York, USA: Wiley; 2004.
- Ye ZQ, Cao BY, Guo ZY. Phonon thermal properties of graphene. Acta Phys Sin. 2014;63:303.
- Ren XX, Kang W, Cheng ZF, et al. Temperature-Dependent Debye Temperature and Specific Capacity of Graphene, Chin. Phys Lett. 2016;33(12):126501.
- Du YS, Kang W, Zheng RL. Study on the change of electrical conductivity and Fermi velocity of epitaxial graphene with temperature. Acta Phys Sin. 2017;66(1):014701.
- Alisultanov ZZ, Mirzegasanova NA. Thermodynamics of electrons in epitaxial graphene. Tech Phys Lett. 2014;40(2):164.