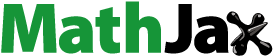
ABSTRACT
The Bi2O4/Bi2WO6 composites were synthesized by one step hydrothermal method, which were characterized by X-ray diffraction (XRD), scanning electron microscopy (SEM), transmission electron microscopy (TEM), Fourier transformed infrared spectroscopy (FT-IR), photoluminescence spectra (PL), X-ray photoelectron spectroscopy (XPS) and UV – Vis diffuse reflectance spectroscopy (DRS). The photocatalytic performance of the composite was tested by degrading methyl orange (MO) under visible light irradiation. The results showed that the Bi2O4/Bi2WO6 composites performed better than pure Bi2O4 and Bi2WO6, whose cycle stability was also greatly improved. Among them, S3 sample (Bi2O4:Bi2WO6 = 16:1) could degrade by about 95% methyl orange (MO) within 50 min, and after three cycles the degradation rate could still reach 74%. The enhanced photocatalytic activity and stability was attributed to the formation of heterojunctions. This work provided a promising composite photocatalyst with high performance for organic pollutant degradation.
GRAPHICAL ABSTRACT

1. Introdution
At present, water pollution resulting from organic pollutants becomes a serious environmental concern. In the treatment of water pollution, photocatalytic technology has received a lot of attention [Citation1,Citation2]. After TiO2 was found to have photocatalytic behaviour, TiO2-based materials have been extensively investigated as photocatalysts [Citation3,Citation4]. However, due to its wide band gap (~3.2 eV), TiO2 can only respond in the ultraviolet region, which accounts for less than 5% of sunlight [Citation5,Citation6]. Therefore, developing new visible light photocatalysts has become the current hot spot.
Recently, some bismuth-containing oxides with narrow band gaps present a wide range of application in photocatalysis, such as BiVO4 [Citation7], Bi2MoO6 [Citation8] and BiOX (X=Cl, Br, I) [Citation9–11]. The bismuth in these photocatalysts all appears as Bi3+. It is known that the 6s2 hybrid orbit of Bi3+can narrow the band gap energy and increase the valence band width by overlapping O2p, which is beneficial to the improvement of photocatalytic performance [Citation12]. In addition, it is reported the empty 6s orbital of Bi5+ ion is also able to improve the band gap structure and endow related compounds with excellent visible light photocatalytic activity [Citation13]. For example, it was reported that NaBiO3 possessed strong absorption under visible light and thus superior activity towards the degradation of organic pollutant [Citation14]. However, few studies have involved bismuth-containing compounds with the mixed valence states so far.
Bi2O4 is a mixed valence oxide of bismuth, which contains Bi3+and Bi5+. It has reported to have a good visible light photocatalytic activity [Citation15]. Nonetheless, the stability of Bi2O4 is not good, since Bi5+ in Bi2O4 tends to Bi3+ under long-term lighting conditions. It is necessary to enhance its stability [Citation16]. It is well known that constructing type-II heterojunctions with other semiconductors is a feasible strategy to improve and photocatalytic performance. Recently, we find that Bi2WO6, as a binary bismuth complex oxide, possesses a narrow bandgap (~2.7 eV), which shows excellent photo-absorption and photocatalytic activity [Citation17]. Therefore, we imagine that the stability and catalytic performance of Bi2O4 might be improved by constructing Bi2O4/Bi2WO6 heterostructure.
Experiment
Materials preparation
All the chemicals were purchased from Sinopharm Chemical Reagent Co., Ltd. (China) and used as received without further purification. The Bi2O4/Bi2WO6 composites were synthesized by one-step hydrothermal method. At room temperature, a certain amount of NaBiO3 and 2 mmol of Bi(NO3)3 and 1 mmol of Na2WO6 were placed in a beaker with 70 ml of water and sonicated for 5 min. Then, the ultrasonically suspended suspension was introduced into a 100 ml Teflon-lined stainless steel autoclave and maintained at 180°C for 6 h. The product was collected by centrifugation, washed for three times with distilled water, and then dried at 80°C for 12 h. In this experiment, the amount of NaBiO3 was 8 mmol, 16 mmol, 32 mmol and 64 mmol, and the corresponding composite samples were called S1, S2, S3 and S4. Correspondingly, pure Bi2O4 was produced by NaBiO3 with hydrothermal, and pure Bi2WO6 was made by Bi(NO3)3 and Na2WO6 with hydrothermal method.
Characterization
The phase structure tests were performed by an X-ray diffractometer (XRD, Bruker D8 Advance) using Cu-Kα radiation. The morphology of the material was characterized by field emission scanning electron microscopy (SEM, Quanta 250) and transmission electron microscopy (TEM, Tecnai G2 F20). Chemical mapping was achieved using energy-dispersive X-ray detector and scanning electron microscopy modules. X-ray photoelectron spectroscopy (XPS: Thermo ESCALAB250, U.S.A) was used to detect the surface properties of the sample. The optical properties of the samples were obtained using UV–vis diffuse reflectance spectroscopy (DRS, Varian Cary 300) and photoluminescence spectroscopy (PL, Varian Cary-Eclipse 500).
Photocatalytic activity tests
The photocatalytic activity of Bi2O4/Bi2WO6 composites was tested under the irradiation of visible light with methyl orange (MO) aqueous solution. In the experimental measurement, a 150 W xenon lamp with a cut-off filter of 400 nm was used as a light source for visible light, and its light intensity is about 0.25mWcm−2. The photocatalytic activity of the experimental process is as follows: 0.05 g Bi2O4/Bi2WO6 composite sample was added 50 mL 20 mg/L MO solution(pH ≈ 6.8). Before using visible light irradiation, the suspension is placed in a dark environment and stirred for about 10 min to establish an equilibrium of adsorption/desorption. Then, take samples every 20 min for 2 h, 3 ml each and centrifuge. Finally, the sample was tested on a UV–visible spectrophotometer to analyse the change of concentration of MO, wherein the special wavelength of MO was set at 464 nm.
Photoelectrochemical measurement
On the electrochemical workstation (CHI660B, China), photoelectrochemical measurements were carried out using a standard three-electrode where platinum electrode (1 × 1 cm2) is as a counter electrode and a saturated calomel electrode (SCE) is as a reference electrode. A 0.5 M Na2SO4 solution was used as electrolyte. The composite electrode of Bi2O4/Bi2WO6 was uniformly coated on 1 cm × 1 cm tin fluoride oxide glass (FTO) to prepare the working electrode.
Results and discussion
Structural and morphology characterization
The purity and crystallization phase of Bi2O4, Bi2WO6 and their composites with different proportions were tested by XRD (). The diffraction peaks of Bi2O4 match well with the monoclinic phase of Bi2O4 (JCPDS card number 50–0864), while all the diffraction peaks of Bi2WO6 can be indexed to the orthorhombic phase (JCPDS card number 39–0256). This shows that pure samples were successfully synthesized. The XRD patterns of S1, S2, S3 and S4 sample present the superposition of the diffractive peaks of the two components, indicating that the obtained catalyst is indeed composed of Bi2O4 and Bi2WO6. As the composite ratio increases, the peak intensity of Bi2O4 gradually increases, suggesting the increase of the content of Bi2O4 in the sample. The relative mass fraction of Bi2WO6 in Bi2O4/Bi2WO6 composites can be estimated according to Equationequation (1)(1)
(1) :
where W1 is the mass fraction of Bi2WO6, I1 and I2 are the strongest integral intensities of Bi2WO6 and Bi2O4, respectively. RIR1 and RIR2 values are obtained from MDI Jade 5.0. By calculation, the content of Bi2WO6 is estimated as 45.6%, 21.4%, 12.7% and 5.9% in the S1, S2, S3 and S4 sample, respectively.
The morphology of the composite samples can be obtained by SEM techniques. As shown in , pure Bi2O4 shows an irregular rod-like morphology with a length of about 2 µm and a diameter of about 0.5 µm. In contrast, shows the morphology of pure Bi2WO6. It can be seen that pure Bi2WO6 generally shows a coherent sheet-like structure. As shown in , there are almost basically short sticks like the morphology of pure Bi2O4. However, the difference is that the length of the rod becomes shorter, which is about 500 nm. It is very likely that a small amount of Bi2WO6 has an effect on the morphology of the sample. In , it can be seen that S3 appears has less rod morphology, which tends to degenerate into fragmented floccules. In , the rod-shaped Bi2O4 crystals further shorten, which tend to stick together. As for , it can be seen that there is a combination of rod-like and small pieces, and a large number of pieces are attached to the surface of fragmented rod.
In order to study the elemental distribution of Bi2O4/Bi2WO6 composites, the EDS elemental analysis of S3 was carried out. As shown in , three elements of W, O and Bi were detected in the sample, which were evenly distributed, suggesting that Bi2O4 and Bi2WO6 were uniformly grown together.
Figure 3. Surface scan image of S3 (a) Surface scan image (b) O element (c) W element (d) Bi element.

Sample S3 was further characterized by TEM. As shown in , the shape of the sample is generally rod-shaped, with some small particles attached to the rod. We can clearly see the complex shape of rods and pieces in , in which the pieces are tightly attached to the surfaces of rods. The TEM results are well consistent with SEM.
XPS spectra were used to identify the elemental composition and chemical state of composite sample S3, pure Bi2O4 and Bi2WO6. All spectra were compared with C1s at 284.8 eV. As shown in , full-scan XPS spectra show that O, Bi and W are indeed present in the sample. shows the Bi4f spectrum of Bi2O4, Bi2WO6 and sample S3. Among them, the peaks of Bi2WO6 at binding energies of 158.7 eV and 164 eV can be attributed to the characteristic spin-orbit splitting of Bi4f5/2 and Bi4f7/2 of Bi3+ [Citation18]. However, Bi2O4 can be decomposed into two doublets at binding energies of 158.2 eV and 158.6 eV (or 163.5 eV and 163.9 eV), and the corresponding sample S3 also has binding energy of 158.4 eV and 158.7 eV (or 163.6 eV and 164 eV ) are decomposed into two peaks, which corresponds exactly to the two valence states of Bi(III) and Bi(V) [Citation19]. is a high resolution XPS spectrum of W4f of Bi2WO6 and sample S3. Among them, the two main peaks at 35 eV and 37.1 eV are W4f5/2 and W4f7/2 of W6+ ions in Bi2WO6. . is the XPS spectrum of O1s. For pure Bi2O4, the two peaks at 529.1 eV and 531.1 eV (and the two peaks in 529.8 eV and 531.7 eV in pure Bi2WO6) can correspond to the lattice oxygen (Bi-O) and hydroxyl oxygen [Citation15]. The binding energy of O in sample S3 synthesized by hydrothermal one step is between two pure samples and should have some coupling effect. Overall, the XPS analysis results can be compared with the XRD analysis results, which proves that we have indeed synthesized Bi2O4/Bi2WO6 composites.
We further tested the optical properties of the composite samples while also testing the optical properties of pure Bi2WO6 and pure Bi2O4. As shown in , the absorption edges of pure Bi2WO6 and Bi2O4 are approximately 475 nm and 740 nm, which correspond to a band gap of 2.61 eV and 1.68 eV (Eg ≈ 1240/λ), respectively. The absorption edges of the composite samples S1, S2, S3 and S4 are significantly larger than the pure Bi2WO6 and less than Bi2O4. Among these, the absorption edge of the sample S3 is the largest, which can reach 630 nm, and the forbidden band width is approximately 1.97 eV. UV spectrum clearly shows that with the increase of the composite ratio, the absorption edge first increases and then decreases, and all of them can well response to visible light.
Photocatalytic performance
In order to test the photocatalytic activity of the composite sample, a series of photocatalytic degradation experiments were performed to degrade the typical organic dye MO, as shown in . First of all, a blank experiment was carried out, and it was found that the photolysis of pure MO solution is negligible in the absence of photocatalyst. In contrast, pure Bi2O4 and Bi2WO6 were able to remove 77.0% and 28.8% of the MO after 120 min of visible light irradiation. After Bi2O4 and Bi2WO6 were recombined, the photocatalytic activity was greatly improved. With the increasing of Bi2WO6 content, the photocatalytic activity of the composite sample increases first and then decreases. The MO degradation rates are 77.3%, 89.3%, 95.2% and 91.9% for S1, S2, S3 and S4, respectively, in which S3 sample show the best photocatalytic activity. The MO degradation rates over S3 is comparable to or higher than those of reported Bi-containing compounds [Citation20]. Generally speaking, the specific surface has obvious effect on the photocatalytic activity. However, the specific surface areas are measured as 4.5, 7.3, 5.8, 5.3, 4.9 and 12.5 m2/g for Bi2O4, S1, S2, S3, S4 and Bi2WO6, respectively. S3 sample possesses moderate surface area but best activity. Therefore, the surface area is not a key factor for the improved activity. The improved photocatalytic activity may be ascribed to the heterojunction effect between Bi2O4 and Bi2WO6, which helps to restrain the recombination of photo-generated electrons and holes.
The stability of photocatalyst is of great significance for practical production applications. The recycling runs of MO degradation over S3 sample and Bi2O4 were performed to test their stability. As shown in and (b), the degradation efficiency of Bi2O4 decreased from 77% to 31% after three cycles, while the degradation efficiency of S3 sample decreased from 95% to 74% after three cycles. Therefore, Bi2WO6 modified Bi2O4 exhibits excellent stability, which is superior to some reported photocatalysts [Citation21].
Mechanism
PL and photocurrent
In general, the separation efficiency and migration rate of photogenerated electrons and holes play a very important role which affects the photocatalytic activity of photocatalysts. We can use the photoluminescence spectra (PL) to qualitatively research their recombination processes. The PL signal is mainly derived from the radiative recombination process of self-trapped excitations. The lower the PL intensity, the smaller the probability of the photogenerated electron – hole pairs recombination. As shown in , the PL emission intensity of sample S3 is much lower than that of Bi2WO6 and Bi2O4, which means that constructing Bi2O4/Bi2WO6 heterostructure can inhibit the recombination of photogenerated electrons and holes, favouring the enhancement of the photocatalytic efficiency. To further confirm this, the I-t curve was also measured by visible light switching illumination on S3, Bi2WO6 and Bi2O4 samples. As shown in , all samples exhibited a significant photocurrent response when the lamp was turned on. Compared to Bi2WO6 and Bi2O4, S3 samples produced higher current densities, indicating that photogenerated electron-holes have a high mobility and a low recombination rate. Therefore, we can confirm that the composite sample S3 effectively inhibits the recombination of photogenerated electrons and holes.
Main active species
In photocatalytic experiments, the three active species that typically cause photocatalytic degradation of organic matter are superoxide radicals (·O2−), hydroxyl radicals (−OH), and active holes (h+) [Citation22]. In order to explain the photocatalytic reaction mechanism, it is necessary to find the main active species of MO photodegradation. In this experiment, samples isopropanol (IPA), benzoquinone (BQ) and triethanolamine (TEOA) [Citation23] were used as scavengers for the three active species. We conducted a series of experiments to test the effect of these active species on the photodegradation rate of MO. As shown in , using IPA to test the active species -OH, there was no decrease in the photodegradation rate of the MO, indicating that there was no -OH effect during the reaction. The addition of TEOA to the sample solution has a certain decrease in the photodegradation rate of MO, which indicates that there are h+ active radicals during photodegradation. After the addition of BQ, the photodegradation rate of MO decreased significantly, indicating that ·O2− plays a major role in photodegradation. A large number of documents indicate that -OH is not present in Bi-based photocatalysts because the reduction potential of Bi(V)/Bi(III) is more negative than the standard·OH/OH- redox potential - (+2.38 eV). The results of these tests show that the active free radicals of the composite photocatalyst Bi2O4/Bi2WO6 when photodegraded are ·O2− and h+. The electrons and holes generated by the light, the electrons migrate to the surface of the catalyst to form oxygen radicals with oxygen, and the holes directly interact with the degradants.
XPS valence band spectrum
In order to further analyse the separation of photoelectrons-holes and the photocatalytic process, it is necessary to understand the band arrangement of Bi2O4/Bi2WO6. As shown in , the resulting valence band edges are approximately 0.9 eV and 2.45 eV for Bi2O4 and Bi2WO6, respectively. Considered that the band gap of Bi2O4 and Bi2WO6 is about 1.68 eV and 2.61 eV, their conduction bandedges are −0.78 eV and −0.19 eV. Obviously, the band potentials of Bi2O4 and Bi2WO6 take on a staggered band alignment, which helps to improve the separation and transfer of photogenerated carriers due to the energy bias.
Photocatalytic mechanism
According to the experimental results and reference energy band theory, we can simply analyse the catalytic mechanism of Bi2O4/Bi2WO6 composite photocatalyst. The band gap of Bi2O4 is relatively narrow, about 1.68 eV, and can be excited to generate electron–hole pairs under visible light irradiation. As shown in , since the CB position of Bi2O4 is higher than that of Bi2WO6, the photo-induced electrons of Bi2O4 migrate to the conduction band of Bi2WO6 under the irradiation of visible light, while the Bi2O4 receives the signal from Bi2WO6 at the VB position of Bi2O4. The photogenerated holes transferred from VB greatly promote the separation and transfer of photogenerated carriers and extend the lifetime of photogenerated carriers. The electrons accumulated in the CB of Bi2WO6 capture dissolved oxygen to generate active species ·O2− radicals, while the holes on VB of Bi2O4 directly degrade MO under irradiation with visible light. Therefore, the catalytic activity of the photocatalyst is greatly improved.
Conclusion
In summary, a Bi2O4/Bi2WO6 composites were successfully synthesized via a hydrothermal method. The composite exhibited improved photocatalytic activity towards the degradation of methyl orange (MO) under visible light irradiation. Especially, the S3 sample could remove 95.2% of MO in 120 min, suggesting the best activity. The improved activity and stability was attributed to the heterojunction effect between Bi2O4 and Bi2WO6, which strengthen effective separation of photo-generated charge carriers. Besides, the trapping experiments exhibited that ·O2− and h+ radicals are the main active species in the photocatalytic degradation of MO.
Acknowledgments
This work was supported by Fundamental Research Funds for the Scientific Research Project of Jiangxi Provincial Education Department of China (GJJ2206602, GJJ217002).
Disclosure statement
No potential conflict of interest was reported by the authors.
Additional information
Funding
References
- Liu Y, Peng C, Cai Y, et al. Hydrothermal preparation and photocatalytic properties of visible light driven AgBr/BiVO4 nanocomposite. Mater Technol. 2022;37(2):104–9. doi: 10.1080/10667857.2020.1816040
- Wu G, Xing W. Fabrication of ternary visible-light-driven semiconductor photocatalyst and its effective photocatalytic performance. Mater Technol. 2019;34(5):292–300. doi: 10.1080/10667857.2018.1553267
- Nakata K, Fujishima A. TiO2 photocatalysis: design and applications. J Photochem Photobiol C Photochem Rev. 2012;13(3):169–189. doi: 10.1016/j.jphotochemrev.2012.06.001
- He Y, He Q, Liu Z, et al. Controllable preparation and improved performance of TiO2 photocatalysts with various structures. Mater Technol. 2019;35(24):1–10. doi: 10.1080/10667857.2019.1644038
- Beldamarco S, Lillorodenas MA. H2 production by cellulose photoreforming with TiO2-Cu photocatalysts bearing different Cu species. 2022. doi: 10.1016/j.cattod.2022.11.006
- Shu R, Lin B, Wang C, et al. Upgrading phenolic compounds and bio-oil through hydrodeoxygenation using highly dispersed Pt/TiO2 catalyst. Fuel. 2019;239:1083–1090. doi: 10.1016/j.fuel.2018.11.107
- Wang X, Shen Y, Zuo G, et al. Influence of heat treatment on photocatalytic performance of BiVO4 synthesised by sol-gel method. Mater Technol. 2016;31(3):176–180. doi: 10.1179/1753555715Y.0000000034
- Peifang W. A one-pot method for the preparation of graphene–Bi2MoO6 hybrid photocatalysts that are responsive to visible-light and have excellent photocatalytic activity in the degradation of organic pollutants. Carbon An International Journal Sponsored By The American Carbon Society. 2012;50:5256–5264. doi: 10.1016/j.carbon.2012.06.063
- Wang Y, Liu XM, Chen QY, et al. Simultaneous photocatalytic oxidation and adsorption for efficient As(III) removal by magnetic BiOI/γ-Fe2O3 core–shell nanoparticles. Mater Today Chem. 2022;24:100823. doi: 10.1016/j.mtchem.2022.100823
- Wang X, Liu X, Liu G, et al. Rapid synthesis of BiOCl graded microspheres with highly exposed (110) facets and oxygen vacancies at room temperature to enhance visible light photocatalytic activity. Catal Commun. 2019;130:105769. doi: 10.1016/j.catcom.2019.105769
- Liu G, Chen Y, Liu X, et al. BiOCl microspheres with controllable oxygen vacancies: synthesis and their enhanced photocatalytic performance. Journal Of Solid State Chemistry France. 2022;306:306. doi: 10.1016/j.jssc.2021.122751
- Liu Z, Lv F, Xiao Y, et al. Morphology-controllable synthesis of BiOBr architectures and their visible light photocatalytic activities. Mater Technol. 2019;34(11):1–6. doi: 10.1080/10667857.2019.1615274
- Yun HN, Iwase A, Kudo A, et al. Reducing Graphene Oxide on a Visible-Light BiVO4 Photocatalyst for an Enhanced Photoelectrochemical Water Splitting. J Phys Chem Lett. 2010;1(17):2607–2612. doi: 10.1021/jz100978u
- Ding Y, Zhu L, Yang F. Bi3+ self doped NaBiO3 nanosheets: facile controlled synthesis and enhanced visible light photocatalytic activity. Applied Catalysis, B Environmental. 2015;164:151–158. doi: 10.1016/j.apcatb.2014.09.019
- Y WH, S LZ, T GL, et al. Novel Bi2O4 /BiOBr heterojunction photocatalysts: in-situ preparation, photocatalytic activity and mechanism. Mater Sci Semicond Process. 2018;77:8–15. doi: 10.1016/j.mssp.2017.12.016
- S LZ, Ran HS, N NJ, et al. One-pot synthesis of Bismuth Oxyhalide/Oxygen-rich bismuth oxyhalide Heterojunction and its photocatalytic activity. J Colloid Interface Sci. 2014;431:187–193. doi: 10.1016/j.jcis.2014.06.020
- Wang S, Yang H, Hu G, et al. Synthesis and enhanced photocatalytic activity of Cu(OH)2 cluster modified Bi2WO6 toward RhB degradation. Mater Technol. 2018;33(8):1–11. doi: 10.1080/10667857.2018.1475141
- Zhou L, A PE, H RM, et al. Fe substitutions improve spectral response of Bi2WO6-Based Photoanodes. ACS Appl Energy Mater. 2022;5(12):15333–15344. doi: 10.1021/acsaem.2c02964
- Yang R, Qin F, Zheng S, et al. Fabrication of three-dimensional hierarchical BiOBr/Bi2O4 p–n heterojunction with excellent visible light photodegradation performance for 4-chlorophenol. J Phys Chem Solids. 2022;2022(161):110381. doi: 10.1016/j.jpcs.2021.110381
- V RC, N RI, Koutavarapu R, et al. Novel BiVO4 nanostructures for environmental remediation, enhanced photoelectrocatalytic water oxidation and electrochemical energy storage performance. Solar Energy. 2020;207:441–449. doi: 10.1016/j.solener.2020.06.075
- Zhang Y, X WW, S GY, et al. Hydrothermal synthesis of Bi2O4/NaBiO3 heterostructures with enhanced visible light photocatalytic properties - ScienceDirect. J Phys Chem Solids. 2020;149:149. doi: 10.1016/j.jpcs.2020.109766
- Wang Y, Zhang Y, C ZT, et al. Removal of Trace Arsenite through Simultaneous Photocatalytic Oxidation and Adsorption by Magnetic Fe3O4@PpPDA@TiO2 Core-Shell Nanoparticles. ACS Appl Nano Mater. 2020;3(8):8495–8504. doi: 10.1021/acsanm.0c02083
- Prasenjit K, Shukla K, Jain P, et al. An activated carbon fiber supported Fe2O3@bismuth carbonate heterojunction for enhanced visible light degradation of emerging pharmaceutical pollutants. React Chem Eng. 2021;6:2029–2041. doi: 10.1039/D1RE00250C