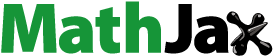
ABSTRACT
The quest for biocompatible and antibacterial wound skin care dressings based on biopolymers has become a burgeoning focus in biomedicine. A wound care dressing of GG+SA@CUR thin film was prepared, and its properties were evaluated. FTIR analysis revealed distinct functional groups for GG, SA, and CUR. The GG+SA@CUR thin film is in an amorphous state, showing that CUR was successfully encapsulated for controlled drug release. The SEM images show the presence of CUR particles, which cause the film to have significant antibacterial properties. The inhibition zone was found to be 16.67 ± 0.26 mm and 12.06 ± 0.08 mm against S. aureus and E. coli. The good biocompatibility characteristics of the GG+SA@CUR thin film were proven by the high number of fibroblast cells. The scratch cells migrated faster after being treated with GG+SA@CUR film and accelerated wound healing in in vitro conditions, indicating their potential as new transdermal skin wound care.
Introduction
Wound healing is a complex process involving haemostasis, inflammation, cell proliferation, and remodelling [Citation1]. During the inflammatory phase, vascular contraction increases vascular permeability, allowing neutrophils, macrophages, and lymphocytes to invade. Cell proliferation is the next stage, and it is generally acknowledged that fibroblasts are necessary for both cell proliferation and the development of new blood vessels since they release collagen [Citation2]. During the remodelling phase, collagen near the injury site is reorganised, and angiogenesis activity is stopped. Additionally, collagenase then mediates the recycling of collagen, with too much collagen at the injury site eventually leading to the creation of hard scars [Citation3].
Reactive oxygen species (ROS) are essential for the removal of germs and necrotic tissue during the inflammation phase; somehow, the excessive ROS levels brought on by a protracted inflammatory response might hinder the regeneration of tissues for the wound healing process [Citation3]. Excessive ROS can not only cause impairment to the cell membrane, proteins, and DNA but also damage the wound edge and the remaining repair cells in the wound [Citation3]. For instance, the overproduction of ROS in diabetic chronic wounds can hinder angiogenesis and cause ongoing inflammation due to oxidative stress [Citation4]. To prevent the damage caused by oxidative stress, the ROS content in the wound microenvironment must be under control. A promising strategy to combat the excess ROS and enhance tissue healing is to incorporate antioxidants to curb ROS release at the wound site [Citation5,Citation6].
One of the greatest natural antioxidant polyphenols, curcumin (CUR) or CUR derivatives, has been extensively used to inhibit ROS metabolic enzymes by competing with co-enzymes in the treatment of wounds. By accelerating the deposition of collagen, increasing vascular density, and restoring the structural epidermis at the wound site, CUR has been shown to accelerate wound healing [Citation7–9]. The CUR compound is also renowned for its antimicrobial properties, which operate through diverse mechanisms against varied bacterial species. Balan et al. demonstrated a noteworthy inhibition of S. aureus growth in vitro through a combination of CUR and whey proteins [Citation10]. Correspondingly, Sharma et al. [Citation11] have previously reported a similar combination effect of CUR and various phytochemicals, such as cinnamaldehyde, ellagic acid, and eugenol, against S. epidermidis, a closely related strain of S. aureus [Citation12]. It has been reported that the primary mechanism responsible for the bactericidal effect induced by CUR is the distortion of the 1,2-dipalmitoyl-sn-glycero-3-phosphocholine (DPPC) membranes found in both S. aureus and E. coli. This has been evidenced by several studies [Citation13,Citation14]. Research has also revealed that CUR’s antibacterial efficacy against Bacillus subtilis is due to its ability to obstruct the assembly dynamics of FtsZ in the Z ring, thus hampering bacterial cell proliferation [Citation15,Citation16]. With respect to P. aeruginosa infection, CUR has been demonstrated to have anti-infective activity by impeding virulence, quorum sensing, and biofilm initiation [Citation17]. While CUR’s lipophilic nature enables it to intercalate into the lipopolysaccharide-containing cell membrane, enhancing the permeability of gramme-negative bacteria [Citation18].
However, there are some drawbacks to using CUR, including its quick metabolization and ability to cause mitochondrial dysfunction at large doses. Moreover, the utilisation of CUR in food supplements and nutraceutical products is a challenging endeavour due to its notable chemical instability [Citation19]. It has been observed that CUR exhibits a propensity for crystallization under aqueous acidic conditions, which greatly contributes to its instability and susceptibility to chemical degradation in basic aqueous conditions. This degradation has been attributed to alterations in the molecular structure of CUR [Citation20]. Additionally, the CUR’s restricted therapeutic application was brought on by its poor water solubility and short lifespan [Citation21]. Shehzad and colleagues conducted a study on the excretion of native CUR following oral administration, revealing that 40% of excretion occurred in the faeces. Furthermore, CUR was observed to undergo degradation in an aqueous solution and at a neutral pH, which may contribute to its short half-life. The lifespan of CUR in rats was reported to be 28.1 ± 5.6 and 44 ± 7.5 h for intravenous and oral doses of 10 mg/kg and 500 mg/kg, respectively [Citation22].
Controlled release platforms utilising nanotechnology and stimuli-responsive systems have been developed to enhance the therapeutic effects and avoid the cytotoxicity of CUR. For instance, a matrix metalloproteinase 9 (MMP-9) responsive system based on CUR self-assembled particles contained in gelatine microspheres and thermos-responsive poloxamer hydrogel has been studied [Citation9]. The controlled release of CUR at the wound site was the goal of incorporating CUR into the biopolymer. Somehow, poor bioavailability of CUR is the major challenge for human application. Upon the administration of substantial doses of CUR, the resultant plasma concentration becomes negligible within hours [Citation23]. The bio-distribution, cellular uptake, and excretion of CUR in Sprague-Dawley rats were examined by Wahlstrom and Blennow, who found that a high oral dose of 1 g/kg resulted in 75% excretion in faeces, with only negligible amounts of native CUR detected in the blood plasma levels of rats. These findings suggest that native CUR is poorly absorbed from the gut [Citation24]. The utilisation of the advanced CUR delivery system has the potential to enhance efficiency, solubility, and bioavailability while simultaneously elevating the blood half-life and reducing the deterioration rate [Citation25]. Various formulations have been utilised for efficient delivery of CUR; among them are polymeric nanoparticles, liposomes, hydrogels, nano-emulsions, nanofibers, lipid transferors, and polymer mixture membranes [Citation26,Citation27]. The muco-adhesiveness of the alginate material makes it easier for drug molecules to be intercalated, which raises the bioavailability of CUR. As a result, sodium alginate (SA) was utilized in the current work to increase the drug encapsulation efficiency of CUR for advanced delivery. SA is an anionic polysaccharide with biocompatibility, hydrophilicity, biodegradability, and potential in drug administration applications [Citation28,Citation29]. It is compatible with other polymers like gellan gum (GG) to regulate release rate and swelling qualities. The GG has been investigated as a stomach-specific delivery system and has shown promise in tissue engineering and wound healing applications [Citation30,Citation31].
This study aimed to fabricate mixed polymers of gellan gum and sodium alginate encapsulating curcumin (GG+SA@CUR) thin films using the solvent casting method as a potential skin wound care dressing. The physicochemical properties of the films were assessed, including antibacterial activity, antioxidant capacity, and release rate. Biocompatibility with fibroblast cells for cell viability and proliferation was also investigated, and in vitro wound healing via scratch fibroblast cells was considered for potential wound care dressings.
Materials and methods
Materials
Low-acyl gellan gum (Gelzan™ CM, Mw ≈2–3 × 105 Da), sodium alginate (SA, viscosity 5.0–40.0 cps), and curcumin powder (CUR, Mw: 368.38 g/mol) were obtained from Sigma-Aldrich, Malaysia. Glycerol, calcium chloride and ethanol were bought from Ajax Finechem Pty Ltd., Australia. For cell culture, Dulbecco’s Modified Eagle’s Medium (DMEM) high glucose, foetal bovine serum (FBS), 0.05% trypsin/EDTA, 3-(4,5-dimethylthiazol-2-yl-2,5-diphenyltetrazolium bromide) (MTT), and phosphate buffer saline (PBS) were acquired from Sigma-Aldrich. The standard analytical grade applied to every other chemical solvent. All materials were used as initially received.
Film formation
The solvent-casting method, which is reported in the literature, was used to create polymer-based films [Citation32,Citation33]. In 100 mL of distilled water that already has 0.5 g of glycerol as a plasticizer, 1 g of GG and 1 g of SA were added. After 1 h of stirring at 1000 rpm to prevent local gelation, the mixture’s temperature was raised to 70°C while the solution was still being stirred. The mixture was then crosslinked by the addition of a 5 mL CaCl2 solution (0.5% w/w) dropwise at a rate of 1 mL/min. Under the same circumstances, stirring was carried out for an additional 30 min after introducing the CaCl2 solution. After that, the mixture was cooled to room temperature. Using a modified antisolvent precipitation method [Citation34], an aqueous CUR solution was prepared by dissolving 5 mg/mL of CUR powder in ethanol. This solution was introduced into deionized water at a fixed flow rate of 1 mL/min while maintaining constant stirring through a dropping funnel. The ratio of ethanol to water was maintained at 1:10 (v/v), which translates to 20 mL of curcumin solution in ethanol for every 200 mL of deionized water. Then, the aqueous solution of CUR was added to the combination of the initial ingredients. The stirring remained at room temperature for another 30 min. The mixture was then homogenised using sonication to make sure that CUR was homogenously distributed in the polymer matrix. The mixture was then put into petri dishes with a diameter of 9 cm and dried for 24 h at 40°C. After drying, films were produced, but they were only partly crosslinked. The dried, partly crosslinked films were dipped into a 10% glycerol and 3% calcium chloride solution to complete the crosslinking process. After crosslinking, extra calcium chloride and glycerol were washed off the film surface using distilled water. Finally, the prepared films were allowed to dry in the air for at least 24 h at room temperature. A similar procedure was repeated for the preparation of GG+SA film, except the CUR was not added to the polymer matrix. Prior to testing, all films were pre-conditioned in a desiccator at 24°C and 50% relative humidity (RH).
Characterization of the films
The characterization of prepared films as well as starting materials were done using Attenuated Total Reflectance-Fourier Transform Infrared Spectroscopy (ATR-FTIR), Scanning Electron Microscopy (SEM) and X-Ray Diffraction (XRD) for physiochemical properties studies according to the previous researchers [Citation35,Citation36]. Starting materials of GG, SA, CUR, and each of the fabricated thin films underwent ATR-FTIR for the systematic analysis of selected functional groups and bonding between the blended polymers using a Perkin Elmer Spectrum 100 FTIR spectrophotometer in the transmittance mode. A single-bounce beam path, 45° incidence angle, 16 scans, 4 cm−1 resolution was used to capture ATR-FTIR spectra at a range of 4000–400 cm− 1. Using SEM, the morphology of the starting materials as well as thin films containing and without curcumin were assessed using JOEL JSM 6360 LA. Each sample was placed to a stub using double-sided carbon adhesive tape after drying for 8 h at 60°C. The dried samples were sputter-coated with a thin coating of gold particles under decreased pressure, and pictures were taken at 5 kV accelerating voltage. XRD analysis was performed at room temperature using a Rigaku Miniflex (II) X-ray diffractometer from 5° to 40° of 2θ.
Antioxidant efficiency
The films antioxidant efficiency was assessed by scavenging the 1,1-diphenyl-2-picrylhydrazyl (DPPH) free radical as previously reported [Citation36]. A 200 µM concentration of DPPH was dissolved in anhydrous ethanol. After that, 500 mg of GG+SA@CUR thin film was put in 3 ml of DPPH solution and incubated for 1 h at 37°C. The reaction solution’s 517 nm absorbance was then measured using a UV-vis spectrophotometer. The effectiveness of scavenging free radicals was determined by EquationEquation (1)(1)
(1) :
where A0 is the absorption of the blank (DPPH solution), and At is the absorption of the sample (DPPH solution + thin film). The entire experiment was done in the dark and the same procedure was repeated for pure CUR powder to study their antioxidant efficiency. The experiment was performed in triplicate, and the average value was reported.
Anti-bacterial studies
Gram-positive of Staphylococcus aureus (MTCC1303) and gram-negative bacteria Escherichia coli (ATCC25922) were used as test organisms to determine the antibacterial efficacy of the film using the disc diffusion technique [Citation37]. Staphylococcus aureus and Escherichia coli were selected because the wound site is typically dominated by these bacteria. Nutrient agar plates were covered with 200 µL of the overnight developed bacterial culture (1 × 106 cells/mL). After that the sterile thin film samples (8 mm diameter) were positioned on the agar surface, which was then incubated at 37°C for 24 h. Zone of inhibition was measured using a digital vernier micrometre following a 24-h incubation period. For every sample, the test was run in triplicates.
CUR loading capability and encapsulation efficiency
The determination of encapsulation efficiency (%) and loading capacity (%) of CUR were adapted from the reported method [Citation38] with modifications. The prepared GG+SA@CUR thin film (0.1 g) was accurately weighed and dissolved in 50 mL of 95% (v/v) ethanol solution. The solutions were continuously stirred for 2 h and sonicated using the ultrasonic processor UP50H (Hielscher, Teltow, Germany, 50 W, 30 kHz) for complete dissolution. The samples were centrifuged, and the free CUR content in the supernatant was determined spectrophotometrically at 420 nm. The concentration of CUR in the solution was determined using a spectrophotometric method. Before that, the stock solution of CUR was serially diluted to obtain six different working concentrations, including 5, 10, 15, 20, 25, and 30 μg/mL of CUR, to establish a calibration curve. The absorbance was measured at 420 nm using an ultraviolet (UV) – visible spectrophotometer (UV-2000, UNICO, Shanghai, China). The encapsulation efficiency and loading capacity of CUR can be calculated using the EquationEquations (2)(2)
(2) and (Equation3
(3)
(3) ), respectively:
Drug release
The drug release of GG+SA@CUR was evaluated by dialysis method [Citation39]. The GG+SA@CUR thin film (2 × 2 cm2) was immersed in 10 ml of phosphate buffer saline (PBS) with a pH of 7.4 and 0.1% Tween 80 used as the dissolving media. Then, the solution was shaken using a horizontal shaker at 37.5°C for 24 h. After that, within 2 days, 1 ml samples were taken at specified intervals of 0, 6, 12, 18, 24, 30, 36, 42 and 48 h. Withdrawn solution at each interval was substituted with equivalent volume of intact buffer solution. The drug concentration was quantified using UV-visible spectrophotometer at 420 nm by comparing it with the constructed standard calibration curve. The amount of released drug can be calculated using EquationEquation (4)(4)
(4) :
where drug released indicates the amount of released CUR from thin film and CUR loading denotes the total amount of curcumin encapsulated in the thin film.
EquationEquation (5)(5)
(5) was used to fit Peppas’ model to the experimental data to study the kinetics of CUR release [Citation40];
The parameters K and n represent the kinetic constant and the diffusion exponent suggesting the nature of the release mechanism, respectively, while Mt and M∞ respond to the amount of drug released at time t and the total amount of drug released, respectively.
Biodegradation studies
The sample biodegradation was performed at a temperature of 37°C within phosphate buffer solutions (PBS) of pH 7.4 comprising lysozyme (0.2% solution). The samples were cut into square pieces (2 × 2 cm2) and incubated in the aforementioned solution with intermittent shaking. The samples were retrieved at different time intervals, rinsed with deionised water, and permitted to air-dry until a consistent weight was achieved. The degradation rate (S) was evaluated by the proportion of the weight loss to the original weight of samples, as illustrated in EquationEquation (6)(6)
(6) :
where Wt and W0 represent the weight of samples following complete drying and the initial weight, respectively.
Biocompatibility studies
The biocompatibility study of the films towards cells was determined using a standard MTT test [Citation41]. The viability of 3T3 fibroblast cells treated with prepared films and an untreated tissue culture polystyrene plate (TCPP) as a control sample was compared. Firstly, the GG+SA and GG+SA@CUR films were cut into cylinders of 8 mm in diameter and then underwent a 2-h UV sterilisation process on both sides. On the other hand, the cell was seeded onto 96-well culture plates (5,000 cells/well) and cultured at 37°C for 24 h to promote cell adhesion to the plate. Then, the films were put into 96-well plates, and a well without a film was used as the positive control. The plates were incubated for 24, 48, and 72 h at 37 degrees Celsius with 5% CO2 and 100% relative humidity in the air. The viability of cells in contact with the thin film samples at different times of incubation was examined through a staining procedure of acridine orange/propidium iodide (AO/PI, Sigma Aldrich, U.S.A.) and observed by a light microscope (Olympus IX73-FL-CCD). The negative control in this experiment is culture media without the presence of film samples. After that, 100 µL of MTT solution (0.5 g/mL) was added, and the cell culture was left to incubate for 4 h. The excess MTT stain was removed from the treated cell culture by using a DMSO solution. After incubation, the absorbance was measured using a microplate reader (Multiskan Ascent 96/384, U.S.A.). The absorbance readings were converted to cell numbers using calibration curves of 3T3 mouse fibroblast cells in 96-well plates under the same condition. Each experiment was carried out in triplicate for cell proliferation measurement.
In vitro wound healing studies
The in vitro wound healing studies using a scratch wound assay were carried out based on the previous study [Citation42]. A scratch wound assay was used to assess the fibroblasts’ ability to migrate and close wounds, which is one of the critical stages of the wound healing process [Citation43,Citation44]. In a 24-well plate, 3T3 fibroblast cells were grown over 24 h to form a cell monolayer that was 80%–90% confluent. Additionally, a serum-free medium containing mitomycin C (5 g/mL) was given to the cells for 12 h before being discarded and washed to inhibit cell multiplication. After that, a micropipette tip was used to scratch the monolayers, creating linear wounds with a cell-free zone. The wells were scratched and then washed three times in PBS to get rid of any floating or decomposing cells. Each well was then filled with the medium containing the sterile films, which were then incubated at 37°C with 5% CO2. The untreated monolayer of scratched cells in the whole medium was regarded as the control. An inverted microscope was used to track and take pictures of the wound closure rate at 0, 8, and 24 h following scratching. Finally, using Image J software, the open wound area was estimated at the appropriate periods.
Statistical analysis
All data were subjected to statistical analysis and were reported as a mean ± standard deviations for triplicate experiments using Statistical Package for Social Science (SPSS). A one-way ANOVA was used to determine the differences between samples. The p-value <0.05 was considered statistically significant.
Results and discussion
Film characterization
The FTIR spectra of gellan gum (GG), sodium alginate (SA), and curcumin (CUR) starting materials were depicted in . Wide absorption bands in the range of 3620–2960 cm−1 and 2960–2785 cm−1 wavelengths may be seen in the spectra of GG, SA, and CUR, respectively. These absorption bands are caused by the valence vibration of the O-H bond and the C-H bond, respectively [Citation45]. For the GG sample, the absorption band at 1739 cm−1 was observed to be characteristic of the GG since it is the vibration of the carbonyl group in the GG [Citation46]. The strong absorption peak that can be observed at ~1592 cm−1 for the GG sample was assigned to the C=C stretching [Citation47]. While the peaks at 1404 cm-1 and 1367 cm-1 were characteristics of the scissoring and asymmetric bending modes of CH2, respectively. The strong absorption band that appeared at 1022 cm1 was attributed to the C-O-C stretching vibration [Citation48]. For the alginate sample, the observed bands at 1592 cm−1 and 1404 cm−1 were attributed to asymmetric and symmetric stretching vibrations of the COO-groups, respectively, and are specific to the ionic binding [Citation49,Citation50]. The peak at 1022 cm−1 attributed to the C-O-C stretching shows a higher intensity, suggesting a stronger binding of the guluronic acids from sodium alginate. The IR spectrum of CUR demonstrated stretching vibration at 1628 cm−1, attributed predominantly to the stretching vibrations of the C=C character. The band at 1511 cm−1 is attributed to the vibrations of stretching the carbonyl bond (C=O). Furthermore, a significant intense band at 1000 cm−1 is attributed to the bending vibration of the C-O-C, which is characteristic of the CUR [Citation51].
also shows the FTIR spectra of GG+SA and GG+SA@CUR thin films. A broad absorption band at 3340 cm−1 may be seen in the spectra of both films due to the valence vibrations of the OH bonds found in polysaccharides. Additionally, it is possible to see the valence vibrations of C-H bonds that take place between 2916 cm−1 and 2856 cm−1. The vibrations of the groups that are distinctive to both GG and SA are all present in the IR spectra from 1400 to 400 cm−1. However, because of the polysaccharides’ interaction, the peaks that correspond to the distinctive vibrations of GG and SA have somewhat changed. The presence of sodium ions facilitates the electrostatic interaction between GG and SA biopolymers. Furthermore, there are substantial hydrogen bonds between biopolymers in addition to this electrostatic interaction, which causes changes in peaks referring to the GG and SA functional groups. While a strong band at 1590 cm−1 that appeared in the GG+SA@CUR sample is due to the vibration of the carbonyl group belonging to CUR. The GG and SA can be linked through ionic crosslinking and hydrogen bonding [Citation52,Citation53]. The GG contains a hydroxyl (−OH) group that can form hydrogen bond with -OH of alginate to form GG+alginate. The bonding and physiochemical properties of GG+alginate can be improved by the addition of cross-linker agent in the structure, such as calcium chloride (CaCl2) [Citation54–57].
The XRD diffractograms for the starting materials and the fabricated films are displayed in , respectively. In , the primary ingredients of GG and SA exhibit a low degree of crystallinity since broad peaks were observed in their XRD pattern. However, CUR has higher crystallinity because sharp peaks were observed at 2 thetas of 15.04°, 17.15°, 18.10°, and 26.60°, besides a broad peak at 23.38°. XRD peaks of curcumin revealed strong reflections in the range of 10–30°, which pointed to the crystalline nature of the used CUR [Citation58]. For the thin film sample, both GG+SA and GG+SA@CUR show an amorphous character because only broad peaks were obtained. A single peak at 27.76° was observed for GG+SA thin film, while the peaks appeared at 11.20°, 16.86°, 19.40°, 22.16°, and 27.76° for GG+SA@CUR thin film. Similar peaks at ~27° in both films suggest that the biopolymer matrix based on GG and SA was successfully fabricated. The additional peaks for the GG+SA@CUR thin film, which appeared at 11.20° to 22.16°, confirm the presence of curcumin [Citation59]. In the film form, the crystallinity of the CUR sample was reduced as compared to pure material, suggesting that the CUR was successfully encapsulated into the GG+SA biopolymer matrix.
The morphology of the GG+SA and GG+SA@CUR films were investigated using SEM. For comparison, the SEM images of GG, SA and CUR starting materials were also captured. The GG was seen to have a non-homogenous and rough surface (). The rough surface morphology may aid in the formation of a very viscous aqueous solution. This finding is in line with the previous study stated that the particle size and specific surface area have a substantial impact on the inherent viscosity and molecular mass of gums [Citation60]. For the pure SA, fragment-like loose surface is observed in , which is indicative of semi-crystalline and amorphous structure [Citation61]. In the case of CUR powder, non-uniform particle shape was observed due to the agglomeration of particle because of its hydrophobicity nature ().
displays the photographic images of the prepared GG+SA and GG+SA@CUR thin films, while their corresponding SEM images are presented in . As shown in , the GG+SA film sample had a rough surface because of the formation of the three-dimensional polymer network between GG and SA. Similar morphology was observed for GG+SA@CUR, except the presence of a small particle on the surface attributed to the CUR (). The CUR is successfully loaded on the thin film surface and homogeneously distributed, as can be observed in the SEM picture with higher magnification corresponding to the GG+SA@CUR thin film sample. In , the cross-section SEM micrograph of the GG+SA@CUR thin film showed some irregularities typical of semi-crystalline structures, namely the co-existence of crystalline and amorphous phases. Moreover, the irregularities were not limited to the layer near the film surface but spread throughout the whole cross section and may have been due to the encapsulation of CUR. Furthermore, the element information and distribution of CUR in GG+SA@CUR were analysed using energy-dispersive x-ray (EDX) mapping, as shown in . The result confirmed that CUR was presented in samples. The typical elements on the surface of the GG+SA@CUR films are C, O, and Na. The presence of Au is due to the gold coating for SEM analysis.
Antioxidant studies
The DPPH reduction technique was used to calculate the films’ free-radical scavenging values. The starting material for CUR powder had 72.29% antioxidant activity. The phenolic hydroxyl group is mostly to blame for the CUR’s antioxidant action. By securing reactive oxygen species, biologically active compounds found in CUR, particularly phenolic compounds, were able to prevent oxidative stress responses and, as a result, confer strong antioxidant properties [Citation62,Citation63]. After encapsulation, the CUR maintained its capacity to scavenge free radicals; in fact, the GG+SA@CUR shows a higher antioxidant activity of 85.79%. The findings demonstrate the better DPPH• free radical scavenging activity of GG+SA@CUR thin film, suggesting that the microenvironment for CUR’s reactivity with DPPH+ radicals is improved by encapsulating CUR in biopolymer. CUR thus more readily offers an H to convert DPPH• to non-radical forms [Citation64]. Moreover, the CUR is more water-soluble in GG+SA@CUR by the interaction of hydrophobic molecules, boosting its antioxidant ability [Citation65]. CUR is a natural antioxidant compound found in turmeric with well-documented antioxidant properties [Citation66]. Thus, the GG+SA@CUR can provide additional antioxidant activity as compared to pure GG+SA film. Moreover, the presence of CUR can scavenge free radicals and reactive oxygen species, reducing oxidative stress and promoting wound healing [Citation67]. This enhanced antioxidant activity can help protect cells and tissues from damage, further supporting the healing process. Interestingly, the antioxidant activity of GG+SA@CUR thin film obtained in this study is higher and comparable with other reported film as depicted in .
Table 1. Anti-oxidant activity of GG+SA@CUR thin film and different samples of the curcumin-loaded film.
Anti-bacterial studies
As illustrated in and stated in , the GG+SA@CUR film displayed inhibition zones measuring 16.67 ± 0.26 mm and 12.06 ± 0.22 mm against Staphylococcus aureus (S. aureus) and Escherichia coli (E. coli). In contrast, no inhibition was observed for the GG+SA thin film. This finding suggests that the GG+SA@CUR thin film exhibits superior antibacterial properties compared to the pure GG+SA thin film and the control sample treated with penicillin. The enhanced antibacterial effect can be attributed to the presence of CUR in the GG+SA@CUR thin film. Moreover, previous studies have also shown that materials containing CUR provide good antibacterial activity (). CUR has the capability to reduce bacterial virulence factors, impede bacterial biofilm formation, and restrict bacterial adherence to host receptors through the bacterial quorum sensing (QS) control system [Citation77]. For instance, S. aureus is known to produce virulence factors such as phenol-soluble modulin α (PSMα) and PSMδ [Citation78]. CUR can thwart these virulence behaviours by affecting the QS system, as the signalling molecules in the QS system regulate virulence factors [Citation77]. Unlike many antibiotics, which often employ a single mechanism [Citation79], CUR operates through a multi-mechanism anti-infection strategy, interacting with numerous molecular targets and transduction pathways [Citation80]. Additionally, CUR inhibits the self-assembly of S. aureus haemolysin, which in turn prevents haemolysis. It achieves this by attaching to the stem region of the protein through Van der Waals interactions and hydrogen bonding, disrupting the conformational changes required for self-assembly and diminishing the biological activity of haemolysin [Citation81]. Furthermore, research conducted by Tyagi et al. demonstrates that CUR induces membrane leakage when applied to the cell membrane of S. aureus, further hindering bacterial growth [Citation82]. In the case of E. coli, CUR suppresses FtsZ (a prokaryotic homologue of eukaryotic cytoskeletal protein tubulin) assembly, which leads to the inhibition of bacterial cell division [Citation16]. These multifaceted mechanisms contribute to the impressive antibacterial properties of the GG+SA@CUR thin film.
Figure 7. Disk diffusion results of penicillin (positive control), GG+SA and GG+SA@CUR thin films against the Staphylococcus aureus (S. aureus) and Escherichia coli (E. coli) after incubating for 24 h.

Table 2. Anti-bacterial activity of penicillin, GG+SA, GG+SA@CUR thin films and different types of the samples containing CUR as indicated by the zone of inhibition (mm).
Encapsulation efficiencies and CUR drug loading studies
The encapsulation efficiencies and drug loading of GG+SA@CUR thin film was found to be 90.61 ± 0.84% and 49.75 ± 0.75%, respectively. The encapsulation rate of CUR in this research is similar to values reported for CUR loaded carriers in recent studies, such as whey protein-based nanoemulsions (90.56%) [Citation83], Lallemantia iberica seed gum (LISG)/Ca2+ nanoparticles (88.23%) [Citation84], and chitosan/poly (ε-caprolactone) nanoparticles (86,70.9%) [Citation85]. Additionally, the drug loading percentage achieved in this study is the highest as compared to previous studies (). This is attributed to the nature of the gelatinized GG+SA films, which have the capacity to encapsulate a greater number of drug molecules, resulting in a higher drug loading percentage.
Table 3. Comparison of CUR drug loading percentage for different delivery systems.
Drug release
The drug release from encapsulated materials often extends the duration of action. By delaying its release into the bloodstream and restricting its effects to the target cell, encapsulation of drugs enhances their bioavailability, sustained delivery, and targeting to a specific spot. From this perspective, the release of CUR in GG+SA@CUR and pure CUR powder has been measured. A free CUR was released quickly in vitro within 24 h (>90%), but incorporation of CUR into the GG+SA delayed this release to 56% (), indicating that CUR was released gradually from the GG+SA@CUR, lengthening the duration of action. The CUR release could be extended up to 48 h, and this action may be attributed to the GG+SA involvement. There is a possibility to release all the loaded drugs from the thin film by elongating the release time. In this study, the drug release experiment extended up to 48 h since more than 80% of the drug had been released in the case of the pure CUR sample. It’s worth noting that previous studies have also reported that approximately 80% of CUR was released within 48 h [Citation92].
The ability to slow the release of CUR species could be evoking antibacterial activity continuously and sustainably on a bacterial strain, particularly against S. aureus and E. coli, two opportunistic microbes frequently linked to human and animal infections or diseases. On top of that, the sustained CUR released from the GG+SA@CUR thin film will prolong the existence of drugs at the wound sites, thus accelerating wound healing. The polymer-drug electrostatic interactions were able to keep the drug within the structure, promoting a sustained release of CUR in simulated saliva media. Based on the previous study, the Peppa’s model is utilized to explicate the release mechanisms [Citation93], wherein the exponent ‘n’ plays a significant role. A value of ‘n’ = 0.5 denotes the typical Fickian diffusion, whilst ‘n’ = 1 represents case II diffusion. An ‘n’ value ranging between 0.5 and 1 indicates a non-Fickian mechanism. The predicted ‘n’ exponent for the sample is 0.384, which is less than 0.5, which infers that the drug release from GG+SA@CUR thin film follows non-Fickian diffusion [Citation94]. The correlation coefficients for the model applied to the CUR containing samples were 0.998, signifying a suitable fit of the model to the experimental data.
Biodegradation studies
A biodegradation investigation was conducted in the presence of the lysozyme enzyme, and both films underwent gradual degradation for a duration of 7 days. The pure GG+SA thin film underwent full degradation in 4 days, while GG+SA@CUR required 7 days for complete degradation. During the initial 3 days, both films experienced rapid degradation at an equivalent enzymatic degradation rate. Subsequently, the degradation transpired at a reduced pace for the following days. Conversely, upon incorporating CUR into GG+SA, the rate and degree of degradation were slightly reduced, indicating an enhanced resistance to enzymatic degradation [Citation95,Citation96]. Nevertheless, after 7 days of incubation, both pure GG+SA and GG+SA@CUR thin films were fully degradable and did not leave any wound dressing residual. This finding is interesting because it can reduce the waste associated with traditional wound dressings, which are not fully biodegradable.
Biocompatibility studies
The MTT test was used to evaluate the biocompatibility of films on the viability of 3T3 fibroblast cells. For comparison, untreated 3T3 fibroblast cells on a tissue culture polystyrene plate (TCPP) were used as a control sample. The cell was growing extremely healthy, and interestingly, the cell viability was much higher in the presence of curcumin-containing films after 24, 48, and 72-h incubation periods as compared to the GG+SA film and control sample (). This is due to the active compound in the CUR of the GG+SA@CUR thin film sample. It is well known that CUR is a therapeutic drug, and as a result, it can increase cell proliferation [Citation97]. CUR has been demonstrated to activate protein kinases in a variety of cell types; however, the outcomes of this activation have been proven to be dosage- and cell-type-dependent. In neural stem cells and some glial cells, activation of these kinases results in cell proliferation and migration [Citation98]. Thus, it can be concluded that curcumin-containing films may be advantageous in the wound-healing process based on cell viability and proliferation.
Figure 9. Fluorescence microscope images of cell viability for the (a) TCPP control sample (b) GG+SA and (c) GG+SA@CUR thin film cultured in the medium containing fibroblast cells for 24 h, 48 h and 72 h.

Moreover, the number of living cells was recorded as the highest in the cell treated with GG+SA@CUR thin film. After 72 h, the cell proliferation multiplies to 158,872 cells/well for GG+SA@CUR, 132,843 cells/well, and 54,446 cells/well for GG+SA and TCPP control samples. Before that, after 48 h of incubation, 104,491 cells/well were measured on GG+SA@CUR films, compared to 88,542 cells/well and 38,544 cells/well for the GG+SA and control samples, respectively. The number of cells was found to be 56,868, 40322, and 24,282, cells/well for the thin film of GG+SA@CUR, GG+SA, and control sample, respectively, after 24 h of incubation. It clearly shows that the GG+SA@CUR was helpful in progressively boosting cell viability and proliferation because the presence of CUR promotes angiogenesis [Citation99]. Thus, the outcome demonstrates that the manufactured GG+SA@CUR thin film is a biocompatible and acceptable material for application in skin wound healing.
In vitro wound healing scratch assays
The scratch wound healing test is a widely used method for studying mammalian cell migration and proliferation. In a typical scratch wound healing test, a ‘wound gap’ is created in a cell monolayer, and cell migration and proliferation then ‘heal’ this gap by closing it. The rate of gap closure can be influenced by factors that affect cell motility and proliferation. In this study, we observed the area of wound closure and cell migration. To assess wound healing and cell mobility, we measured the artificial wound area at 0, 8, and 24 h. Our findings showed that the GG+SA@CUR film exhibited a higher rate of fibroblast cell migration and wound healing, as depicted in . After 24 h, the GG+SA@CUR film successfully closed the wound gap (). Conversely, the pure GG+SA thin film () and the control sample () were unable to close the gap. Notably, the presence of CUR in the GG+SA@CUR film sample stimulated cell proliferation and migration, covering approximately 26% of the wound area after 8 h, compared to 18% for GG+SA and 12% for the control. Remarkably, after 24 h, the GG+SA@CUR film achieved an average wound closure rate of 98.4%, surpassing the control (64.6%) and pure GG+SA (72.8%). Visual evidence supports this, clearly illustrating the robust migration of fibroblast cells in the GG+SA@CUR film compared to the control and GG+SA thin films without CUR. This assay confirms the GG+SA@CUR thin film’s ability to promote cell migration and proliferation, rendering it a promising candidate for potential wound healing applications. The healing process involves well-controlled phases, including inflammation, granulation, tissue reconstruction, and collagen deposition [Citation100]. CUR can target each of these phases by suppressing pro-inflammatory cytokines, reducing inflammation, scavenging reactive oxygen species, activating growth factors for granulation, and stimulating fibroblast differentiation to enhance collagen deposition and wound healing [Citation101]. Numerous studies have demonstrated that CUR components can expedite the healing process by reducing inflammation, promoting cell proliferation, and enhancing re-epithelialization [Citation102,Citation103]. Additionally, CUR’s potent antioxidant properties help prevent tissue oxidation, further promoting efficient and rapid recovery [Citation104].
Figure 10. Illustrations of fibroblast cells moving into a scratch area at 0, 8 and 24 hours for (a) control sample and in the presence of (b) GG+SA, (c) GG + SA@CUR thin films.

Based on the obtained results, the performance of the GG+SA@CUR thin film for wound healing compares favourably with commercially available wound dressing materials such as Sorbsan™, Kaltostat™, and Algisite™ [Citation105]. However, to provide a comprehensive comparison, further in-vivo and clinical studies are necessary to directly assess the performance of the GG+SA@CUR thin film in wound healing. These studies would offer detailed insights and specific data regarding the comparative advantages and limitations of various wound dressing options. In the context of in vitro wound healing scratch assays, the GG+SA@CUR thin film exhibited a faster wound closure rate compared to other nanofibrous materials, including curcumin-loaded poly(lactic acid) blend hyperbranched polyglycerol (PLA/HPG@Cur), carboxymethyl guar gum mixed with polyvinyl alcohol potentiated with reduced graphene oxide (CMGG/PVA@rGO), and various other nanofibers, as indicated in .
Table 4. Performance of wound dressing for in vitro wound healing scratch assays.
Conclusion
In the present study, the GG+SA@CUR thin film has demonstrated remarkable potential for applications in wound healing. The research supports the successful encapsulation of CUR within the GG+SA biopolymer. Furthermore, the constructed thin film has displayed impressive free-radical scavenging capabilities and exhibited high antibacterial activity against both gram-positive and gram-negative bacteria. Extensive biocompatibility testing conducted on fibroblast growth has confirmed the thin film’s excellent biocompatibility. Notably, the complete closure of cell-scratched areas within 24 h strongly suggests that the GG+SA@CUR thin film holds promise as an effective wound dressing for promoting wound healing.
Acknowledgments
The authors are grateful to Universiti Malaysia Terengganu (UMT) for facilities and King Saud University, Riyadh, Saudi Arabia for the financial support vote (RSP2023R371).
Disclosure statement
No potential conflict of interest was reported by the authors.
Additional information
Funding
References
- Prakash J, Venkataprasanna KS, Bharath G, et al. In-vitro evaluation of electrospun cellulose acetate nanofiber containing Graphene oxide/TiO2/Curcumin for wound healing application. Colloids Surf A Physicochem Eng Asp. 2021;627:127166. doi: 10.1016/j.colsurfa.2021.127166
- Zhang M, Fan Z, Zhang J, et al. Multifunctional chitosan/alginate hydrogel incorporated with bioactive glass nanocomposites enabling photothermal and nitric oxide release activities for bacteria-infected wound healing. Int J Biol Macromol. 2023;232:123445. doi: 10.1016/j.ijbiomac.2023.123445
- Fan MH, Zhu Q, Li HH, et al. Fibroblast activation protein (FAP) accelerates collagen degradation and clearance from lungs in mice. J Biol Chem. 2016;291(15):8070–18. doi: 10.1074/jbc.M115.701433
- Zhu Y, Hoshi R, Chen S, et al. Sustained release of stromal cell derived factor-1 from an antioxidant thermoresponsive hydrogel enhances dermal wound healing in diabetes. J Control Release. 2016;238:114–122. doi: 10.1016/j.jconrel.2016.07.043
- Andrade S, Ramalho MJ, Loureiro JA, et al. Natural compounds for Alzheimer’s disease therapy: a systematic review of preclinical and clinical studies. Int J Mol Sci. 2019;20(9):2313. doi: 10.3390/ijms20092313
- Yen YH, Pu CM, Liu CW, et al. Curcumin accelerates cutaneous wound healing via multiple biological actions: the involvement of TNF‐α, MMP‐9, α‐SMA, and collagen. Int Wound J. 2018;15(4):605–617. doi: 10.1111/iwj.12904
- Anand P, Kunnumakkara AB, Newman RA, et al. Bioavailability of curcumin: problems and promises. Mol Pharm. 2007;4(6):807–818. doi: 10.1021/mp700113r
- Khan S, Imran M, Butt TT, et al. Curcumin based nanomedicines as efficient nanoplatform for treatment of cancer: new developments in reversing cancer drug resistance, rapid internalization, and improved anticancer efficacy. Trends Food Sci Technol. 2018;80:8–22. doi: 10.1016/j.tifs.2018.07.026
- Liu J, Chen Z, Wang J, et al. Encapsulation of curcumin nanoparticles with MMP9-responsive and thermos-sensitive hydrogel improves diabetic wound healing. ACS Appl Mater Inter. 2018;10(19):16315–16326. doi: 10.1021/acsami.8b03868
- Balan P, Mal G, Das S, et al. Synergistic and additive antimicrobial activities of curcumin, manuka honey and whey proteins. J Food Biochem. 2016;40(5):647–654. doi: 10.1111/jfbc.12249
- Dong Y, Rao Z, Liu Y, et al. Soluble soybean polysaccharide/gelatin active edible films incorporated with curcumin for oil packaging. Food Pack Shelf Life. 2023;35:101039. doi: 10.1016/j.fpsl.2023.101039
- Sharma G, Raturi K, Dang S, et al. Combinatorial antimicrobial effect of curcumin with selected phytochemicals on Staphylococcus epidermidis. J Asian Nat Prod Res. 2014;16(5):535–541. doi: 10.1080/10286020.2014.911289
- Varshney GK, Saini RK, Gupta PK, et al. Effect of curcumin on the diffusion kinetics of a hemicyanine dye, LDS-698, across a lipid bilayer probed by second harmonic spectroscopy. Langmuir. 2013;29(9):2912–2918. doi: 10.1021/la304778d
- Barry J, Fritz M, Brender JR, et al. Determining the effects of lipophilic drugs on membrane structure by solid-state NMR spectroscopy: the case of the antioxidant curcumin. J Am Chem Soc. 2009;131(12):4490–4498. doi: 10.1021/ja809217u
- Rai D, Singh JK, Roy N, et al. Curcumin inhibits FtsZ assembly: an attractive mechanism for its antibacterial activity. Biochem J. 2008;410(1):147–155. doi: 10.1042/BJ20070891
- Kaur S, Modi NH, Panda D, et al. Probing the binding site of curcumin in Escherichia coli and Bacillus subtilis FtsZ–a structural insight to unveil antibacterial activity of curcumin. Eur J Med Chem. 2010;45(9):4209–4214. doi: 10.1016/j.ejmech.2010.06.015
- Rudrappa T, Bais HP. Curcumin, a known phenolic from Curcuma longa, attenuates the virulence of Pseudomonas aeruginosa PAO1 in whole plant and animal pathogenicity models. J Agric Food Chem. 2008;56(6):1955–1962. doi: 10.1021/jf072591j
- Saha S, Pramanik K, Biswas A. Antibacterial activity and biocompatibility of curcumin/TiO2 nanotube array system on Ti6Al4V bone implants. Mater Technol. 2021;36(4):221–232. doi: 10.1080/10667857.2020.1742984
- Kharat M, Du Z, Zhang G, et al. Physical and chemical stability of curcumin in aqueous solutions and emulsions: impact of pH, temperature, and molecular environment. J Agric Food Chem. 2017;65(8):1525–1532. doi: 10.1021/acs.jafc.6b04815
- Hafez Ghoran S, Calcaterra A, Abbasi M, et al. Curcumin-based nanoformulations: a promising adjuvant towards cancer treatment. Molecules. 2022;27(16):5236. doi: 10.3390/molecules27165236
- Andrabi SM, Majumder S, Gupta KC, et al. Dextran based amphiphilic nano-hybrid hydrogel system incorporated with curcumin and cerium oxide nanoparticles for wound healing. Colloids Surf B Biointerfaces. 2020;195:111263. doi: 10.1016/j.colsurfb.2020.111263
- Shehzad A, Wahid F, Lee YS. Curcumin in cancer chemoprevention: molecular targets, pharmacokinetics, bioavailability, and clinical trials. Arch Pharm (Weinheim). 2010;343(9):489–499. doi: 10.1002/ardp.200900319
- Subramani PA, Panati K, Narala VR. Curcumin nanotechnologies and its anticancer activity. Nutr Cancer. 2017;69(3):381–393. doi: 10.1080/01635581.2017.1285405
- Wahlström B, Blennow G. A study on the fate of curcumin in the rat. Acta Pharmacol Toxicol. 1978;43(2):86–92. doi: 10.1111/j.1600-0773.1978.tb02240.x
- Li H, Yan L, Tang EK, et al. Synthesis of TPGS/curcumin nanoparticles by thin-film hydration and evaluation of their anti-colon cancer efficacy in vitro and in vivo. Front Pharmacol. 2019;10:769. doi: 10.3389/fphar.2019.00769
- Obeid MA, Alsaadi M, Aljabali AA. Recent updates in curcumin delivery. J Liposome Res. 2023;33(1):53–64. doi: 10.1080/08982104.2022.2086567
- Mahmoudi A, Kesharwani P, Majeed M, et al. Recent advances in nanogold as a promising nanocarrier for curcumin delivery. Colloids Surf B Biointerfaces. 2022;215:112481. doi: 10.1016/j.colsurfb.2022.112481
- Fernando IPS, Lee W, Han EJ, et al. Alginate-based nanomaterials: Fabrication techniques, properties, and applications. Chem Eng J. 2020;391:123823. doi: 10.1016/j.cej.2019.123823
- Ahmad A, Mubarak NM, Jannat FT, et al. A critical review on the synthesis of natural sodium alginate based composite materials: an innovative biological polymer for biomedical delivery applications. Processes. 2021;9(1):137. doi: 10.3390/pr9010137
- Hishamuddin NI, Razali MH, Mat Amin KA. Application of gellan gum biopolymer in biomedical applications: a review. Makara J Sci. 2022;26(1):2.
- Das M, Giri TK. Hydrogels based on gellan gum in cell delivery and drug delivery. J Drug Delivery Sci Technol. 2020;56:101586. doi: 10.1016/j.jddst.2020.101586
- Subbuvel M, Kavan P. Development and investigation of antibacterial and antioxidant characteristics of poly lactic acid films blended with neem oil and curcumin. J Appl Polym Sci. 2022;139(14):51891. doi: 10.1002/app.51891
- De Mohac LM, Caruana R, Cavallaro G, et al. Spray-drying, solvent-casting and freeze-drying techniques: a comparative study on their suitability for the enhancement of drug dissolution rates. Pharm Res. 2020;37(3):1–11. doi: 10.1007/s11095-020-2778-1
- Kakran M, Sahoo NG, Tan IL, et al. Preparation of nanoparticles of poorly water-soluble antioxidant curcumin by antisolvent precipitation methods. J Nanopart Res. 2012;14(3):1–11. doi: 10.1007/s11051-012-0757-0
- Liu J, Ismail NA, Yusoff M, et al. Physicochemical properties and antibacterial activity of gellan gum incorporating zinc oxide/carbon nanotubes bionanocomposite film for wound healing. Bioinorg Chem Appl. 2022;2022:1–12. doi: 10.1155/2022/3158404
- Poornima B, Korrapati PS. Fabrication of chitosan-polycaprolactone composite nanofibrous scaffold for simultaneous delivery of ferulic acid and resveratrol. Carbohydr Polym. 2017;157:1741–1749. doi: 10.1016/j.carbpol.2016.11.056
- Balouiri M, Sadiki M, Ibnsouda SK. Methods for in vitro evaluating antimicrobial activity: A review. J Pharm Anal. 2016;6(2):71–79. doi: 10.1016/j.jpha.2015.11.005
- gjgfjfj
- Kim Y, Park EJ, Kim TW, et al. Recent progress in drug release testing methods of biopolymeric particulate system. Pharmaceutics. 2021;13(8):1313. doi: 10.3390/pharmaceutics13081313
- Lao LL, Venkatraman SS, Peppas NA. Modeling of drug release from biodegradable polymer blends. Eur J Pharm Biopharm. 2008;70(3):796–803. doi: 10.1016/j.ejpb.2008.05.024
- Mosmann T. Rapid colorimetric assay for cellular growth and survival: application to proliferation and cytotoxicity assays. J Immunol Methods. 1983;65(1–2):55–63. doi: 10.1016/0022-1759(83)90303-4
- Doostan M, Doostan M, Mohammadi P, et al. Wound healing promotion by flaxseed extract-loaded polyvinyl alcohol/chitosan nanofibrous scaffolds. Int j biol macromol. 2023;228:506–516. doi: 10.1016/j.ijbiomac.2022.12.228
- You C, Li Q, Wang X, et al. Silver nanoparticle loaded collagen/chitosan scaffolds promote wound healing via regulating fibroblast migration and macrophage activation. Sci Rep. 2017;7(1):10489. doi: 10.1038/s41598-017-10481-0
- Radstake WE, Gautam K, Van Rompay C, et al. Comparison of in vitro scratch wound assay experimental procedures. Biochem Biophys Rep. 2023;33:101423. doi: 10.1016/j.bbrep.2023.101423
- Postolović K, Ljujić B, Kovačević MM, et al. Optimization, characterization, and evaluation of carrageenan/alginate/poloxamer/curcumin hydrogel film as a functional wound dressing material. Mater Today Commun. 2022;31:103528. doi: 10.1016/j.mtcomm.2022.103528
- Ismail NA, Amin KAM, Majid FAA, et al. Gellan gum incorporating titanium dioxide nanoparticles biofilm as wound dressing: physicochemical, mechanical, antibacterial properties and wound healing studies. Mater Sci Eng C. 2019;103:109770. doi: 10.1016/j.msec.2019.109770
- Razali MH, Ismail NA, Amin KAM. Titanium dioxide nanotubes incorporated gellan gum bio-nanocomposite film for wound healing: Effect of TiO2 nanotubes concentration. Int J Biol Macromol. 2020;153:1117–1135. doi: 10.1016/j.ijbiomac.2019.10.242
- Ismail NA, Amin KAM, Razali MH. Novel gellan gum incorporated TiO2 nanotubes film for skin tissue engineering. Mater Lett. 2018;228:116–120. doi: 10.1016/j.matlet.2018.05.140
- Badita CR, Aranghel D, Burducea C, et al. Characterization of sodium alginate based films. Rom J Phys. 2020;65:1–8.
- Larosa C, Salerno M, de Lima JS, et al. Characterisation of bare and tannase-loaded calcium alginate beads by microscopic, thermogravimetric, FTIR and XRD analyses. Int J Biol Macromol. 2018;115:900–906. doi: 10.1016/j.ijbiomac.2018.04.138
- Kirupha SD, Elango S, Vadodaria K. Fabrication of nanofibrous membrane using stingless bee honey and curcumin for wound healing applications. J Drug Delivery Sci Technol. 2021;63:102271. doi: 10.1016/j.jddst.2020.102271
- Dong K, Xu K, Wei N, et al. Three-dimensional porous sodium alginate/gellan gum environmentally friendly aerogel: preparation, characterization, adsorption, and kinetics studies. Chem Eng Res Des. 2022;179:227–236. doi: 10.1016/j.cherd.2022.01.027
- Busto F, Licini C, Luccarini A, et al. Oleuropein-rich gellan gum/alginate films as innovative treatments against photo-induced skin aging. Molecules. 2023;28(11):4352. doi: 10.3390/molecules28114352
- Zia KM, Tabasum S, Khan MF, et al. Recent trends on gellan gum blends with natural and synthetic polymers: a review. Int J Biol Macromol. 2018;109:1068–1087. doi: 10.1016/j.ijbiomac.2017.11.099
- Das S, Mondal S. Formulation and in vitro study of Ibuprofen loaded crosslinked sodium alginate and gellan gum microspheres. Int J Adv Pharm. 2017;6(3):86–90.
- Yang H, Wang W, Zhang J, et al. Preparation, characterization, and drug-release behaviors of a pH-sensitive composite hydrogel bead based on guar gum, attapulgite, and sodium alginate. Int J Polym Mater Polym Biomater. 2013;62(7):369–376. doi: 10.1080/00914037.2012.706839
- Xing J, Peng X, Li A, et al. Gellan gum/alginate-based Ca-enriched acellular bilayer hydrogel with robust interface bonding for effective osteochondral repair. Carbohydr Polym. 2021;270:118382. doi: 10.1016/j.carbpol.2021.118382
- Mahmoud R, Safwat N, Fathy M, et al. Novel anti-inflammatory and wound healing controlled released LDH-curcumin nanocomposite via intramuscular implantation, in-vivo study. Arabian J Chem. 2022;15(3):103646. doi: 10.1016/j.arabjc.2021.103646
- Niranjan R, Kaushik M, Prakash J, et al. Enhanced wound healing by PVA/Chitosan/curcumin patches: in vitro and in vivo study. Colloids Surf B Biointerfaces. 2019;182:110339. doi: 10.1016/j.colsurfb.2019.06.068
- Rosland Abel SE, Yusof YA, Chin NL, et al. Characterisation of physicochemical properties of gum arabic powder at various particle sizes. Food Res. 2020;4(S1):107–115. doi: 10.26656/fr.2017.4(S1).S32
- Makhado E, Hato MJ. Preparation and characterization of sodium alginate-based oxidized multi-walled carbon nanotubes hydrogel nanocomposite and its adsorption behaviour for methylene blue dye. Front Chem. 2021;9:576913. doi: 10.3389/fchem.2021.576913
- Hosseini-Zare MS, Sarhadi M, Zarei M, et al. Synergistic effects of curcumin and its analogs with other bioactive compounds: a comprehensive review. Eur J Med Chem. 2021;210:113072. doi: 10.1016/j.ejmech.2020.113072
- Rezagholizade-Shirvan A, Najafi MF, Behmadi H, et al. Preparation of nano-composites based on curcumin/chitosan-PVA-alginate to improve stability, antioxidant, antibacterial and anticancer activity of curcumin. Inorg Chem Commun. 2022;145:110022. doi: 10.1016/j.inoche.2022.110022
- Mohammadian M, Salami M, Moghadam M, et al. Mung bean protein as a promising biopolymeric vehicle for loading of curcumin: Structural characterization, antioxidant properties, and in vitro release kinetics. J Drug Delivery Sci Technol. 2021;61:102148. doi: 10.1016/j.jddst.2020.102148
- Casadey R, Broglia M, Barbero C, et al. Controlled release systems of natural phenolic antioxidants encapsulated inside biocompatible hydrogels. React Funct Polym. 2020;156:104729. doi: 10.1016/j.reactfunctpolym.2020.104729
- Geevarghese AV, Kasmani FB, Dolatyabi S. Curcumin and curcumin nanoparticles counteract the biological and managemental stressors in poultry production: an updated review. Res Vet Sci. 2023;162:104958. doi: 10.1016/j.rvsc.2023.104958
- Cai X, He Y, Cai L, et al. An injectable elastic hydrogel crosslinked with curcumin–gelatin nanoparticles as a multifunctional dressing for the rapid repair of bacterially infected wounds. Biomater Sci. 2023;11(9):3227–3240. doi: 10.1039/D2BM02126A
- Roy S, Min SJ, Biswas D, et al. Pullulan/chitosan-based functional film incorporated with curcumin-integrated chitosan nanoparticles. Colloids Surf A Physicochem Eng Asp. 2023;660:130898. doi: 10.1016/j.colsurfa.2022.130898
- Łupina K, Kowalczyk D, Lis M, et al. Antioxidant polysaccharide/gelatin blend films loaded with curcumin—A comparative study. Int J Biol Macromol. 2023;236:123945. doi: 10.1016/j.ijbiomac.2023.123945
- Meiguni MSM, Salami M, Rezaei K, et al. Fabrication and characterization of a succinyl mung bean protein and arabic gum complex coacervate for curcumin encapsulation. Int J Biol Macromol. 2023;224:170–180. doi: 10.1016/j.ijbiomac.2022.10.113
- Nasiri SS, Ahmadi Z, Afshar-Taromi F. Design and characterization of poly (glycerol sebacate)/Poly (3-hydroxybutyrate)/bioglass/curcumin nanocomposite scaffold for wound healing application. Int J Biol Macromol. 2023;245:125521. doi: 10.1016/j.ijbiomac.2023.125521
- Duan M, Sun J, Huang Y, et al. Electrospun gelatin/chitosan nanofibers containing curcumin for multifunctional food packaging. Food Sci Hum Wellness. 2023;12(2):614–621. doi: 10.1016/j.fshw.2022.07.064
- Rashid N, Khalid SH, Ullah Khan I, et al. Curcumin-loaded bioactive polymer composite film of PVA/Gelatin/Tannic acid Downregulates the Pro-inflammatory Cytokines to expedite healing of full-thickness wounds. ACS Omega. 2023;8(8):7575–7586. doi: 10.1021/acsomega.2c07018
- Kour P, Afzal S, Gani A, et al. Effect of nanoemulsion-loaded hybrid biopolymeric hydrogel beads on the release kinetics, antioxidant potential and antibacterial activity of encapsulated curcumin. Food Chem. 2022;376:131925. doi: 10.1016/j.foodchem.2021.131925
- Kenawy ERS, Kamoun EA, Ghaly ZS, et al. Novel physically cross-linked curcumin-loaded PVA/aloe vera hydrogel membranes for acceleration of topical wound healing: in vitro and in vivo experiments. Arab J Sci Eng. 2023;48(1):497–514. doi: 10.1007/s13369-022-07283-6
- Alizadeh N, Malakzadeh S. Antioxidant, antibacterial and anti-cancer activities of β-and γ-CDs/curcumin loaded in chitosan nanoparticles. Int J Biol Macromol. 2020;147:778–791. doi: 10.1016/j.ijbiomac.2020.01.206
- Zheng D, Huang C, Huang H, et al. Antibacterial mechanism of curcumin: A review. Chem Biodivers. 2020;17(8):e2000171. doi: 10.1002/cbdv.202000171
- Nakagawa S, Matsumoto M, Katayama Y, et al. Staphylococcus aureus virulent PSMα peptides induce keratinocyte alarmin release to orchestrate IL-17-dependent skin inflammation. Cell Host & Microbe. 2017;22(5):667–677.e5. doi: 10.1016/j.chom.2017.10.008
- Selvam C, Prabu SL, Jordan BC, et al. Molecular mechanisms of curcumin and its analogs in colon cancer prevention and treatment. Life Sci. 2019;239:117032. doi: 10.1016/j.lfs.2019.117032
- Krausz AE, Adler BL, Cabral V, et al. Curcumin-encapsulated nanoparticles as innovative antimicrobial and wound healing agent. Nanomedicine. 2015;11(1):195–206. doi: 10.1016/j.nano.2014.09.004
- Guo LY, Yan SZ, Tao X, et al. Evaluation of hypocrellin A-loaded lipase sensitive polymer micelles for intervening methicillin-resistant Staphylococcus aureus antibiotic-resistant bacterial infection. Mater Sci Eng C. 2020;106:110230. doi: 10.1016/j.msec.2019.110230
- Tyagi P, Singh M, Kumari H, et al. Bactericidal activity of curcumin I is associated with damaging of bacterial membrane. PLoS One. 2015;10(3):e0121313. doi: 10.1371/journal.pone.0121313
- Liu J, Xu L, Liu C, et al. Preparation and characterization of cationic curcumin nanoparticles for improvement of cellular uptake. Carbohydr Polym. 2012;90(1):16–22. doi: 10.1016/j.carbpol.2012.04.036
- Fathi M, Emam-Djomeh Z, Aliabbasi N. Developing two new types of nanostructured vehicles to improve biological activity and functionality of curcumin. Food Biosci. 2021;44:101386.87. doi: 10.1016/j.fbio.2021.101386
- Sari TP, Mann B, Kumar R, et al. Preparation and characterization of nanoemulsion encapsulating curcumin. Food Hydrocolloids. 2015;43:540–546.89. doi: 10.1016/j.foodhyd.2014.07.011
- Li R, Lin Z, Zhang Q, et al. Injectable and in situ-formable thiolated chitosan-coated liposomal hydrogels as curcumin carriers for prevention of in vivo breast cancer recurrence. ACS Appl Mater Inter. 2020;12(15):17936–17948. doi: 10.1021/acsami.9b21528
- Samadi A, Haseli S, Pourmadadi M, et al., 2020, November. Curcumin-loaded chitosan-agarose-montmorillonite hydrogel nanocomposite for the treatment of breast cancer. In 2020 27th National and 5th International Iranian Conference on Biomedical Engineering (ICBME), Tehran, Iran (pp. 148–153). IEEE.
- Peng S, Li Z, Zou L, et al. Improving curcumin solubility and bioavailability by encapsulation in saponin-coated curcumin nanoparticles prepared using a simple pH-driven loading method. Food Funct. 2018;9(3):1829–1839. doi: 10.1039/C7FO01814B
- Mandal B, Rameshbabu AP, Soni SR, et al. In situ silver nanowire deposited cross-linked carboxymethyl cellulose: a potential transdermal anticancer drug carrier. ACS Appl Mater Inter. 2017;9(42):36583–36595. doi: 10.1021/acsami.7b10716
- Rajaei H, Mofazzal Jahromi MA, Khoramabadi N, et al. Immunoregulatory properties of arteether in folic acid-chitosan-Fe3O4 composite nanoparticle in 4T1 cell line and mice bearing breast cancer. Immunoregulation. 2020;2(2):89–102. doi: 10.32598/IMMUNOREGULATION.1.4.207
- Deka R, Sarmah JK, Baruah S, et al. An okra polysaccharide (abelmoschus esculentus) reinforced green hydrogel based on guar gum and poly-vinyl alcohol double network for controlled release of nanocurcumin. Int J Biol Macromol. 2023;234:123618. doi: 10.1016/j.ijbiomac.2023.123618
- Sundar S, Mariappan R, Piraman S. Synthesis and characterization of amine modified magnetite nanoparticles as carriers of curcumin-anticancer drug. Powder Technol. 2014;266:321–328. doi: 10.1016/j.powtec.2014.06.033
- Mosallanezhad P, Nazockdast H, Ahmadi Z, et al. Fabrication and characterization of polycaprolactone/chitosan nanofibers containing antibacterial agents of curcumin and ZnO nanoparticles for use as wound dressing. Front Bioeng Biotechnol. 2022;10:59–72. doi: 10.3389/fbioe.2022.1027351
- Shahrousvand M, Hajikhani M, Nazari L, et al. Preparation of colloidal nanoparticles PVA-PHEMA from hydrolysis of copolymers of PVAc-PHEMA as anticancer drug carriers. Nanotechnology. 2022;33(27):275603. doi: 10.1088/1361-6528/ac6089
- Babaluei M, Mottaghitalab F, Seifalian A, et al. Injectable multifunctional hydrogel based on carboxymethylcellulose/polyacrylamide/polydopamine containing vitamin C and curcumin promoted full-thickness burn regeneration. Int J Biol Macromol. 2023;236:124005. doi: 10.1016/j.ijbiomac.2023.124005
- Karri VVSR, Kuppusamy G, Talluri SV, et al. Curcumin loaded chitosan nanoparticles impregnated into collagen-alginate scaffolds for diabetic wound healing. Int J Biol Macromol. 2016;93:1519–1529. doi: 10.1016/j.ijbiomac.2016.05.038
- Zhang M, Zhang J, Chen J, et al. Fabrication of curcumin modified TiO2 nanoarrays via cyclodextrin based polymer functional coatings for osteosarcoma therapy. Adv Healthcare Mater. 2019;8(23):1901031. doi: 10.1002/adhm.201901031
- Ma J, Yu H, Liu J, et al. Curcumin promotes nerve regeneration and functional recovery after sciatic nerve crush injury in diabetic rats. Neurosci lett. 2016;610:139–143. doi: 10.1016/j.neulet.2015.11.005
- Thangapazham RL, Sharad S, Maheshwari RK. Skin regenerative potentials of curcumin. BioFactors. 2013;39(1):141–149. doi: 10.1002/biof.1078
- Sinno H, Prakash S. Complements and the wound healing cascade: an updated review. Plast Surg Int. 2013;2013:1–7. doi: 10.1155/2013/146764
- Akbik D, Ghadiri M, Chrzanowski W, et al. Curcumin as a wound healing agent. Life Sci. 2014;116(1):1–7. doi: 10.1016/j.lfs.2014.08.016
- Bahramsoltani R, Farzaei MH, Rahimi R. Medicinal plants and their natural components as future drugs for the treatment of burn wounds: an integrative review. Arch Dermatol Res. 2014;306(7):601–617. doi: 10.1007/s00403-014-1474-6
- Rezkita F, Wibawa KG, Nugraha AP. Curcumin loaded chitosan nanoparticle for accelerating the post extraction wound healing in diabetes mellitus patient: a review. Res J Pharm Technol. 2020;13(2):1039–1042. doi: 10.5958/0974-360X.2020.00191.2
- Phan TT, See P, Lee ST, et al. Protective effects of curcumin against oxidative damage on skin cells in vitro: its implication for wound healing. J Trauma Acute Care Surg. 2001;51(5):927–931. doi: 10.1097/00005373-200111000-00017
- Laurano R, Boffito M, Ciardelli G, et al. Wound dressing products: a translational investigation from the bench to the market. Eng Regener. 2022;3(2):182–200. doi: 10.1016/j.engreg.2022.04.002
- Perumal G, Pappuru S, Chakraborty D, et al. Synthesis and characterization of curcumin loaded PLA—hyperbranched polyglycerol electrospun blend for wound dressing applications. Mater Sci Eng C. 2017;76:1196–1204. doi: 10.1016/j.msec.2017.03.200
- Koyyada A, Orsu P. Nanofibrous scaffolds of carboxymethyl guargum potentiated with reduced graphene oxide for in vitro and in vivo wound healing applications. Int J Pharmaceut. 2021;607:121035. doi: 10.1016/j.ijpharm.2021.121035
- Abolghasemzade S, Pourmadadi M, Rashedi H, et al. PVA based nanofiber containing CQDs modified with silica NPs and silk fibroin accelerates wound healing in a rat model. J Mat Chem B. 2021;9(3):658–676. doi: 10.1039/D0TB01747G
- Nemati D, Ashjari M, Rashedi H, et al. PVA based nanofiber containing cellulose modified with graphitic carbon nitride/nettles/trachyspermum accelerates wound healing. Biotechnol Prog. 2021;37(6):e3200. doi: 10.1002/btpr.3200
- Szymańska E, Wojasiński M, Czarnomysy R, et al. Chitosan-enriched solution blow spun poly (ethylene oxide) nanofibers with poly (dimethylsiloxane) hydrophobic outer layer for skin healing and regeneration. Int J Mol Sci. 2022;23(9):5135. doi: 10.3390/ijms23095135