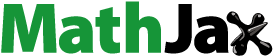
ABSTRACT
The characteristics and performance of perovskites are adversely impacted by the presence of a Sn metal at high-temperature conditions. In this study, CASTEP analysis and density functional theory are used to examine the impact of 1-butyl-3-methylimidazolium bromine ionic liquid in enhancing the features of Pb-Sn halide perovskite alloys. According to the study’s findings, the ionic liquid device has better electronic, optical, mechanical, and thermodynamic properties than the control sample because it can anchor the MA cation through hydrogen bonding and can reduce the grain boundaries of the perovskite film, making it tolerable up to high temperatures above 80°C. In comparison to the control device, the ionic liquid perovskites’ photovoltaic characteristics were also improved. This research lays the way for the development of Pb-Sn alloys with enhanced characteristics that are likely to result in the development of stable and effective lead-free perovskite solar cells.
Introduction
For effective solar energy-conversion applications, a potential absorber material is anticipated to possess several essential properties, including an appropriate direct energy band gap in the visible range, a high solar radiation absorption coefficient, high charge carrier mobility, and a high solar to electrical energy conversion efficiency [Citation1] [Citation2]. Additionally, they must be stable at high ambient temperatures in a humid environment. A new class of lead-based hybrid halide perovskite absorber materials has been evolved, and these materials have demonstrated intriguing characteristics for optoelectronic applications. Despite the lead-based halide perovskites’ low cost and excellent solar power conversion efficiency which is now 26.1% [Citation3,Citation4], their instability in the presence of heat, sunlight, and moisture poses significant obstacles to their use in commercial applications [Citation5]. Scientists have investigated into alternative metals such as Sn, Ge2+, Cu, Mn, Fe, Co, and Ni to solve these drawbacks [Citation2,Citation6]. Among the proposed metals, Sn has received the greatest attention in the quest to replace Pb-due to their identical electronic configuration and the existence of a single pair of electrons in the s orbital [Citation2,Citation7]. It has been shown that raising the content of Sn in Pb-based perovskites reduces the band gap and moves the electronic absorption spectra to a long wavelength region of around 1060 nm [Citation8]. This makes them ideal for creating tandem perovskite solar cells, which may be able to surpass the Shockley–Queisser limit. The development of Pb-Sn perovskites is currently hampered by issues such as the rapid crystallization of SnI2 and MAI, the poor quality of the perovskite films, ineffective charge transport, and severe charge recombination [Citation9]. This has an impact on the materials’ characteristics and optoelectronic parameters. To overcome the problems with Pb-Sn perovskites, several theoretical investigations have recently been conducted [Citation10–13]. However, only a small number of research have simultaneously published on the characteristics and optoelectronic performance of MAPb0.5Sn0.5I3 perovskites, leaving their properties unclear [Citation8].
In this study, the characteristics and optoelectronic performance of MAPb0.5Sn0.5I3 perovskites were further investigated. Density Functional Theory (DFT) was used to determine the concentration of 1-butyl-3-methylimidazolium bromine (BMIBr) ionic liquid (IL) in assistance with the CASTEP analysis from the Materials Studio. However, an optimal concentration of IL is required for the design of perovskites with ILs additive since high concentrations of IL may exhibit enhanced hysteresis and an asymmetrical eclipse shape in the J-V curve [Citation14,Citation15]. In essence, more positive ions would result in more electron trapping centres which may act as different sorts of defects in the devices, thereby affecting the properties of the device [Citation16]. This necessitates the use of DFT calculations with the assistance of CASTPE analysis providing a theoretical baseline towards enhancing the properties of perovskite materials before experiments are implemented which is always expensive and time-consuming. The IL was chosen because of its exceptionally low vapour pressure, outstanding electrical conductivity, good solubility, low toxicity, non-flammable and thermally stable, reducing the chance of acute exposure and pollution due to evaporation [Citation14,Citation17,Citation18]. It has also never been applied to Pb-Sn perovskite solar cells. BMIBr in perovskite films would interact with the perovskite rather than being absorbed into the perovskite lattice. Its functions include the anchoring of MA molecules via the creation of hydrogen bonds between BMI and MA, the inhibition of perovskite film breakdown, and the stimulation of crystal development, which minimizes grain boundaries and quality of the film where MA would rapidly decay which contributed to the improved properties of perovskite materials.
Methodology
The perovskites’ computations were performed through DFT and CASTEP analysis available in Materials Studio [Citation10,Citation19,Citation20]. The slabs for simulations were produced by changing the amounts of IL additives in the Crystallization Information Files (CIF) from the Crystallography Open Database (COD), which were 0.5%, 1.5%, and 3%, respectively, as shown in [Citation21–24]. The ultrasoft pseudopotential corresponding to an exchange correlation (XC) functional developed by Perdew, Burke, and Ernzerhof (PBE) has been used throughout the calculation. The atomic locations are optimized with a cut-off energy of 500 eV until the force reaches below 0.04 eV Å−1. The cut-off energy and force parameters employed in this investigation are equivalent to those from earlier theoretical studies [Citation25]. The structures were optimized using 3 × 2 × 3 Γ -centred grids for k-point sampling, which are believed to be sufficient for the convergence of total energy within 1 meV [Citation8,Citation26,Citation27]. The following formulas were used to compute the optical parameters such as dielectric function, refractive index, and absorption coefficient [Citation20,Citation28].
where h – Planck’s constant, c – velocity of light, λ – frequency, I – intensity of light, ε1(ω) and ε2(ω) – dielectric functions real and imaginary parts, ω – frequency, P – the principal value, T – temperature and q – elementary charge, e – electron, Ω – cell volume, u – the incident electric field’s polarization vector, and
– conduction (CB) and valence band (VB) electrons’ wave functions, n – refractive index, α – absorption coefficient,
– photon energy,
– Boltzmann constant.
Results & discussions
Structural properties
Orthorhombic phases were discovered to exist in both control and IL-added perovskites at normal temperature. In contrast to the IL-added perovskite, which maintained the initial phase structures as shown in , the control structure underwent an isostructural transition above 60°C similar to other related studies of the control sample [Citation8]. This is due to the fact that IL does not absorb into the perovskite lattice but instead interacts with the perovskite, strengthening the hydrogen bonds between BMI and MA and reducing the grain boundaries and crystal surfaces that are induced by crystal growth [Citation29]. The lattice volume steadily increased after the addition of IL. This resulted from the crystal lattice’s enlargement as a result of the increased size of IL [Citation15]. The total energy of a unit cell was calculated during the optimization process with the corresponding volumes as well as the lattice parameters, as shown in . The results are in good agreement with the experimental findings when compared with the findings of other studies [Citation7,Citation8,Citation30,Citation31].
Table 1. Hybrid perovskite lattice parameters and volume (V) at room temperature.
Table 2. Phase structure at temperatures above 60°C.
Electronic properties
Direct band gap materials were shown by the estimated electronic characteristics of the control and IL-added perovskites. They have Brillouin zone Γ- points separating their valence band maxima and conduction band minima, the predicted energy band gap of the control sample is 1.26 eV, which is in agreement with experimental and other theoretical findings [Citation25,Citation30]. The estimated band gap makes it evident that when IL is added, the band gap shrinks due to an increase in atomic size, as shown in making the perovskite essential towards fabrication. This is brought on by the larger atomic size of IL, which results in a reduction in the nuclei’s electrostatic attractive force on the electrons in the outermost shell. The bonding energy between CB and VB is reduced because the nucleus no longer has much control over the electrons in the outermost shell. As the electrons occupy positions close to the Fermi level, the energy gap between the VB and CB eventually narrows and the VB moves up to the Fermi level.
The nature of the materials is described by the total density of states (TDOS), which is depicted in . The figures demonstrate that these materials have semiconducting characteristics. The figures show that as IL is added, the valence and conduction bands move closer to the Fermi level, indicating a decrease in the band gap energy. The figures also demonstrate how atoms, and their various states affect the band gap energy of compounds. Pb and Sn make up most of the valence band, while the I atom dominates the conduction band. The p-orbitals of the I, MA, and IL atoms make up most of the contribution at the Fermi level. TDOS with the MA and IL atom levels were aligned, which reduced the variability in the Pb or Sn circumstances, to evaluate the energy levels of mixed metal perovskites. This is due to the weak interaction that the IL/MA is projected to have with the inorganic cavities. This is consistent with the variability in the bandgap, and the findings are in agreement with earlier studies [Citation30,Citation32].
Optical properties
The materials’ reaction to the incident photons is described by the optical parameters [Citation33]. The dielectric functions and absorption coefficient are discussed in this study to characterize the characteristics of materials regarding light absorption. The real and imaginary components of the dielectric functions, respectively, describe the energy gain of the photovoltaic materials, the energy absorption capacity and behaviour of these materials, and the stored energy of the material that is ready to be released [Citation34].
shows the calculated static dielectric constant of the control sample is 3.7, which is in good agreement with the results from prior investigations [Citation34]. This constant gradually rises to 5.68 with IL incorporation of 0.5%, then rises to 5.85 at 1.5%, and ultimately decreases to 3.43 with IL incorporation of 3%. This fluctuation can be attributed to the occurrence of shallow impurity bands, which are primarily controlled by structural factors such as particle size, crystallinity, and orientation [Citation33]. Therefore, the large grain size of the IL-added perovskites at 0.5% and 1.5% is what causes the increase in dielectric constant, whereas at 3%, the large structural alteration reduced the polarization, which agrees with the other observations [Citation35]. The larger polarons that are formed by the higher dielectric value reduce charge traps and enhance charge transport [Citation36], lower the exciton binding energy, and ultimately lead to more free charge carriers for efficient charge separation in solar cells [Citation33]. This means that adding IL at low concentrations enhances charge separation and transportation by improving binding energy and lowering defect density. In response to an increase in energy, dielectric constants rise for all materials and peaks between 1.21 and 1.24 eV, exhibiting maximum dispersion. As a result of the alignment of dipoles in the opposite direction from the direction that the light wave is moving, which indicates the reflection of all incident electromagnetic radiation, the values then drop to negative values in the energy range of roughly 1.4–1.6 eV [Citation34].
The imaginary part describes how the incident light wave is attenuated as it passes through the material. This attenuation may be the result of energy lost inside the material and appearing as reflected waves. With the addition of IL, the computed imaginary part in depicts a red shift in the absorption edges, which is consistent with the narrowing of the band gaps. Previous experimental and theoretical studies found the peak for the control at 1.26 eV which is in good agreement with the findings of this study [Citation37]. Additionally, the peak is redshifted for IL additions of 0.5% and 1.5%, whereas a much smaller peak at 1.21 eV is present for IL addition of 3%.
The absorption of control and IL-added devices is depicted in . The absorption of the IL-added perovskite materials is greater than that of the control at wavelengths between 200 and 300 nm. Additionally, as demonstrated in , their light absorption is also significant in the long-wavelength range between 600 and 1000 nm. These mixed perovskites have great visible light absorption, good stability, and a decrease in hazardous Pb, making Pb-Sn halide a promising absorbing layer for perovskite solar cells.
Mechanical properties
Due to mechanical stress during the manufacturing process as well as age, solar cells frequently develop microscopic fractures. This malfunctioning results in a decreased current density, which reduces the performance and lifespan of the cell [Citation33]. The mechanical stability of a solar cell under various circumstances is becoming an increasing source of worry as solar cells have recently become much thinner and more portable. Mechanical characteristics are crucial for the effective operation of perovskite solar cells.
Stiffness or constants of elasticity Cij are the parameters that reveal a material’s resistance to deformation in various directions when subjected to shearing, volumetric, and uniaxial stresses. The mechanical properties of these materials can also be controlled by these constants during production and use.
The CASTEP stress-strain method is used in this investigation to obtain the six independent elastic constants, C11, C12, C13, C22, C23 and C33 as shown in [Citation11]. A good agreement has been found between the estimated values of the control structure and the theoretical values that are currently available [Citation38]. Mechanical stability for orthorhombic structures is determined by the following criteria: C22+C33-2C23 >0, C11+C22+C33+C12 +2C13 +2C23 >0 [Citation11]. The findings demonstrate that perovskites satisfy the orthorhombic crystal Born stability criterion where the elastic constants are positive and are mechanically stable. The young’s modulus (E), bulk modulus (B), Poisson’s ratio (τ), and shear modulus (G) are determined using the Voigt-Reuss-Hill (VRH) estimation as shown in based on the computed elastic constants. Through relations 8–10, the temperature of Debye and the velocities of sound in the crystal are closely related to the elastic properties:
Table 3. Calculated elastic constants by adding different concentrations of IL (x%).
Table 4. Calculated parameters of perovskites by adding different amount of IL (x%).
where h – Planck constant and k – Boltzmann constant; NA – Avogadro’s number, n – molecular atomic number, M – molecular mass, and ρ – density, and
longitudinal and transverse, vm – average sound velocities, and
– Debye temperature.
The C11 elastic constant is the greatest of the independent parameters for the halide perovskites. Additionally, the critical value of 1.75 has been widely utilized to gauge a material’s ductility [Citation39]. The results of the calculations showed that the B/G values are significantly bigger than 2.0, indicating that they will deform and yet have bending, tensile, and compression-sensitive properties. For large-scale deformation purposes, they can be employed to fabricate thin-film absorber layers that operate at room temperature. The main cause is ascribed to perovskites’ low shear modulus. A ductile solid’s Poisson’s ratio (τ) is often greater than 0.26 [Citation40]. For all perovskites in this investigation, the Poisson’s ratio was discovered to be greater than 0.3. suggesting that the materials’ band gaps can be adjusted due to their elasticity. The resulting element-dependent perovskite molecules’ weak chemical bonds could be the root of the problem.
Thermodynamic properties
In perovskite solar cells, the synthesis enthalpy and the breakdown reaction energy play a significant influence in the thermodynamic properties of materials under different conditions like heat, and UV light [Citation5,Citation31,Citation41]. The Pb-Sn halide perovskites quickly change into the halides at high temperatures [Citation42,Citation43]. To determine the perovskites’ thermodynamic properties, the formation energies of those materials must be computed.
Expression 12 modified from the general perovskite formation formula is used to estimate the formation energies (H) for the mixed ILxMA1-xSn0.5Pb0.5I3 perovskites [Citation37,Citation44]:
where x represents the quantity of IL additive and ΔHf represents the formation’s enthalpy for ILxMA1-xSn0.5Pb0.5I3. A stable perovskite structure is indicated by endothermic breakdown processes, which occur when ΔH is negative [Citation37].
shows that the addition of up to 1.5% IL tends to lower the formation energies of ILxMA1-xSn0.5Pb0.5I3 perovskites, indicating a structure is thermally stable compared to the control. This could be caused by the presence of IL, which uses hydrogen bonds to anchor MA in the perovskite film and subsequently lower the tendency of MA from escaping and suppressing perovskite deterioration. Additionally, improved grain growth could reduce the number of grain boundaries where the MA can easily escape [Citation29]. When the IL concentration exceeds 3%, the film form begins to deteriorate once more, most likely as a result of shallow impurity bands, which are primarily controlled by structural factors such as particle size, crystallinity, and orientation [Citation45], which increased energy. These results are comparable to both experimental and theoretical Pb-Sn halide perovskites data from the literature [Citation9,Citation44,Citation46,Citation47].
Table 5. Formation energy (ΔH) of perovskites.
Optoelectronic performance
The current density-voltage (J-V) curves shown in were used to evaluate the effectiveness of the device’s photovoltaic capability. The results for the control sample were comparable with other experimental and theoretical studies [Citation39,Citation44]. According to , the IL added perovskite from 0.5% to 15% showed an increased optoelectronic performance metrics compared to the control device. This is sped up by the early light soaking during the current-voltage (J-V) measurements, the morphological trends, and the crystal development, which regulates the grain boundaries, defects or imperfections, and crystal surfaces leading to better charge mobility caused by the stronger electric field [Citation48,Citation49]. Performance characteristics were shown to significantly decline when the IL content increased from 1.5% to 3 mol%. High concentration may exhibit enhanced hysteresis and an asymmetrical eclipse shape in the J-V curve [Citation14,Citation15]. Likewise, more positive ions may result into more electron trapping centres which act as defects in the devices, thereby affecting the optoelectronic properties of the device [Citation16]. Furthermore, there are a possibility of shallow impurity bands that may prevent more light absorption and are primarily regulated by structural parameters including particle size, crystallinity, and orientation which also lowers the optoelectronic properties [Citation45].
Table 6. Optoelectronic performance of perovskites.
The findings of the external quantum efficiency (EQE) spectra, which were integrated over the solar spectrum in , closely match the simulated values of JSC from the J-V curves in . The outcomes show that adding an optimized amount of IL to Sn-based perovskites can improve their optoelectronic performance. A 1.5% IL was used in this study as the ideal quantity to examine the approach for improving the properties and optoelectronic performance of MAPb0.5Sn0.5I3 perovskite since it demonstrated better characteristics and optoelectronic performance. The study’s findings have produced the best outcomes that are equivalent to earlier theoretical and experimental Pb-based research, showing optoelectronic properties such as VOC, JSC and FF ranging between 0.74 v-1.14 v, 21.6mAcm−2-25.55mAcm−2 and 66.2%-84.9%, respectively [Citation50–55].
The improvement in VOC and FF was the most noticeable result after the addition of IL. Considering this, the study simulated the device to learn more about the charge transport and recombination dynamics. To do this, analyses of JSC, VOC, and FF on light-dependent were carried out. VOC is closely related to the quasi-Fermi states of electrons and holes because the positions of the quasi-Fermi levels are governed by the concentrations of free charge carriers [Citation56,Citation57]. The relationship between VOC and light intensity (I) is given by equation 13 [Citation58].
where n – ideality factor, q – elementary charge, k – Boltzmann constant, and T – temperature.
A deviation of the slope from unity indicates that monomolecular Shockley-Read-Hall (SRH) recombination predominates. Previous researches argue that recombination is exclusively caused by traps as the ideality factor approaches a value of 2 [Citation58]. The device in displayed a lower ideality factor value (1.18) than the control device (1.71) implying that the bimolecular recombination predominates in the IL added device whereby trap assisted SRH recombination in the control device predominates.
shows that the device containing IL has a lower degree of non-radiative recombination with integration and a steeper slope of 0.93 contrary to the control with 0.85. These findings agree with previous studies [Citation59,Citation60] and are supported by the FF plot, as demonstrated in . The FF is associated with trap filling and bimolecular recombination which takes place at low photo-generated charge carrier light intensities and at high photo-generated carrier densities, respectively [Citation61].
The value of FF for the IL added device declined first up to around 55 mWcm−2, after which it remained nearly constant at increased light intensities as in . A better FF seen at low light levels is a result of less trap-assisted recombination taking place in the IL-added device. In contrast, in the control device, FF increased as light intensity increased reaching a maximum of about 55 mW/cm−2, then declined with additional increases in light intensity. This indicates that more photo-generated charge carriers are required to fill the traps in the control device, which is consistent with the dominance of trap-assisted recombination. These findings support the VOC vs. light intensity test, which revealed that bimolecular and trap-assisted recombination predominated in the device. However, as compared to the control device, the IL devices were found to have less severe trap-assisted recombination. The findings are also comparable to other theoretical and experimental studies [Citation62,Citation63].
Thermal stability
Both the control and the IL added devices were simulated at various temperatures to investigate the devices’ thermal stability, as illustrated in . The PCEs from the control device reveal a drop to 40% at comparatively low temperatures of 80°C. The control results in this study are in good agreement with the other studies [Citation7]. The PCE of the IL added devices remained above 45% under the same circumstances. The 1.5%IL added device was found to have the highest thermal stability of above 90% at a temperature of 80°C. Both devices drop above 80°C, but the decline of the PCE to IL-added devices is still smaller than the control device. The decrease in device stability at high temperatures in comparison to control devices demonstrates that devices with IL added have the highest thermal stability. This is so that the IL can sequentially lower the amount of leaking MA and stop the breakdown of the perovskite by hydrogen attaching the MA to the perovskite coating. Furthermore, the grain expansion brought on by the addition of IL successfully narrows the grain borders, which allows the MA to escape more easily.
Conclusion
The study concentrated on enhancing the Pb-Sn perovskites’ characteristics. The optoelectronic performance as well as the characteristics of Pb-Sn perovskites were greatly enhanced by adding the ideal amount of BMIBr-IL to the perovskites. This was linked to the development of hydrogen bonds between the MA cations and the ionic liquid as well as the grain growth effect, which lessens the instability of the perovskite films’ grain borders. According to the study’s findings, the most effective technique to boost the performance and long-term stability of Pb-Sn perovskites is to prevent MA molecules from escaping and reduce the weak spots in the perovskite layer.
Disclosure statement
No potential conflict of interest was reported by the author(s).
References
- Wang J, Liu H, Zhao Y, et al. Perovskite-based tandem solar cells gallop ahead. Joule. 2022;6(3):509–12. doi: 10.1016/j.joule.2022.02.011
- Schileo G, Grancini G. Lead or no lead? Availability, toxicity, sustainability and environmental impact of lead-free perovskite solar cells. J Mater Chem C. 2021;9(1):67–76. doi: 10.1039/d0tc04552g
- Min H, Lee DY, Kim J, et al. Perovskite solar cells with atomically coherent interlayers on SnO2 electrodes. Nature. 2021;598(7881):444–450. doi: 10.1038/s41586-021-03964-8
- Matondo JT, Malouangou MD, Wu J, et al. Lead acetate (PbAc2)-derived and chloride-doped MAPbI3 solar cells with high fill factor resulting from optimized charge transport and trap state properties. Sol Energy. 2021;228(August):129–139. doi: 10.1016/j.solener.2021.09.010
- Mbumba MT, Malouangou DM, Tsiba JM, et al. Degradation mechanism and addressing techniques of thermal instability in halide perovskite solar cells. Sol Energy. 2021;230(October):954–978. doi: 10.1016/j.solener.2021.10.070
- Mbumba MT, Malouangou DM, Tsiba JM, et al. Compositional engineering solutions for decreasing trap state density and improving thermal stability in perovskite solar cells. J Mater Chem C. 2021;9(40):14047–14064. doi: 10.1039/d1tc02315b
- Liu X, Yang Z, Chueh C, et al. Improved efficiency and stability of Pb–Sn binary perovskite solar cells by CS substitution. J Mater Chem A Mater Energy Sustain. 2016;4:17939–17945. doi: 10.1039/C6TA07712A
- Ju MG, Sun G, Zhao Y, et al. A computational view of the change in the geometric and electronic properties of perovskites caused by the partial substitution of Pb by Sn. Phys Chem Chem Phys. 2015;17(27):17679–17687. doi: 10.1039/c5cp01991e
- Niu G, Li W, Li J, et al. Enhancement of thermal stability for perovskite solar cells through cesium doping. RSC Adv. 2017;7(28):17473–17479. doi: 10.1039/c6ra28501e
- Korshunova K, Winterfeld L, Beenken WJD, et al. Thermodynamic stability of mixed Pb: Sn methyl-ammonium halide perovskites. Phys Status Solidi Basic Res. 2016;253(10):1907–1915. doi: 10.1002/pssb.201600136
- Feng J. Mechanical properties of hybrid organic-inorganic CH3NH3BX3 (B = Sn, Pb; X = br, I) perovskites for solar cell absorbers. APL Mater. 2014;2(8): doi: 10.1063/1.4885256
- Kahmann S, Chen Z, Hordiichuk O, et al. Compositional variation in FAPb1-xSnxI3 and its impact on the electronic structure: a combined density functional theory and experimental study. ACS Appl Mater Interfaces. 2022;14(30):34253–34261. doi: 10.1021/acsami.2c00889
- Borriello I, Cantele G, Ninno D. Ab initio investigation of hybrid organic-inorganic perovskites based on tin halides. Phys Rev B - Condens Matter Mater Phys. 2008;77(23):1–9. doi: 10.1103/PhysRevB.77.235214
- Shahiduzzaman M, Muslih EY, Hasan AM, et al. The benefits of ionic liquids for the fabrication of efficient and stable perovskite photovoltaics. Chem Eng J. 2021;411(January):128461. doi: 10.1016/j.cej.2021.128461
- Bai S, Da P, Li C, et al. Planar perovskite solar cells with long-term stability using ionic liquid additives. Nature. 2019;571(7764):245–250. doi: 10.1038/s41586-019-1357-2
- Huang C, Lin P, Fu N, et al. Ionic liquid modified SnO2 nanocrystals as a robust electron transporting layer for efficient planar perovskite solar cells. J Mater Chem A. 2018;6(44):22086–22095. doi: 10.1039/c8ta04131h
- Yang D, Zhou X, Yang R, et al. Surface optimization to eliminate hysteresis for record efficiency planar perovskite solar cells. Energy Environ Sci. 2016;9(10):3071–3078. doi: 10.1039/c6ee02139e
- Ghosh S, Singh T. Role of ionic liquids in organic-inorganic metal halide perovskite solar cells efficiency and stability. Nano Energy. 2019;63(June):103828. doi: 10.1016/j.nanoen.2019.06.024
- Diao X, Tang Y, Xiong D, et al. Study on the properties of perovskite materials under light and different temperatures and electric fields based on DFT. RSC Adv. 2020;10(35):20960–20971. doi: 10.1039/d0ra02841j
- Lhouceine M, Omar B, Abdelhafid N, et al. The study of electronic and optical properties of perovskites CH3NH3PbCl3 and CH3NH3PbBr3 using first-principle. E3S Web Conf. 2022;336(January):00015. doi: 10.1051/e3sconf/202233600015
- Soe CMM, Nagabhushana GP, Shivaramaiah R, et al. Structural and thermodynamic limits of layer thickness in 2D halide perovskites. Proc Natl Acad Sci U S A. 2019;116(1):58–66. doi: 10.1073/pnas.1811006115
- Travis W, Glover ENK, Bronstein H, et al. On the application of the tolerance factor to inorganic and hybrid halide perovskites: a revised system. Chem Sci. 2016;7(7):4548–4556. doi: 10.1039/c5sc04845a
- Stoumpos CC, Malliakas CD, Kanatzidis MG. Semiconducting tin and lead iodide perovskites with organic cations: phase transitions, high mobilities, and near-infrared photoluminescent properties. Inorg Chem. 2013;52(15):9019–9038. doi: 10.1021/ic401215x
- Baikie T, Fang Y, Kadro JM, et al. Synthesis and crystal chemistry of the hybrid perovskite (CH3NH3)PbI3 for solid-state sensitised solar cell applications. J Mater Chem A. 2013;1(18):5628–5641. doi: 10.1039/c3ta10518k
- Liu Q, Li A, Chu W, et al. Influence of intrinsic defects on the structure and dynamics of the mixed Pb-Sn perovskite: first-principles DFT and NAMD simulations. J Mater Chem A. 2022;10(1):234–244. doi: 10.1039/d1ta09027e
- Huang J, Yuan Y, Shao Y, et al. Understanding the physical properties of hybrid perovskites for photovoltaic applications. Nat Rev Mater. 2017;2(7). doi: 10.1038/natrevmats.2017.42
- Ahmad M, Rehman G, Ali L, et al. Structural, electronic and optical properties of CsPbX3(X=Cl, Br, I) for energy storage and hybrid solar cell applications. J Alloys Compd. 2017;705:828–839. doi: 10.1016/j.jallcom.2017.02.147
- Kholil MI, Bhuiyan MTH, Rahman MA, et al. Effects of fe doping on the visible light absorption and bandgap tuning of lead-free (CsSnCl3) and lead halide (CsPbCl3) perovskites for optoelectronic applications. AIP Adv. 2021;11(3). doi: 10.1063/5.0042847
- Du J, Wang Y, Zhang Y, et al. Ionic liquid-assisted improvements in the thermal stability of CH3NH3PbI3 perovskite photovoltaics. Phys Status Solidi - Rapid Res Lett. 2018;12(8):1–6. doi: 10.1002/pssr.201800130
- Hao F, Stoumpos CC, Chang RPH, et al. Anomalous band gap behavior in mixed Sn and Pb perovskites enables broadening of absorption spectrum in solar cells. J Am Chem Soc. 2014;136(22):8094–8099. doi: 10.1021/ja5033259
- Mbumba MT, Zhang Y, Yang Y, et al. Role of formamidinium (FA) in the electronic and thermodynamic properties of MA(Pb: Sn)I3 perovskites using first principle calculations. Int J Adv Sci Res Eng. 2023;9(7):1–11. doi: 10.31695/IJASRE.2023.9.7.1
- Ogomi Y, Morita A, Tsukamoto S, et al. CH 3 NH 3 Sn x Pb (1– x) I 3 perovskite solar cells covering up to 1060 nm. J Phys Chem Lett. 2014;5(6):1004–1011. doi: 10.1021/jz5002117
- Mayengbam R, Tripathy SK, Palai G. Structural, electronic, optical and mechanical properties of Zn-doped MAPbI3 perovskites and absorber layer efficiencies: an ab-initio investigation. Mater Today Commun. 2020;24(February):101216. doi: 10.1016/j.mtcomm.2020.101216
- Joshi TK, Pravesh GS, Verma AS. Investigation of structural, electronic, optical and thermoelectric properties of ethylammonium tin iodide (CH3CH2NH3SnI3): an appropriate hybrid material for photovoltaic application. Mater Sci Semicond Process. 2020;115(April):105111. doi: 10.1016/j.mssp.2020.105111
- Joshi TK, Shukla A, Sharma G, et al. Computational determination of structural, electronic, optical, thermoelectric and thermodynamic properties of hybrid perovskite CH3CH2NH3GeI3: an emerging material for photovoltaic cell. Mater Chem Phys. 2020;251(April):123103. doi: 10.1016/j.matchemphys.2020.123103
- Zheng F, Wang LW. Large polaron formation and its effect on electron transport in hybrid perovskites. Energy Environ Sci. 2019;12(4):1219–1230. doi: 10.1039/c8ee03369b
- Zhu S, Ye J, Zhao Y, et al. Structural, electronic, stability, and optical properties of CsPb1-xSnxIBr2 perovskites: a first-principles investigation. J Phys Chem C. 2019;123(33):20476–20487. doi: 10.1021/acs.jpcc.9b04841
- Laamari ME, Cheknane A, Benghia A, et al. Optimized opto-electronic and mechanical properties of orthorhombic methylamunium lead halides (MAPbX3) (X = I, br and cl) for photovoltaic applications. Sol Energy. 2019;182(February):9–15. doi: 10.1016/j.solener.2019.02.035
- Sa R, Liu D, Chen Y, et al. Mixed-cation mixed-metal halide perovskites for photovoltaic applications: a theoretical study. ACS Omega. 2020;5(8):4347–4351. doi: 10.1021/acsomega.9b04484
- Mohd Zaki NH, Ali AMM, Mohamad Taib MF, et al. Dispersion-correction density functional theory (DFT+D) and spin-orbit coupling (SOC) method into the structural, electronic, optical and mechanical properties of CH3NH3PbI3. Comput Condens Matter. 2023;34(July 2022):e00777. doi: 10.1016/j.cocom.2022.e00777
- Mazumdar S, Zhao Y, Zhang X. Stability of perovskite solar cells: degradation mechanisms and remedies. 2021;2(August):1–34.doi: 10.3389/felec.2021.712785
- Ha M, Karmakar A, Bernard GM, et al. Phase evolution in methylammonium tin halide perovskites with variable temperature solid-state 119 Sn NMR spectroscopy. J Phys Chem C. 2020;124(28):15015–15027. doi: 10.1021/acs.jpcc.0c03589
- Savill KJ, Ulatowski AM, Herz LM. Optoelectronic properties of tin–lead halide perovskites. ACS Energy Lett. 2021;6(7):2413–2426. doi: 10.1021/acsenergylett.1c00776
- Liu H, Li X, Zeng Y, et al. Effects of halogen substitutions on the properties of CH3NH3Sn0.5Pb0.5I3 perovskites. Comput Mater Sci. 2020;177(January):109576. doi: 10.1016/j.commatsci.2020.109576
- Ašmontas S, Cerškus A. Impact of cesium concentration on optoelectronic properties of metal halide perovskites. Materials. 2022;15(5):1936. doi: 10.3390/ma15051936
- You Q, Gu S, Gou X. The Highly accurate interatomic potential of CsPbBr3 perovskite with temperature dependence on the structure and thermal properties. Materials. 2023;16(5):2043. doi:10.3390/ma16052043
- Ciccioli A, Latini A. Thermodynamics and the intrinsic stability of lead halide perovskites CH3NH3PbX3. J Phys Chem Lett. 2018;9(13):3756–3765. doi: 10.1021/acs.jpclett.8b00463
- Wang P, Li R, Chen B, et al. Gradient energy alignment engineering for planar perovskite solar cells with efficiency over 23%. Adv Mater. 2020;32(6):1–8. doi: 10.1002/adma.201905766
- Rahman SI, Lamsal BS, Gurung A, et al. Grain boundary defect passivation of triple cation mixed halide perovskite with hydrazine-based aromatic iodide for efficiency improvement. ACS Appl Mater Interfaces. 2020;12(37):41312–41322. doi:10.1021/acsami.0c10448
- González-Juárez E, Espinosa-Roa A, Cadillo-Martínez AT, et al. Enhancing the stability and efficiency of MAPbI3 perovskite solar cells by theophylline-BF4− alkaloid derivatives, a theoretical-experimental approach. RSC Adv. 2023;13(8):5070–5080. doi: 10.1039/d2ra07580f
- Chandel A, Bin Ke Q, Chiang SE, et al. Improving device performance of MAPbI3 photovoltaic cells by manipulating the crystal orientation of tetragonal perovskites. Nanotechnology. 2022;33(41): doi: 10.1088/1361-6528/ac7474
- Raoui Y, Ez-Zahraouy H, Tahiri N, et al. Performance analysis of MAPbI3 based perovskite solar cells employing diverse charge selective contacts: simulation study. Sol Energy. 2019;193(February):948–955. doi: 10.1016/j.solener.2019.10.009
- Ashrafi SS, Hossain MK, Islam MM, et al. Characterization and fabrication of Pb-based perovskites solar cells under atmospheric condition and stability enhancement. Adv Mater Phys Chem. 2020;10(11):282–296. doi: 10.4236/ampc.2020.1011022
- Prasanna JL, Goel E, Kumar A. Numerical investigation of MAPbI3 perovskite solar cells for performance limiting parameters. Opt Quantum Electron. 2023;55(7):1–21. doi: 10.1007/s11082-023-04876-9
- Wang Y, Liang Y, Zhang Y, et al. Pushing the envelope: achieving an open-circuit voltage of 1.18 V for unalloyed MAPbI3 perovskite solar cells of a planar architecture. Adv Funct Mater. 2018;28(30):1801237. doi: 10.1002/adfm.201801237
- Rajagopal A, Liang PW, Chueh CC, et al. Defect passivation via a graded fullerene heterojunction in low-bandgap Pb-Sn binary perovskite photovoltaics. ACS Energy Lett. 2017;2(11):2531–2539. doi: 10.1021/acsenergylett.7b00847
- Kapil G, Ripolles TS, Hamada K, et al. Highly efficient 17.6% tin-lead mixed perovskite solar cells realized through spike structure. Nano Lett. 2018;18(6):3600–3607. doi: 10.1021/acs.nanolett.8b00701
- Ghimire N, Bobba RS, Gurung A, et al. Mitigating open-circuit voltage loss in Pb-Sn low-bandgap perovskite solar cells via additive engineering. ACS Appl Energy Mater. 2021;4(2):1731–1742. doi: 10.1021/acsaem.0c02895
- Hu H, Moghadamzadeh S, Azmi R, et al. Sn-Pb mixed perovskites with fullerene-derivative interlayers for efficient four-terminal all-perovskite tandem solar cells. Adv Funct Mater. 2022;32(12). doi: 10.1002/adfm.202107650
- Yao D, Mao X, Wang X, et al. Dimensionality-controlled surface passivation for enhancing performance and stability of perovskite solar cells via triethylenetetramine vapor. ACS Appl Mater Interfaces. 2020;12(5):6651–6661. doi:10.1021/acsami.9b19908
- Lian X, Chen J, Zhang Y, et al. Highly efficient Sn/Pb binary perovskite solar cell via precursor engineering: a two-step fabrication process. Adv Funct Mater. 2019;29(5):1–9. doi: 10.1002/adfm.201807024
- Wang Y, Yang Y, Li N, et al. Ionic liquid stabilized perovskite solar modules with Power conversion efficiency exceeding 20%. Adv Funct Mater. 2022;32(38): doi: 10.1002/adfm.202204396
- Jahandar M, Khan N, Jahankhan M, et al. High-performance CH3NH3PbI3 inverted planar perovskite solar cells via ammonium halide additives. J Ind Eng Chem. 2019;80(September):265–272. doi: 10.1016/j.jiec.2019.08.004