Abstract
Currently, traumatic brain injury (TBI) is the leading cause of death or disabilities in young individuals worldwide. The multi-complexity of its pathogenesis as well as impermeability of the blood–brain barrier (BBB) makes the drug choice and delivery very challenging. The brain-derived neurotrophic factor (BDNF) regulates neuronal plasticity, neuronal cell growth, proliferation, cell survival and long-term memory. However, its short half-life and low BBB permeability are the main hurdles to be an effective therapeutic for TBI. Poly (lactic-co-glycolic acid) (PLGA) nanoparticles coated by surfactant can enable the delivery of a variety of molecules across the BBB by receptor-mediated transcytosis. This study examines the ability of PLGA nanoparticles coated with poloxamer 188 (PX) to deliver BDNF into the brain and neuroprotective effects of BNDF in mice with TBI. C57bl/6 mice were subjected to weight-drop closed head injuries under anesthesia. Using enzyme-linked immunosorbent assay, we demonstrated that the intravenous (IV) injection of nanoparticle-bound BDNF coated by PX (NP-BDNF-PX) significantly increased BDNF levels in the brain of sham-operated mice (p < 0.001) and in both ipsi- (p < 0.001) and contralateral (p < 0.001) parts of brain in TBI mice compared to controls. This study also showed using the passive avoidance (PA) test, that IV injection of NP-BDNF-PX 3 h post-injury prolonged the latent time in mice with TBI thereby reversing cognitive deficits caused by brain trauma. Finally, neurological severity score test demonstrated that our compound efficiently reduced the scores at day 7 after the injury indicating the improvement of neurological deficit in animals with TBI. This study shows that PLGA nanoparticles coated with PX effectively delivered BDNF into the brain, and improved neurological and cognitive deficits in TBI mice, thereby providing a neuroprotective effect.
Introduction
Traumatic brain injury (TBI) is a term referring to brain dysfunction triggered by an external force. Currently, it is the leading cause of death or disability in individuals aged below 40 years worldwide (Grafman & Salazar, Citation2015). The physiological pattern of TBI includes primary and secondary damage of brain tissue. Primary injury is defined as the immediate consequence of a mechanical impact that results in direct damaging of neurons, axons, glia and blood vessels, thereby initiating acute neurodegeneration (Graham DI & Mcintosh, Citation2002). Secondary injury is a cascade of delayed, potentially reversible, reactions which follow the primary injury. These reactions include ischemic damage, neuroinflammation, neurotoxicity, oxidative stress, apoptosis and traumatic axonal injury (TAI). The outcome of secondary brain injury is chronic neurodegeneration, which is associated with neurological and cognitive disorders that increase the risk for the development of such neurodegenerative diseases as Alzhiemer’s disease, Parkinson’s disease and amyotrophic lateral sclerosis (ALS) (Graham DI & Mcintosh, Citation2002). While the treatment of primary TBI has been fairly well established and evidence-based therapy has led to substantial reduction in mortality as well as improved TBI outcomes (Guidelines, Citation2007), management of secondary TBI remains an ongoing challenge. Despite a number of advanced pre-clinical studies, to date all clinical trials for the treatment of secondary TBI have failed (Kabadi & Faden, Citation2014).
Brain-derived neurotrophic factor (BDNF) is a neurotrophin that is responsible for the regulation of neuronal plasticity, neuronal cell growth, proliferation, cell survival and long-term memory (Allen et al., Citation2013). Interestingly, studies have shown an increase in expression of BDNF mRNA acute after experimental brain trauma (Oyesiku et al., Citation1999; Griesbach et al., Citation2002) that might provide an endogenous neuroprotection. This makes BDNF to be an excellent candidate for development of new strategies for the management of TBI as BDNF may provide a protection and repairing of neurons and restore the axonal connectivity in injured areas. Other studies, however, indicated that endogenous BDNF had transient but ultimately a failed protective response (Hicks et al., Citation1998). It stands to reason that efficacious neuroprotective treatment of brain trauma could be strengthened by exogenously enhancing the concentration of BDNF in central nervous system (CNS). On the other hand, preclinical studies devoted to treatment of TBI using BDNF resulted in fairly contrasting data (Blaha et al., Citation2000; Mahmood et al., Citation2002). At the same time, numerous successful preclinical studies established BDNF as a therapeutic tool in many other neurodegenerative diseases of the brain as well as the retina and the inner ear (Khalin et al., Citation2015). Nevertheless, despite promising therapeutic properties of BDNF in vivo, the recombinant BDNF infused subcutaneously or intrathecally to patients with ALS had failed in a phase III clinical trial (Group, Citation1999). It subsequently has been shown that poor pharmacokinetic properties of BDNF, including its short half-life and low blood–brain barrier (BBB) permeability, are the main hurdles in both clinical and in vivo studies (Poduslo & Curran, Citation1996).
In 1995, Kreuter and Alyautdin demonstrated that poly (butyl cyanoacrylate) (PBCA) nanoparticles (NPs) coated with polysorbate 80 are able to deliver the hexapeptide dalargine across the BBB in vivo (Kreuter et al., Citation1995). Subsequently, many substances were successfully delivered to the brain using PBCA NPs (Alyautdin & Kreuter, Citation2000), namely loperamide (Alyautdin et al., Citation1997), tubocurarine (Alyautdin et al., Citation1998), doxorubicine (Steiniger et al., Citation2004) and even nerve growth factor (Kurakhmaeva et al., Citation2009). It was shown that surfactant coatings play a crucial role in the delivery of molecules to the brain (Schroeder et al., Citation2000). In fact, in vitro experiments revealed that polysorbate 80 or poloxamer 188 are able to serve as an anchor for apolipoproteins (Petri et al., Citation2007). Wagner at al. showed that apolipoprotein E (ApoE) is responsible for NPs penetration by interaction with the low-density lipoprotein receptor LRP1 located on the endotheliocytes surface of the BBB (Wagner et al., Citation2012). Further, it was demonstrated that human serum albumin NPs with covalently bound ApoE were able to penetrate into CNS to a greater extent than NPs coated with polysorbate 80 (Michaelis et al., Citation2006). Thus, NPs coated with certain surfactants are capable of adsorbing endogenic low-density lipoprotein (LDL) ApoE from plasma; this compound then interacts with LDL receptors on the surface of brain endotheliocytes thereby providing transport across the BBB (Wohlfart et al., Citation2012). Moreover, surfactant coating decreases clearance of nanoparticles by the reticuloendothelial system in that way increasing the circulation time of the compound. The NPs are then transported across the BBB either via endocytosis by the endothelial cells (with subsequent release of the drug within the cells and diffusion into the brain) or dominantly by transcytosis across the endothelium. Such “Trojan horse” drug-delivery systems allow the targeting of a drug into the brain as well as prolonging the drug half-life.
Poly (lactic-co-glycolic acid) (PLGA) is a well-established biocompatible and biodegradable copolymer made by copolymerization of two different monomers, the cyclic dimers (1,4-dioxane-2,5-diones) of glycolic acid and lactic acid. PLGA NPs have a low-systemic toxicity, good mechanical properties and quite ease of managing (Sharma et al., Citation2012). It was demonstrated that modified PLGA NPs were capable of penetrating through BBB along with a cargo (Tosi et al., Citation2010). PLGA has also been approved by FDA for human treatment, diagnostics and other applications of clinical and basic science research (Lu et al., Citation2009, Grazu, Citation2012). Importantly, poloxamer 188 (PX) is significantly more apt for PLGA NPs to provide a good penetration into the CNS in comparison with other surfactants (Gelperina et al., Citation2010).
As such, in present study, we investigated possibility of a delivery of BDNF into the CNS using PLGA NPs coated with PX and efficacy of the compound to serve as an effective neuroprotectant for the treatment of TBI.
Materials and methods
Materials
Recombinant BDNF (Antibodies-online GmbH, Aachen, Germany), phosphate-buffered saline (PBS) (Sigma-Aldrich, St. Louis, MO), 10% poloxamer 188 (Sigma-Aldrich, St. Louis, MO), PLGA nanoparticles 200 nm (Phosphorex Inc., Hopkinton, MA), sodium pentobarbital (Dorminal, Alfasan, Holland), fentanyl (Talgesil, Duopharma(M), Klang, Malaysia).
Preparation of the drug formulations
BDNF solution
The lyophilized recombinant BDNF was dissolved in PBS at a concentration of 25 μg/ml.
Solution of BDNF with poloxamer 188
To prepare a 1% poloxamer 188 solution with BDNF, 0.14 ml of 10% poloxamer 188 was dissolved in 1.26 ml of stock BDNF solution.
Drug-free 2% PLGA nanoparticle dispersion
To obtain a 2% PLGA homogenous dispersion, 28 mg of freeze-dried PLGA nanoparticles were dispensed in 1.4 ml PBS solution and subjected to sonication (SOLTEC®, Milano, Italy) for 60 s in two cycles.
Preparation of nanoparticle dispersion with adsorbed BDNF
Twenty eight milligram of freeze-dried PLGA nanoparticles were dispersed in 0.8 mL of PBS solution to obtain a homogenous 2% dispersion as described above. The lyophilized BDNF (40 μg) was dissolved in 0.8 mL of PBS solution and added into the nanoparticle dispersion. The mixture was then incubated at 25 ± 1 °C for 4 h by continuous magnetic stirring (Favorit, Kuala Lumpur, Malaysia). The resultant dispersion (1.6 mL) contained 25 μg/mL of BDNF in 2% dispersion of PLGA nanoparticles.
Poloxamer 188-coated nanoparticles dispersion with adsorbed BDNF
Twenty eight mg of freeze-dried PLGA nanoparticles were dispersed in 0.64 mL PBS solution to obtain a homogenous 2% suspension. The lyophilized BDNF (40 μg) was dissolved in 0.8 mL of PBS solution and added into the nanoparticle dispersion. The mixture was then incubated at 25 ± 1 °C for 4 h with continuous magnetic stirring. Subsequently, a 10% solution of poloxamer 188 (0.16 ml) was added to the mixture, and the mixture was incubated for an additional 30 min at the same temperature. The resultant dispersion (1.6 mL) contained 25 μg/mL of BDNF in 2% dispersion of PLGA nanoparticles with 1% of poloxamer 188.
Determination of BDNF loading
The drug loading of the PLGA-NPs was calculated as the difference between total amount of drug in dispersion and amount of unbound drug. For this purpose, the unbound drug was collected by filtration through a membrane filter (MILLEX®GS, pore size = 220 nm, diameter = 33 mm; Merck Milipore, Carrigtwohill, Ireland) and its concentration in the filtrate was measured with BDNF Human ELISA kit (Abcam, Cambridge, UK) (Wallace et al., Citation2012). Duplicates were conducted and the results were averaged.
Animals
Male C57BL/6N mice (In Vivos®, Singapore, Singapore) 11–13 weeks old, weighing 25–28 g were used in order to avoid biases since variations of estrogen levels seen in female may influence our data (Singh et al., Citation2008). The animals were kept in groups of six in individually ventilated cages (Techniplast®, Buguggiate, Italy) at 20–23 °C with a 12-h light/dark cycle with free access to water and food (Teklad Global Diets®, Harlan, Indianapolis, IN). To minimize possible drawbacks due to circadian rhythm effects, all manipulations began in the morning (8–9 a.m.) after the beginning of the light cycle. All laboratory procedures were conducted in accordance with the European Convention for the protection of vertebrate animals (Strasbourg, 03.18.86), Declaration of Helsinki and the Guide for the Care and Use of Laboratory and had been approved by institutional animal care and use committee.
Brain trauma
Among the different types of weight-drop TBI models, the closed head injury (CHI) (Flierl et al., Citation2009) is considered to be one of the experimental methods relevant for the clinical condition in human CHI (Xiong et al., Citation2013). The weight-drop apparatus was developed in our laboratory in accordance to the standardized device as described in our previous work (Khalin et al., Citation2016). Following general anesthesia with intraperitoneal (IP) injection of 60 mg/kg sodium pentobarbital, a midline incision over the skull was performed. The skin was retracted, and the skull was exposed to locate the area of impact. The head was manually fixed on the platform at the bottom of the weight-drop device. The 3 mm diameter plastic tip of a metal rod weighing 250 g was gently advanced onto the exposed mouse skull at the left anterior frontal area. The head was held in place manually while the rod was lifted up to a height of 2 cm and held by a magnet. The trauma was induced by pressing the pedal which switched off the magnet and the rod felt onto the mouse skull. Immediately, oxygen (4 liters O2 per min) was being supplied to the mice for the next 2 min. Later, IP injection of 0.05 mg/kg fentanyl was given and the mice were returned to their cages. Sham controls received anesthesia and skin incision only.
Evaluation of the mouse brain BDNF level
For the determination of the BDNF concentration in the brain, sham-operated mice and mice with brain trauma were used. General anesthesia was performed by IP injection of 60 mg/kg sodium pentobarbital. Brain trauma was induced as described above with sham-operated mice receiving anesthesia and skin incision only. Thirty sham-operated male C57BL/6 mice and 30 male C57BL/6 mice with TBI were divided in 5 groups (n = 6). Three hours post-injury, the mice were administered intravenously 0.2 ml of the following solutions: group 1: solution of PBS; group 2: solution of recombinant BDNF (5 μg/mouse) in PBS (PBS + BDNF); group 3: solution of recombinant BDNF (5 μg/mouse) in PBS with 1% poloxamer 188 (PBS + BDNF-PX); group 4: suspension of 2% PLGA nanoparticles with adsorbed BDNF (5 μg/mouse) on their surface (PBS + NP-BDNF); group 5: suspension of 2% PLGA with adsorbed BDNF (5 μg/mouse) on their surface and coated by 1% poloxamer 188 (PBS + NP-BDNF-PX). Following 1 h drug injection, mice were killed by cervical dislocation and brain tissues were immediately harvested from injured site and contralateral site or left hemisphere in sham animals. Appropriate brain regions were weighed, snap frozen in liquid nitrogen then stored at −80 °C. Within two weeks, the brain samples were placed in sterile PBS containing a protease inhibitor cocktail (Sigma-Aldrich, St. Louis, MO), homogenized, sonicated and centrifuged (Rotina 380R, Hettich, Kirchlengern, Germany) at 11,000 rpm for 20 min at 4 °C. The supernatant was then removed, aliquoted and stored at −20 °C till analysis. Protein concentrations of all samples were measured using a BCA protein assay kit (BioVision Inc., Milpitas, Canada) and BDNF levels were measured with BDNF Human ELISA kit (Abcam, Cambridge, United Kingdom) and expressed as pg/mg protein.
Drug treatment scheme in the neurological severity score and passive avoidance test experiments
Three hours post-injury, mice were subjected to IV injection of 0.2 ml as follows: group 1: PBS; group 2: PBS + BDNF; group 3: PBS + BDNF-PX; group 4: suspension of empty poly(lactic-co-glycolic acid) (PLGA) nanoparticles (NP); group 5: PBS + NP-BDNF; group 6: PBS + NP-BDNF-PX.
Neurological severity score (NSS)
The NSS test was described in our previous work (Khalin et al., Citation2016). Briefly, the test was performed at 1, 4, 24, 48, 72 h and at 7th day after CHI. This test includes 10 tasks (Flierl et al., Citation2009) to assess motor, reflex and balance ability of the mouse (). One point was given when mouse was unable to perform the tasks and no points were given if the task was done with success.
Table 1. Neurological severity score test.
Passive avoidance
The passive avoidance (PA) task was performed to evaluate cognitive impairments. This step-through method was described in our previous work (Khalin et al., Citation2016). To perform this task, a PA apparatus (Ugo Basile, Varese, Italy) was used (Quertemont et al., Citation2004). Our previous data revealed that posttraumatic memory deficit after 250 g, 2 cm TBI was detected on day 7 (Khalin et al., Citation2016). Based on these results, we evaluated TBI-induced amnesia in current work at day 7 as well.
Statistical analysis
Data are presented as the mean and standard error of the mean (mean ± SEM). Statistical analysis was performed using the GraphPad Prizm® 6 software (La Jolla, CA) where two-way and one-way ANOVA followed by Bonferroni post-tests was used. Statistical significance was kept at p < 0.05.
Results
BDNF loading
About 97% of BDNF was adsorbed onto the PLGA-NPs validating the good drug loading () after addition to the NPs and equilibration.
BDNF brain concentrations
To evaluate the ability of surfactant-coated PLGA nanoparticles to deliver BDNF into the brain, we determined the brain levels of BDNF 1 h post-injection after 3 h of induced brain injury. illustrates brain levels of BDNF in sham-operated and TBI animals.
Figure 2. A bar graphs depicting brain-derived neurotrophic factor (BDNF) concentrations (pg/mg protein) measured by enzyme-linked immunosorbent assay (ELISA) in the brain of male C57Bl/6 mice at one hour post-injection of phosphate-buffered saline (PBS); solution of recombinant BDNF in PBS (PBS + BDNF); solution of recombinant BDNF in PBS with poloxamer 188 (PBS + BDNF + PX); suspension of poly(lactic-co-glycolic acid) nanoparticles (NP) with adsorbed BDNF on their surface (PBS + NP + BDNF); suspension of NP with adsorbed BDNF on their surface and coated by poloxamer 188 (PBS + NP + BDNF + PX). Sham: left brain hemisphere of sham-operated animals; Ipsi: ipsilateral brain hemisphere of animals with traumatic brain injury (TBI); Contra: contralateral brain hemisphere of animals with TBI. Values are mean ± SD, n = 6; *p < 0.05, **p < 0.01 and ***p < 0.001 versus PBS group; #p < 0.05, ##p < 0.01 and ###p < 0.001 versus PBS + NP + BDNF + PX group. Repeated measures two-way ANOVA with Bonferroni post-hoc analysis. ^p < 0.05, ^^p < 0.01 and ^^^p < 0.001 versus Sham group. Repeated measures one-way ANOVA with Bonferroni post-hoc analysis.
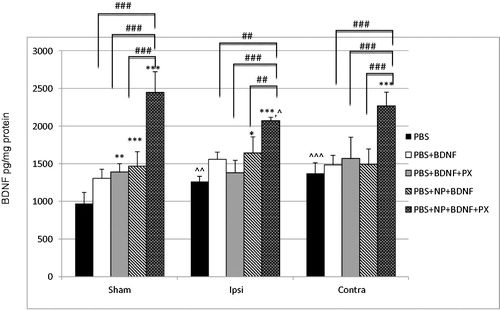
Sham-operated (healthy) mice
It can be seen that basic level of BDNF in the entire brain of healthy mice is 939.25 ± 148.89 BDNF pg/mg of total protein, this value is fully comparable with findings reported by other researches (Klein et al., Citation2011; Wu et al., Citation2014). Notably, IV injection of free BDNF in solution (group 2) caused a slight increase in BDNF levels, which was not significant compared to PBS alone. At the same time, after IV injection of the BDNF solution containing poloxamer 188 (group 3) as well as uncoated nanoparticle-bound BDNF (group 4) there were a significant increases in BDNF levels in comparison with PBS (p < 0.01 and p < 0.001, respectively). It is important to note that no significant differences in BDNF levels occurred in all cases between the three BDNF-containing groups 2, 3 and 4. In contrast, IV injection of nanoparticle-bound BDNF coated with poloxamer 188 (group 5) led to a substantial enhancement of the concentration of BDNF in sham-operated animals’ brain, resulting in 2.52-fold increase (p < 0.001). Moreover, this level was also significantly larger compared to groups 2–4 (p < 0.001).
TBI-injured mice versus sham-operated mice
Basic BDNF level in injured brains (group 1) significantly rose in both ipsi- and contralateral hemispheres compared with sham-operated to 130% (p < 0.01) and 141% (p < 0.001), respectively, indicating that TBI itself caused an increase in endogenous BDNF acute after injury. IV injection of free BDNF solution (group 2) and BDNF solution with poloxamer 188 (group 3) caused insignificant increase in BDNF levels compared to PBS alone in both injured hemispheres. Remarkably, injection of uncoated nanoparticulate BDNF (group 4) increased the concentration of BDNF in ipsilateral hemisphere of injured mice compared with PBS to 130% (p < 0.05), while in the contralateral hemisphere only statistically insignificant increase was observed. Similar to healthy mice, no significant differences in BDNF levels were observed between groups 2, 3 and 4. In addition, IV injection of nanoparticle-bound BDNF coated with poloxamer 188 (group 5) also led to a significant elevation of the concentration of BDNF in both ipsi- and contralateral hemispheres of animals’ brain with TBI, resulting in 1.64-fold and 1.65-fold increases (p < 0.001), respectively, compared with PBS group and significantly larger compared to groups 2–4 (p < 0.001).
Thereby, it is clearly seen that the overall pattern of BDNF concentration in injured mice brains was similar to the sham-operated mice. However, increase in brain BDNF levels in injured mice was not as substantial as the increase in the healthy mice and it was a statistical difference between groups 5 of sham-operated mice and ipsi-lateral part of the injured brain (p < 0.05).
Behavioral study
Neurological deficit
To evaluate functional neurological impairment after TBI, an established neurological severity score (NSS) system was used (). demonstrates changes in NSS for 1 week in C57Bl/6 male mice subjected to TBI. As shown in the figure, 1 h post-injury mice from group 1 (control) scored 7.17 ± 0.69 points, indicating severe injury. There were no significant differences in NSS compared to other groups at this time point, thus all mice were considered to have TBI with equal severity. It is important to note that mice with a score above 8 were withdrawn from the experiment to prevent extreme distress and high lethality rates as per recommendation of the protocol (Flierl et al., Citation2009). Further evaluation of the control group showed a gradual decrease in NSS during 7 days of observation with 4.33 ± 0.75 points at day 7 post-injury, indicating a spontaneous recovery phenomenon. A similar picture was observable in groups 2–5, and no difference was found at any single time point compared to the control group. Significant improvements of neurological deficit were noticed in the animals of group 6 at day 7 after injury. At 1.67 ± 0.47 points, they demonstrated significantly larger neurological outcomes compared to the control group (p < 0.001) as well as to groups 2–5 (p < 0.05).
Figure 3. A bar graphs depicting neurological severity score (NSS) in male C57Bl/6 mice with brain trauma at 1 h, 4 h, 1d, 2d, 3d and 7d post-injury. 3 h post-injury, intravenous injection in volume 0.2 ml was administered with the following solutions: phosphate-buffered saline (PBS); solution of recombinant BDNF in PBS (PBS + BDNF); solution of recombinant BDNF in PBS with poloxamer 188 (PBS + BDNF + PX); suspension of empty poly(lactic-co-glycolic acid) nanoparticles (NP); suspension of NP with adsorbed BDNF on their surface (PBS + NP + BDNF); suspension of NP with adsorbed BDNF on their surface and coated by poloxamer 188 (PBS + NP + BDNF + PX). Values are mean ± SD, n = 6; *p < 0.05, **p < 0.01 and ***p < 0.001 versus PBS + NP + BDNF + PX group. Repeated measures two-way ANOVA with Bonferroni post-hoc analysis.
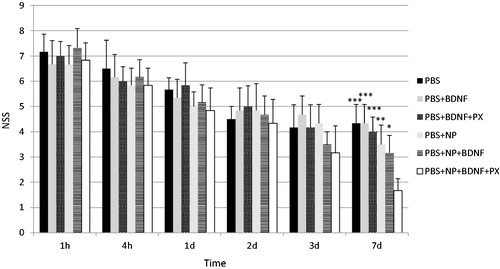
Cognitive deficits
The passive avoidance paradigm was used to evaluate cognitive impairments in mice after TBI. Our previous experimental findings have demonstrated that cognitive deficits after severe TBI could be detected by the PA test on day 7 after injury (Khalin et al., Citation2016). depicts TBI-induced cognitive impairments in C57Bl/6 male mice at day 7 post-injury expressed as a mean latent time (LT) that animals spent in the lighted chamber. A cutoff time of 180 s was chosen, therefore, this LT in sham-operated mice indicated no memory disorders. Animals with TBI treated with PBS (group 1) showed LT of 114.9 ± 29.2 s which was significantly lower than in animals without trauma, indicating a post-traumatic memory deficit. The same picture was observed in animals in groups 2–5 (). In contrast, the LT in mice with TBI injected by PBS + NP + BDNF + PX reached 170.3 ± 19.6 s, which was statistically higher than the control group as well as groups 2–5 and was not statistically different from the sham-operated mice. These data show that the BDNF-loaded nanoparticles coated with poloxamer 188 could reverse the cognitive deficits induced by TBI in mice.
Figure 4. Mean latent time (sec ± m, n = 6) that the mice spent at the light chamber, at day 7 after the brain injury (cutoff time 180 s). Group Sham includes mice with sham operation. The rest groups include mice subjected to TBI and received IV injection 3 h post-injury of following solutions: PBS: control group with PBS solution as drug vehicle; PBS + BNDF: solution of BDNF (5 μg/per mouse); PBS + BNDF + PX: 1% solution of Poloxamer®188 with BDNF (5 μg/per mouse); PBS + NP: solution of empty 2% PLGA NPs; PBS + NP + BNDF: solution of BDNF (5 μg/per mouse) adsorbed on PLGA NP; PBS + NP + BDNF + PX: solution of BDNF (5 μg/per mouse) adsorbed on PLGA NP coated with Poloxamer®188. *p < 0.05, **p < 0.01, ***p < 0.001 versus PBS + NP + BDNF + PX group; #p < 0.05, ##p < 0.01, ###p < 0.001 versus control (PBS) group repeated measures one-way ANOVA, with Bonferroni’s multiple comparison tests.
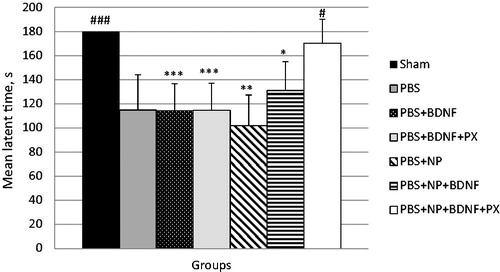
Discussion
This in vivo study for the first time demonstrates that IV injection of PLGA NPs with BDNF coated with poloxamer 188 leads to increased BDNF levels in the CNS and to an improvement in neurological and cognitive outcomes in animals with TBI.
In this study, we used the C57Bl/6 male mice and induced TBI. We demonstrated that at 4 h post-injury, the level of endogenous BNDF was significantly higher in comparison with sham-operated mice in both ipsi- (p < 0.01) and contralateral (p < 0.001) brain hemispheres. Working with rats with experimental TBI, Oyesiku et al. demonstrated an increase in endogenous BDNF expression in the injured as well as the non-injured regions of the brain after 12 h post-injury (Oyesiku et al., Citation1999). This increase was transient, and the concentration was restored to control levels at 36 h post-injury. Research by Wu et al. suggested a similar pattern, demonstrating that after 4 days post-injury the levels of BDNF in C57Bl/6 mice already had decreased in both, ipsi- and contralateral brain hemispheres (Wu et al., Citation2014). In contrast, Griesbach et al. reported increased levels of BDNF in the brains of young rats up to 14 days after TBI (Griesbach et al., Citation2002). Despite these controversies in duration, it has been consistently shown that an early but transient increase in BDNF expression is an endogenous neuroprotective compensatory response to brain trauma, which is in line with our study findings.
Thus, sustained increase in BDNF levels in the brain tissues could be beneficial for recovery from injury and hence the use of exogenous BDNF as a therapeutic agent has gained importance. However, Pardridge et al. demonstrated in rats that after IV injection, BDNF itself was unable to cross BBB in healthy animals and its plasma half-life was ∼2.7 min (Pardridge et al., Citation1994). In contrast, Pan et al. showed in mice the ability of BDNF to penetrate the BBB with a stable blood half-life up to 60 min (Pan et al., Citation1998). In this study, we have shown that 1 h (60 min) after IV administration of 5 μg BDNF reconstituted in PBS solution, the BDNF concentration in the mice’s brain was higher in both sham-operated and injured mice, but the difference between the two groups did not reach statistical significance. We did not measure the BDNF plasma concentration, therefore, it is difficult to say whether this increase was detected in the brain parenchyma, endothelial cells or in the blood. We presume that these data, likely, reflect the binding of BDNF to brain capillaries rather than penetration across the BBB, as BNDF has the ability to bind negatively charged surfaces of brain micro-vessels (Pardridge et al., Citation1994).
Despite the fact that BDNF level reached significance in some control groups containing BDNF adsorbed onto uncoated NPs or BDNF and PX in solution, the total amount of BNDF still was fairly low () and, probably, such an increase was due to a decreased rate of elimination of these compounds compared to free BNDF (Sakane & Pardridge, Citation1997). In contrast, 1 h post-injection of PLGA NPs with adsorbed BDNF coated with PX into healthy mice resulted in extremely elevated levels of BNDF in the brain, which was substantially higher compared to all other groups.
Targeted delivery of BDNF to the brain using polymeric nanoparticles or liposomes thus far has not been published anywhere. Pardridge et al., studied the delivery of BDNF conjugated to the OX26 vector exploiting the transferrin receptor-mediated transcytosis through the BBB (Pardridge et al., Citation1994). This study demonstrated that 60 min after IV injection [3H]-BDNF conjugated to that vector achieved nine-fold higher concentrations in the brain than non-conjugated BDNF. Unfortunately, it is impossible to compare our findings with that study as we cannot differentiate endogenous BNDF from injected growth factor.
It is well known that TBI leads to dysfunction of the BBB (Chodobski et al., Citation2011). By demonstrating that the increase in brain BDNF levels in injured mice was not as substantial as the increase in the sham-operated mice (), our results indicate that TBI interferes with the LDL receptor transport system at 4 h post injury. We don’t exclude that an increased permeability of the BBB could contribute to a higher penetration of BDNF into the brain; however, this phenomenon was shown to occur later than at 4 h (Başkaya et al., Citation1997).
It is clear that coating of PLGA NPs with PX is a crucial factor for the transport of BNDF into the brain. Chemically, PX is an amphiphilic triblock copolymer consisting of a central hydrophobic polyoxypropylene (POP) molecule, surrounded by two hydrophilic chains of polyoxyethylene (POE) (Moghimi & Hunter, Citation2000). When adsorbed onto PLGA NP, the POP chain anchors the molecule to the hydrophobic surface, while the PEO chains extend into the environs (Li et al., Citation1994) forming a conformational cloud on NP’s surface that reduce recognition by macrophages (Moghimi & Hunter, Citation2000), consequently enhancing the circulation time. As PX does not reduce the hepatic sequestration of the NPs to a large extent, the effect on circulation time is limited (Moghimi, Citation1997). However, when it comes to penetration across the BBB, PX is a more effective overcoating surfactant for PLGA particles than polysorbate 80 (Gelperina et al., Citation2010). Current understanding of the role of PX in penetration of PLGA NPs across the BBB was comprehensively described by Kreuter (Citation2014). Briefly, PLGA NPs coated with PX acquire the ability to adsorb onto their surface apolipoproteins E or A–I from the blood, which then interact with LDL or scavenger receptors of the brain capillary endothelial cells. The resulting carrier system mimics a lipoprotein, is transcytosed, and, acting as Trojan horse, appears to cross the BBB, delivering the cargo (Kreuter, Citation2014).
In this study, we found that IV injection of PLGA NPs with BDNF coated with PX achieved neuroprotection in mice with brain trauma. This was confirmed by significant improvements of neurological and cognitive deficit in mice with TBI on day 7 post-injury ( and ). The mechanism behind the neuroprotective properties of BDNF is related to the dimerization of the TrkB receptor, which then initiates three major signaling cascades. The first is the phospholipase Cγ pathway, which is associated with synaptic plasticity (Reichardt, Citation2006), the second is the phosphoinositide 3-kinase pathway, which is responsible for the survival and proliferation of neuronal cells (Zheng & Quirion, Citation2004) and, finally, the third is the mitogen-activated protein kinases pathway, which is crucial in neuronal cell growth, differentiation and protection (Numakawa et al., Citation2010). Our data are supported by numerous reviews devoted to neuroprotective properties of BDNF (Cowansage et al., Citation2010; Géral et al., Citation2013; Khalin et al., Citation2015) and consistent with many experimental studies. In particular, a plethora of in vitro studies have demonstrated the neuroprotective potential of BNDF (Kume et al., Citation1997; Zhang et al., Citation2003; Liu et al., Citation2007; Casalbore et al., Citation2010; Zhu et al., Citation2011), showing that BDNF is also able to provide anti-apoptotic, anti-inflammatory and anti-neurotoxic actions that are essential for secondary TBI treatment. In addition, BDNF itself contributes to cognitive functions improving long-term memory (Blurton-Jones et al., Citation2009) and was shown to improve working and reference memories in transient forebrain ischemia in rats (Kiprianova et al., Citation1999). However, to date only a handful of in vivo studies have been done with regards to the use of BDNF in TBI treatment. The main strategy of these studies was to increase the BDNF concentration in the CNS. A recent publication demonstrated that transplantation of neural stem cells genetically modified to encode BDNF resulted in an increase in BDNF mRNA levels in transplanted site of rat injured brain during two weeks. Importantly, this increase was accompanied by functional recovery of rats on day 7 after TBI (Ma et al., Citation2012). Another more recent study explored bone marrow stromal cells transfected with BDNF and injected intracerebroventricularly (ICV) into the rats with TBI (Wang et al., Citation2013), the authors found an increase in BDNF concentration using ELISA in cerebrospinal fluid from 12 h after injection during 90 days, leading to early improvements in neurologic deficit proven by significant decrease of NSS test detected at day 3 after TBI. These data corroborate our results as we also showed an increase in BDNF concentration and early functional recovery. It has to be noted that Blaha et al. found no significant effects on behavioral and cognitive outcomes after BDNF infusion directly into the cortex or hippocampus of rats with TBI using osmotic mini pump (Blaha et al., Citation2000). Probably in that study, the method of administration is a critical factor as BDNF does not diffuse well through brain parenchyma (Anderson et al., Citation1995). ICV infusion into ventricles would not be effective as well, because BDNF was shown to diffuse only 0.2 mm of brain from the ventricle following the ICV administration (Yan et al., Citation1994). Moreover, in contrast to the local administration using an osmotic pump, the employment of poloxamer188-coated nanoparticles allows the delivery to all parts of the brain as the responsible transcytosis appears to occur throughout the entire brain endothelium (Zensi et al., Citation2009). Therefore, use of molecular “Trojan horse” technology is considered to be a major approach in delivery of large molecules to the brain (Pardridge, Citation2016). In addition, the neuroprotective benefit from the activation of the TrkB receptor pathways was also demonstrated by Wu et al. using a BDNF-mimetic, also known as 7,8-dihydroxyflavone, in the treatment of C57Bl/6 mice with TBI (Wu et al., Citation2014). Indirectly this comprehensive study supports our data, showing an early neurological recovery (as assessed by NSS, beam walk traversing time and rotarod time) and an increased brain BDNF concentration.
Our study was limited to only one time point at 60 min as it was considered to be a peak of the maximal concentration (Kreuter, Citation2014) despite the presence of some contradictory data in other related studies. For example, Schroeder at al., demonstrated that 3H-labeled dalargin adsorbed onto PBCA NPs coated by another surfactant, i.e. polysorbate 80 after IV injection to the mice was 2-3-fold higher in brain homogenates compared to free 3H-labeled dalargin or adsorbed onto uncoated NPs, with a peak at 20 min after injection (Schroeder et al., Citation2000). However, other studies indirectly showed that maximal concentrations of dalargin appeared later: for instance, Kreuter et al., found maximal analgesic effects of dalargin adsorbed onto polysorbate-coated PBCA was 45 min after injection (Kreuter et al., Citation1995), whereas Das and Lin demonstrated the same effects at 60 min after injection. More relevant to this study was work done by Kurankhmaeva et al. where IV injection of another neurotrophin, namely NGF adsorbed onto PBCA NPs coated with P80, resulted in a substantial increase in NGF concentration in the C57Bl/6 mice brain, reaching a peak at 45 min after injection (Kurakhmaeva et al., Citation2009). The present findings coincide with that study and, although our time point was at 60 min, the levels of growth factors in the brain still are comparable.
The time frame of drug administration to patients with TBI is crucial, ideally the “therapeutic window” should coincide with clinical relevance (Chodobski et al., Citation2011). Our decision to employ a 3 h-delayed administration of the compound was based on two important factors: the first is associated with a real opportunity to reach the patient with unpredicted TBI; the second factor accounts to the particulars of acute reactive axonal swelling due to TAI, which is accompanied by an increased calcium influx that in turn contributes to the collapse of the axolemma and further swelling and axonal disconnection (Smith et al., Citation2013). BDNF itself is known to elevate intracellular calcium levels (Berninger et al., Citation1993) which in turn may worsen local calcium dysregulation thereby providing a progression towards disconnection of axons rather than neuroprotection. Blaha et al. suggested a 4-h delay after TBI to be optimal (Blaha et al., Citation2000). We performed the injection 3 h after injury allowing one more hour for the concentration in the brain to reach its maximum. The resulting 4-h delay attempted to minimize BDNF interference with acute axonal reactions.
Conclusions
Our study demonstrates the potential of using nanoparticulate delivery of BDNF into CNS for the treatment of TBI. We employed clinically relevant modeling of TBI, an optimal time point of drug administration, and a clinically feasible method of drug delivery. We believe these to be critically important considerations for a successful translation of the results into clinical practice in patients with TBI. However, many avenues for future investigations remain open. For example, it is important to assess the long-term action of nanoparticulate BDNF. Single injection of BDNF can probably produce only a transient effect, similar to that demonstrated in the neuroprotection of retinal ganglion cells in rats with axotomy (Mey & Thanos, Citation1993). Further, it is crucial to investigate morphological changes and biological markers of TBI and how our delivery system may influence them. Finally, it will be important to evaluate the rate of activation of TrkB pathways. Nevertheless, this study is the first to show that PLGA nanoparticles coated with poloxamer 188 can successfully deliver large molecule BDNF into the brain, subsequently resulting in the improvement of neurological and cognitive deficits in TBI mice, thereby providing a neuroprotective effect.
Declaration of interest
The authors report no conflicts of interest in this work. The study was supported by a grant from the Ministry of Higher Education of Malaysia (RAGS/2013/UPNM/SKK/01/2).
References
- Allen SJ, Watson JJ, Shoemark DK, et al. (2013). GDNF, NGF and BDNF as therapeutic options for neurodegeneration. Pharmacol Ther 138:155–75
- Alyautdin R, Kreuter J. (2000). Using nanoparticles to target drugs to the central nervous system. In: Kreuter J, Begley DJ, Bradbury MW, eds. The blood-brain barrier and drug delivery to the CNS. USA: CRC Press
- Alyautdin RN, Petrov VE, Langer K, et al. (1997). Delivery of loperamide across the blood-brain barrier with polysorbate 80-coated polybutylcyanoacrylate nanoparticles. Pharm Res 14:325–8
- Alyautdin RN, Tezikov EB, Ramge P, et al. (1998). Significant entry of tubocurarine into the brain of rats by adsorption to polysorbate 80-coated polybutylcyanoacrylate nanoparticles: an in situ brain perfusion study. J Microencapsul 15:67–74
- Anderson KD, Alderson RF, Altar CA, et al. (1995). Differential distribution of exogenous BDNF, NGF, and NT‐3 in the brain corresponds to the relative abundance and distribution of high‐affinity and low‐affinity neurotrophin receptors. J Comp Neurol 357:296–317
- Başkaya MK, Rao AM, Doğan A, et al. (1997). The biphasic opening of the blood–brain barrier in the cortex and hippocampus after traumatic brain injury in rats. Neurosci Lett 226:33–6
- Berninger B, García DE, Inagaki N, et al. (1993). BDNF and NT-3 induce intracellular Ca2+ elevation in hippocampal neurones. Neuroreport 4:1303–6
- Blaha GR, Raghupathi R, Saatman KE, McIntosh TK. (2000). Brain-derived neurotrophic factor administration after traumatic brain injury in the rat does not protect against behavioral or histological deficits. Neuroscience 99:483–93
- Blurton-Jones M, Kitazawa M, Martinez-Coria H, et al. (2009). Neural stem cells improve cognition via BDNF in a transgenic model of Alzheimer disease. Proc Natl Acad Sci U S A 106:13594–9
- Casalbore P, Barone I, Felsani A, et al. (2010). Neural stem cells modified to express BDNF antagonize trimethyltin-induced neurotoxicity through PI3K/Akt and MAP kinase pathways. J Cell Physiol 224:710–21
- Chodobski A, Zink BJ, Szmydynger-Chodobska J. (2011). Blood-brain barrier pathophysiology in traumatic brain injury. Transl Stroke Res 2:492–516
- Cowansage KK, Ledoux JE, Monfils MH. (2010). Brain-derived neurotrophic factor: a dynamic gatekeeper of neural plasticity. Curr Mol Pharmacol 3:12–29
- Flierl MA, Stahel PF, Beauchamp KM, et al. (2009). Mouse closed head injury model induced by a weight-drop device. Nat Protoc 4:1328–37
- Gelperina S, Maksimenko O, Khalansky A, et al. (2010). Drug delivery to the brain using surfactant-coated poly(lactide-co-glycolide) nanoparticles: influence of the formulation parameters. Eur J Pharm Biopharm 74:157–63
- Géral C, Angelova A, Lesieur S. (2013). From molecular to nanotechnology strategies for delivery of neurotrophins: emphasis on brain-derived neurotrophic factor (BDNF). Pharmaceutics 5:127–67
- Grafman J, Salazar AM. 2015. Traumatic brain injury, Part I: handbook of clinical neurology (Series editors: Aminoff, Boller and Swaab). Amsterdam: Elsevier
- Graham Di GT, McIntosh TK. 2002. Trauma. In: Graham Di LP, ed. Greenfield's neuropathology. London: Arnold
- Grazu VMM. 2012. Nanocarriers as nanomedicines: design concepts and recent advances. In: De La Fuente JGV, ed. Nanobiotechnology inorganic nanoparticles vs organic nanoparticle. Amsterdam: Elsevier
- Griesbach GS, Hovda DA, Molteni R, Gomez-Pinilla F. (2002). Alterations in BDNF and synapsin I within the occipital cortex and hippocampus after mild traumatic brain injury in the developing rat: reflections of injury-induced neuroplasticity. J Neurotrauma 19:803–14
- Group BS. (1999). A controlled trial of recombinant methionyl human BDNF in ALS: the BDNF Study Group (Phase III). Neurology 52:1427–33
- Guidelines. (2007). Guidelines for the management of severe traumatic brain injury. J Neurotrauma 24:S1–106
- Hicks RR, Zhang L, Dhillon HS, et al. (1998). Expression of trkB mRNA is altered in rat hippocampus after experimental brain trauma. Brain Res Mol Brain Res 59:264–8
- Kabadi SV, Faden AI. (2014). Neuroprotective strategies for traumatic brain injury: improving clinical translation. Int J Mol Sci 15:1216–36
- Khalin I, Alyautdin R, Kocherga G, Bakar MA. (2015). Targeted delivery of brain-derived neurotrophic factor for the treatment of blindness and deafness. Int J Nanomedicine 10:3245–67
- Khalin I, Jamari NLA, Razak NBA, et al. (2016). A mouse model of weight-drop closed head injury: emphasis on cognitive and neurological deficiency. Neural Regen Res 11:630–5
- Kiprianova I, Sandkuhler J, Schwab S, et al. (1999). Brain-derived neurotrophic factor improves long-term potentiation and cognitive functions after transient forebrain ischemia in the rat. Exp Neurol 159:511–19
- Klein AB, Williamson R, Santini MA, et al. (2011). Blood BDNF concentrations reflect brain-tissue BDNF levels across species. Int J Neuropsychopharmacol 14:347–53
- Kreuter J. (2014). Drug delivery to the central nervous system by polymeric nanoparticles: what do we know? Adv Drug Deliv Rev 71:2–14
- Kreuter J, Alyautdin RN, Kharkevich DA, Ivanov AA. (1995). Passage of peptides through the blood-brain barrier with colloidal polymer particles (nanoparticles). Brain Res 674:171–4
- Kume T, Kouchiyama H, Kaneko S, et al. (1997). BDNF prevents NO mediated glutamate cytotoxicity in cultured cortical neurons. Brain Res 756:200–4
- Kurakhmaeva KB, Djindjikhashvili IA, Petrov VE, et al. (2009). Brain targeting of nerve growth factor using poly(butyl cyanoacrylate) nanoparticles. J Drug Target 17:564–74
- Li JT, Caldwell KD, Rapoport N. (1994). Surface properties of pluronic-coated polymeric colloids. Langmuir 10:4475–82
- Liu Z, Ma D, Feng G, et al. (2007). Recombinant AAV-mediated expression of human BDNF protects neurons against cell apoptosis in Abeta-induced neuronal damage model. J Huazhong Univ Sci Technolog Med Sci 27:233–6
- Lu JM, Wang X, Marin-Muller C, et al. (2009). Current advances in research and clinical applications of PLGA-based nanotechnology. Expert Rev Mol Diagn 9:325–41
- Ma H, Yu B, Kong L, et al. (2012). Neural stem cells over-expressing brain-derived neurotrophic factor (BDNF) stimulate synaptic protein expression and promote functional recovery following transplantation in rat model of traumatic brain injury. Neurochem Res 37:69–83
- Mahmood A, Lu D, Wang L, Chopp M. (2002). Intracerebral transplantation of marrow stromal cells cultured with neurotrophic factors promotes functional recovery in adult rats subjected to traumatic brain injury. J Neurotrauma 19:1609–17
- Mey J, Thanos S. (1993). Intravitreal injections of neurotrophic factors support the survival of axotomized retinal ganglion cells in adult rats in vivo. Brain Res 602:304–17
- Michaelis K, Hoffmann MM, Dreis S, et al. (2006). Covalent linkage of apolipoprotein e to albumin nanoparticles strongly enhances drug transport into the brain. J Pharmacol Exp Ther 317:1246–53
- Moghimi SM. (1997). Prolonging the circulation time and modifying the body distribution of intravenously injected polystyrene nanospheres by prior intravenous administration of poloxamine-908. A 'hepatic-blockade' event or manipulation of nanosphere surface in vivo? Biochim Biophys Acta 1336:1–6
- Moghimi SM, Hunter AC. (2000). Poloxamers and poloxamines in nanoparticle engineering and experimental medicine. Trends Biotechnol 18:412–20
- Numakawa T, Suzuki S, Kumamaru E, et al. (2010). BDNF function and intracellular signaling in neurons. Histol Histopathol 25:237–58
- Oyesiku NM, Evans CO, Houston S, et al. (1999). Regional changes in the expression of neurotrophic factors and their receptors following acute traumatic brain injury in the adult rat brain. Brain Res 833:161–72
- Pan W, Banks WA, Fasold MB, et al. (1998). Transport of brain-derived neurotrophic factor across the blood-brain barrier. Neuropharmacology 37:1553–61
- Pardridge WM. (2016). CSF, blood-brain barrier, and brain drug delivery. Expert Opin Drug Deliv. [Epub ahead of print]. DOI: 10.1517/17425247.2016.1171315
- Pardridge WM, Kang YS, Buciak JL. (1994). Transport of human recombinant brain-derived neurotrophic factor (BDNF) through the rat blood-brain barrier in vivo using vector-mediated peptide drug delivery. Pharm Res 11:738–46
- Petri B, Bootz A, Khalansky A, et al. (2007). Chemotherapy of brain tumour using doxorubicin bound to surfactant-coated poly(butyl cyanoacrylate) nanoparticles: revisiting the role of surfactants. J Control Release 117:51–8
- Poduslo JF, Curran GL. (1996). Permeability at the blood-brain and blood-nerve barriers of the neurotrophic factors: NGF, CNTF, NT-3, BDNF. Brain Res Mol Brain Res 36:280–6
- Quertemont E, Tambour S, Bernaerts P, et al. (2004). Behavioral characterization of acetaldehyde in C57BL/6J mice: locomotor, hypnotic, anxiolytic and amnesic effects. Psychopharmacology (Berl) 177:84–92
- Reichardt LF. (2006). Neurotrophin-regulated signalling pathways. Philos Trans R Soc Lond B Biol Sci 361:1545–64
- Sakane T, Pardridge WM. (1997). Carboxyl-directed pegylation of brain-derived neurotrophic factor markedly reduces systemic clearance with minimal loss of biologic activity. Pharm Res 14:1085–91
- Schroeder U, Schroeder H, Sabel BA. (2000). Body distribution of 3H-labelled dalargin bound to poly(butyl cyanoacrylate) nanoparticles after i.v. injections to mice. Life Sci 66:495–502
- Sharma A, Madhunapantula SV, Robertson GP. (2012). Toxicological considerations when creating nanoparticle-based drugs and drug delivery systems. Expert Opin Drug Metab Toxicol 8:47–69
- Singh M, Sumien N, Kyser C, Simpkins JW. (2008). Estrogens and progesterone as neuroprotectants: what animal models teach us. Front Biosci 13:1083–9
- Smith DH, Hicks R, Povlishock JT. (2013). Therapy development for diffuse axonal injury. J Neurotrauma 30:307–23
- Steiniger SC, Kreuter J, Khalansky AS, et al. (2004). Chemotherapy of glioblastoma in rats using doxorubicin-loaded nanoparticles. Int J Cancer 109:759–67
- Tosi G, Vergoni AV, Ruozi B, et al. (2010). Sialic acid and glycopeptides conjugated PLGA nanoparticles for central nervous system targeting: in vivo pharmacological evidence and biodistribution. J Control Release 145:49–57
- Wagner S, Zensi A, Wien SL, et al. (2012). Uptake mechanism of ApoE-modified nanoparticles on brain capillary endothelial cells as a blood-brain barrier model. PLoS One 7:e32568
- Wallace SJ, Li J, Nation RL, Boyd BJ. (2012). Drug release from nanomedicines: selection of appropriate encapsulation and release methodology. Drug Deliv Transl Res 2:284–92
- Wang Z, Yao W, Deng Q, et al. (2013). Protective effects of BDNF overexpression bone marrow stromal cell transplantation in rat models of traumatic brain injury. J Mol Neurosci 49:409–16
- Wohlfart S, Gelperina S, Kreuter J. (2012). Transport of drugs across the blood-brain barrier by nanoparticles. J Control Release 161:264–73
- Wu CH, Hung TH, Chen CC, et al. (2014). Post-injury treatment with 7,8-dihydroxyflavone, a TrkB receptor agonist, protects against experimental traumatic brain injury via PI3K/Akt signaling. PLoS One 9:e113397
- Xiong Y, Mahmood A, Chopp M. (2013). Animal models of traumatic brain injury. Nat Rev Neurosci 14:128–42
- Yan Q, Matheson C, Sun J, et al. (1994). Distribution of intracerebral ventricularly administered neurotrophins in rat brain and its correlation with trk receptor expression. Exp Neurol 127:23–36
- Zensi A, Begley D, Pontikis C, et al. (2009). Albumin nanoparticles targeted with Apo E enter the CNS by transcytosis and are delivered to neurones. J Control Release 137:78–86
- Zhang J, Geula C, Lu C, et al. (2003). Neurotrophins regulate proliferation and survival of two microglial cell lines in vitro. Exp Neurol 183:469–81
- Zheng WH, Quirion R. (2004). Comparative signaling pathways of insulin-like growth factor-1 and brain-derived neurotrophic factor in hippocampal neurons and the role of the PI3 kinase pathway in cell survival. J Neurochem 89:844–52
- Zhu JM, Zhao YY, Chen SD, et al. (2011). Functional recovery after transplantation of neural stem cells modified by brain-derived neurotrophic factor in rats with cerebral ischaemia. J Int Med Res 39:488–98