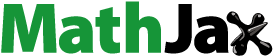
Abstract
Focused Ultrasound (FUS)-triggered nano-sized drug delivery, as a smart stimuli-responsive system for treating solid tumors, is computationally investigated to enhance localized delivery of drug and treatment efficacy. Integration of thermosensitive liposome (TSL), as a doxorubicin (DOX)-loaded nanocarrier, and FUS, provides a promising drug delivery system. A fully coupled partial differential system of equations, including the Helmholtz equation for FUS propagation, bio-heat transfer, interstitial fluid flow, drug transport in tissue and cellular spaces, and a pharmacodynamic model is first presented for this treatment approach. Equations are then solved by finite element methods to calculate intracellular drug concentration and treatment efficacy. The main objective of this study is to present a multi-physics and multi-scale model to simulate drug release, transport, and delivery to solid tumors, followed by an analysis of how FUS exposure time and drug release rate affect these processes. Our findings not only show the capability of model to replicate this therapeutic approach, but also confirm the benefits of this treatment with an improvement of drug aggregation in tumor and reduction of drug delivery in healthy tissue. For instance, the survival fraction of tumor cells after this treatment dropped to 62.4%, because of a large amount of delivered drugs to cancer cells. Next, a combination of three release rates (ultrafast, fast, and slow) and FUS exposure times (10, 30, and 60 min) was examined. Area under curve (AUC) results show that the combination of 30 min FUS exposure and rapid drug release leads to a practical and effective therapeutic response.
1. Introduction
The efficacy of conventional chemotherapy, as a mainstay of cancer treatment, is restricted by insufficient delivery of anti-cancer drugs to cancer cells and by toxicity to healthy tissue that limits the administered dosage. The former is primarily attributed to poor drug penetration from microvessels into the tumor interior arising from physiological obstacles of the tumor microenvironment (TME) and short circulation time (Jain, Citation1987). On the other hand, unfavorable deposition of drugs in healthy tissue can damage normal cells, causing a range of adverse effects. Different nano-sized drug delivery systems (DDSs) including liposomes, micelles, solid nanoparticles, and macromolecular drug carriers have been developed to overcome these restrictions. Stimuli-responsive nano-sized DDSs have recently illustrated great promise for cancer treatment to improve the drug bioavailability locally by providing controlled, timed, and destination-specific drug release (Mura et al., Citation2013; Karimi et al., Citation2016; Kashkooli et al., Citation2020; Mi, Citation2020; Kashkooli et al., Citation2021; Souri et al., Citation2022). The stimulus that facilitates drug release can be internal (such as pH, enzyme, hypoxia), external (such as magnetic, ultrasonic, light), or a combination of these (Gao et al., Citation2010; Karimi et al., Citation2016; Kashkooli et al., Citation2020; Citation2021; Van Durme et al., Citation2021). Most of these therapeutic agents are passive and aggregate in tumors due to the unique pathophysiology of the TME, including the large size of vessel wall pores that results in the enhanced permeability and retention (EPR) effect (Kashkooli et al., Citation2021; Moradi Kashkooli et al., Citation2021). The EPR effect is highly heterogeneous in different tumors. In addition, large drug carriers have a poor transvascular rate; hence, there is growing consensus that alternative methods are needed to improve targeting (Danhier, Citation2016; Ten Hagen et al., Citation2021). One proposed method is stimuli-responsive intravascular release (Ten Hagen et al., Citation2021), in which the encapsulated drug is released within the vessels and readily extravasated into the tumor. To achieve a high therapeutic response, the intravascular release paradigm should meet a basic principle: drug release at a high rate (Seynhaeve et al., Citation2020; Souri et al., Citation2022). External stimuli perform better for targeted rapid release within microvessels (Ten Hagen et al., Citation2021).
Among different external stimuli for activating drugs from drug-loaded NPs, ultrasound offers promising therapeutic results in both preclinical (Schultz et al., Citation2021) and clinical (Dimcevski et al., Citation2016) settings, by increasing localized uptake of therapeutic agents in solid tumors. Apart from common diagnostic uses, ultrasound has also been widely utilized in cancer treatments, including both destructive (i.e. thermal ablation) (Ter Haar, Citation2016) and nondestructive (i.e. mild hyperthermia) (Sirsi & Borden, Citation2014; Boissenot et al., Citation2016; Kashkooli et al., Citation2023) applications. Generally, FUS is capable of reaching deeper tissues while having minimal impact on the tissue between the surface of transducer and the focus area (Mihcin & Melzer, Citation2018). However, the heterogeneity of biological tissue can enhance complexity in ultrasound targeting of tumor areas (Zhang et al., Citation2022). Ultrasound-activated nano-sized DDSs have recently gained interest because they enable localized delivery of drugs to specific locations such as tumors (Couture et al., Citation2014; Ahmadi et al., Citation2020). Combining ultrasound with nano-sized DDSs can resolve some of the limitations of conventional nano-sized DDSs, including poor penetration into tumor tissue, the limited amount of drug released from NPs (Du et al., Citation2011), and loss of targeting ability. Ultrasound-nanocarrier interactions which lead to drug release are generally categorized as either thermal or mechanical interactions (Kashkooli et al., Citation2023). For any given application, a nanocarrier-drug compound can be engineered to react to a thermal excitation, mechanical stimulation, or a mix of the two to trigger drug release (Tharkar et al., Citation2019; Souri et al., Citation2022; Hornsby et al., Citation2023; Kashkooli et al., Citation2023). Therefore, ultrasound-induced DDSs can be designed to allow localized drug delivery into tumors while preserving normal tissues and organs at risk (Souri et al., Citation2022).
To enable ultrasound-activated release of drug, DDSs can be engineered with drug-loaded NPs that respond to ultrasonic waves (Hornsby et al., Citation2023). In the case of FUS-induced thermal fields, a mild temperature rise below the threshold of inducing any thermal tissue damage (<45 °C) can stimulate drug release from TSLs. This is the most well-known temprature-controlled drug-containing NPs reported in the literature (Kneidl et al., Citation2014), leading to enhanced drug and synergism with FUS in killing tumor cells (Papaioannou & Avgoustakis, Citation2022). Consequently, localized heating can be obtained through FUS, which is preferred in clinical thermal treatment due to its noninvasive nature, precision, and reliability (Hynynen, Citation2011). The safety and possibility of such a FUS-TSL hybrid delivery system were confirmed in recently published clinical human trials for treating liver tumors (Lyon et al., Citation2017; Citation2018; Citation2019). However, there is still an incomplete understanding of the mechanism of drug transport and drug uptake by tumor cells in response to FUS-induced temperature changes. In addition, heat absorption strongly depends on the thermophysical characteristics of the targeted tissue and the exposure parameters of the ultrasound beam (Izadifar et al., Citation2017). Hence, optimal treatment parameters should be chosen to increase the procedure’s efficacy, including providing the required temperature level, reducing the treatment duration, and inhibiting adverse effects on adjacent cells (Roohi et al., Citation2021).
Due to several stages included in the heat exchange and drug delivery process and the complex interactions of the ultrasound with tissue, the nanocarrier, and drugs, computational modeling plays a significant role in gaining in-depth insights into the delivery system and prediction of their therapeutic effectiveness. Early computational models in this field were developed to investigate interstitial fluid flow and macromolecule transport in solid tumors (Baxter & Jain, Citation1989; Citation1990; Citation1991). Subsequently, they expanded to several scales—including cellular and tissue—to examine the influences of various factors on drug transport (Wang et al., Citation1999; El-Kareh & Secomb, Citation2000; Goh et al., Citation2001; Eikenberry, Citation2009; Stylianopoulos et al., Citation2015; Zhan & Wang, Citation2018; Kashkooli et al., Citation2020; Löke et al., Citation2021; Moradi Kashkooli & Soltani, Citation2021; Soltani et al., Citation2021). Results of a one-dimensional drug delivery model showed that blood temperature had a significant influence on drug delivery outcome utilizing TSL (El-Kareh & Secomb, Citation2003). TSL performance was further analyzed compared with conventional chemotherapy and stealth liposomes under various circumstances (Gasselhuber et al., Citation2012), while a systemic parameter study was carried out to distinguish the most effective factors for determining maximum intracellular concentration by the TSL-high intensity focused ultrasound (HIFU) system (Liu & Xu, Citation2015). Gasselhuber et al. (Gasselhuber et al., Citation2012) combined the bio-heat transfer equation (BHTE) and a macroscopic drug transport model to predict the delivery of TSL and chemotherapeutic drugs under various heating regimens. To the best of our knowledge, no comprehensive mathematical modeling is presented in the literature that couples the physics of ultrasound and ultrasound-induced drug release kinetics with drug transport and delivery, especially for intravascular drug release. In addition, the temperature dependence of parameters associated with tumor tissue and therapeutic agents (TSL and chemotherapeutic agents) has not been rigorously investigated. More recently, a few groups attempted to address some of these issues for specific applications (Rezaeian et al., Citation2019; Zhan et al., Citation2019; Kim et al., Citation2021; Namakshenas & Mojra, Citation2023), but they do not present a comprehensive computational model that incorporates different physics, scales, and various details on the heterogeneous geometry of the tumor (e.g. microvasculature, necrotic core, hypoxic regions) in order to predict and optimize this DDS.
The main goal of the current study is to present a multi-scale and multi-physics computational model for FUS-triggered nano-sized DDS delivery in solid tumors. The present study proposes a model that predicts treatment responses by considering a wide range of biological interactions with drug agents in the intravascular release of DOX from TSL. Based on an extensive literature review and our best knowledge, this is one of the-first-of-its-kind studies to computationally examine FUS-triggered nano-sized DDS by integrating acoustic, heat transfer, intravascular drug release, interstitial fluid flow, mass transport, and bio-chemical activity of drug based on pharmacodynamics equations to examine treatment efficacy and AUC. For this purpose, we first examine the model’s validity in predicting the acoustic and thermal fields, which are the primary influencing factors on the TSL drug release. Subsequently, TSL/drug transport simulations are carried out in vascular, interstitial, and cellular spaces. Temperature-induced changes in tissue, TSL, and drug transport characteristics are also taken into account in the model. To quantify the effectiveness of treatment, pharmacodynamic parameters (i.e. the fraction of survival cancerous cells and the AUC) are calculated using intracellular and extracellular drug concentrations, respectively. In the following, two significant factors in FUS-induced nano-sized DDSs, drug release rate from TSLs and exposure time of FUS, are selected to examine their impacts on the AUC.
2. Materials and methods
2.1. Drug delivery mechanism
Schematics of the DDS and different interactions of TSL/drug agents, along with a multi-compartment model of the current study, are shown in . Integration of TSL and FUS provides a DDS via drug release at the tumor site, where TSLs are administrated intravenously and reach the tumor site via the circulatory system. TSLs enter the tumor microvessels where thermally triggered drug release is initiated due to temperature increase in the sonicated region inside the tumor due to acoustic energy absorption. The released drug extravasates rapidly into the tumor tissue and is then taken up by tumor cells. Therapeutic drug molecules have the potential to bind to plasma proteins in the blood, preventing drug agents from targeting cancer cells (Greene et al., Citation1983). The assumption is that free drug molecules can enter the cellular space; on the other hand, free drug molecules can be pumped out of cells based on multidrug-resistant traits of cells (El-Kareh & Secomb, Citation2000; Chabner & Longo, Citation2011). The present mathematical model has been developed according to a multi-compartment model in which each bio-physical medium is considered a compartment, and therapeutic agents are exchanged between these compartments.
Figure 1. (a) Schematic of DDS and different interactions of TSL/drug agents. T, Tm, kr, S, P, Pb, and Pu Stand for temperature, melting temperature of TSLs, the release rate of the drug in response to the FUS-induced temperature rise, transport of drug agents between systemic circulation and microvessels, transport of drug agents between microvessels and interstitium, association/dissociation of drug molecules to protein, exchange of drug molecules between interstitium and cellular space, (b) multi-compartment model of the current study. T: TSL, F: free DOX, B: Protein-bound DOX, L: DOX removed by the lymphatic drainage system, E: Elimination in the microvascular network, S: Exchange between circulatory system and microvessels, P: Exchange between microvascular network and interstitium, I: Exchange between interstitium and intracellular, O: Exchange between systemic circulation and other tissue and organs, : Release rate of drug at 37 °C,
: Release rate of drug at temperature T,
: Association/dissociation rate of drug molecules to protein.

2.2. Mathematical modeling and governing equations
A detailed description of mathematical models, different parameters used in the models, and their references are listed in . First, the FUS-induced thermal field in the tissue and its microvessels is simulated. The acoustic wave propagation equation and BHTE are solved to simulate acoustic wave and thermal fields in the computational domain, respectively. Subsequently, a set of partial differential equations governing the TSL and DOX concentration distribution are solved, including the equations governing fluid flow in the interstitium and mass transport in systemic plasma and tissue. Darcy’s law, which describes the relationship between interstitial fluid velocity (IFV) and interstitial fluid pressure (IFP), is considered to define interstitial fluid flow in tissue as a porous medium. TSL transport and drug concentration in tissue can be determined through the convection-diffusion-reaction (CDR) equation. Upon entering the tissue interstitium, the free drug is taken up by cells through a passive mechanism driven by the drug concentration gradient. Intracellular drugs can be pumped out of cells, most probably through membrane transporter proteins involved in multidrug resistance (e.g. P-glycoprotein). Additionally, the free drugs binding to interstitial proteins (such as albumin) is also taken into account in the equations. Eventually, two pharmacodynamic models, which are dependent on internalized and extracellular concentrations, are used to calculate the cancer cells’ survival fraction and AUC, respectively.
Table 1. Different equations used in each step for modeling US-mediated nano-sized DDSs alongside their references.
2.3. Parameter values
Since the tumor and healthy tissue growth are negligible during drug delivery, all the geometric and drug transport parameters utilized in the present study are considered time-independent. Values adopted for different parameters and physics are summarized in Tables S1–S4. Physiological parameters, parameters for DOX, and parameters for the TSLs are listed in Tables S1–S3, respectively. In addition, since the temperature increase induced by ultrasound could affect some of the physical characteristics utilized in the drug delivery model, the temperature dependence of perfusion rate, thermal conductivity, specific heat capacity, density, vascular permeability, viscosity, diffusion coefficient, transmembrane rate are also considered (). Subsequently, acoustic parameters of the domain are presented in Table S4.
Table 2. Temperature dependency of some properties of tissue and drug alongside their references.
Table 3. Release rates of drug from TSLs at different temperatures (some data have been interpolated).
2.4. Model geometry and boundary conditions
A schematic of the computational domain used in this study, including the tumor, surrounding normal tissue, and transducer along with boundary conditions (BCs) are presented in . A spherically focused 2.0 MHz single-element transducer, with 64.0 mm aperture diameter and 63.2 mm radius of curvature is selected in this simulation study. This transducer is located at the bottom side of the computational domain. As a baseline geometry, a spherical tumor with a radius of 12 mm is positioned in the center of a 2D block of normal tissue (72 × 45 mm2). The domain is surrounded by a 5-mm perfectly matched layer (PML) that uses artificial absorption to prevent waves from reflecting into the domain. In addition, BCs for acoustic, thermal and concentration simulations are shown in . The COMSOL software defines the BCs between the tumor and healthy tissue while the user determines the other BCs.
2.5. Assumptions
Key assumptions considered in the simulation study are listed below:
In this study, all simulations have been done using a two-dimensional axisymmetric geometry to reduce computational costs.
The tumor is assumed to be well-vascularized with a uniform distribution of microvessels in the computational domain. Therefore, the tumors are not hypoxic. Despite this simplification, this is a widespread assumption in modeling drug delivery to solid tumors in the literature (Kashkooli et al., Citation2020; Soltani et al., Citation2021; Souri et al., Citation2021).
Since ultrasound is not pulsed, we employ a frequency domain solution, which determines the steady-state acoustic field according to the operating circumstances and BCs. It is worth mentioning that non-linear effects and shear waves induced by ultrasound are ignored in the present study.
There is always the possibility of sonoporation with or without ultrasound contrast agents. However, we have focused on the thermal effects caused by ultrasound in this study, and any mechanical effects (including sonoporation) have not been considered.
Simulation time for ultrasound-mediated drug delivery is much shorter than the time scale for tumor growth; therefore, geometry and physiological variables are considered time-independent.
In intravenous administration, it is considered that a perfect mixing within the systemic plasma happens, meaning that the entire body is exposed to the drug post-administration (Baxter & Jain, Citation1989; Gasselhuber et al., Citation2012; Souri et al., Citation2021). Therefore, the body is considered to be subjected to the administered drug concentration at time zero.
DOX-loaded TSL is considered to mix perfectly and homogenously inside the plasma during administration.
Only free DOX drug molecules can enter the cellular space and induce DNA damage and kill cancer cells. Efflux pumps wash out inactive drug molecules within the cellular space (Ughachukwu et al., Citation2012).
2.6. Numerical mesh size study
To correctly simulate the results, it is necessary to check the independence of the results from the mesh. The acoustic pressure and acoustic intensity are selected for the mesh analysis. Triangular elements discretize the entire domain. It is worth mentioning that the mesh independence test is also carried out during the mesh size selection. Seven meshes changing from normal to ultra-fine meshes are considered. Meshes with a smaller number of elements provide an inaccurate estimation of the acoustic field. After increasing the number of elements to 1,161,279, there is no significant change in the results, so this mesh is selected as the optimum mesh to evaluate the acoustic field for this study (see ). In this case, the ratio between the maximum mesh element size (H) and the minimum mesh element size (h) is H/h=5 (in which H=λ/5 and λ is wavelength). To optimize the number of elements, the maximum element size has been decreased, while maintaining the minimum element size constant.
For heat transfer modeling in the computational domain, a mesh element size of H/h=15 is chosen for the grids in the tumor area, the same morphology as the grid generation for acoustic modeling but in lower density, whereas in the surrounding healthy tissue, the mesh size gradually increases from the tumor boundary to achieve H/h=35, thereby reducing the computational costs. The time-step is adjusted to 0.01 [s] due to the results of grid independence tests. For concentration (or AUC) analysis, triangular elements have also been used. Like temperature analysis, the H/h=15 case with a total number of 122,572 elements is selected for solving drug transport equations because of its acceptable accuracy and capability to reduce computational costs ().
2.7. Solution strategy and simulation cases
The commercial finite element simulation tool COMSOL Multiphysics™ 6.0 (COMSOL Multiphysics Modeling Software, Stockholm, Sweden) is employed to model FUS-triggered nano-sized TSLs. A 2D-axisymmetric computational domain is simulated to attain a computation time in a reasonable range. The coupled acoustic and thermal fields calculate the temperature distribution by simultaneously solving the Helmholtz equation and BHTE. Frequency domain and time-dependent parameters were used. After determining acoustic and thermal fields, to obtain drug concentrations, there are two distinct solution phases in the current study: steady-state and transient. First, IFP and IFV are achieved via the momentum and continuity equations for interstitial fluid flow in the steady-state phase. Then, in a transient phase, distributions of different drug concentrations (CL, CF, CB) are obtained by solving mass transport equations, as listed in . The injected dose of TSL is 0.019 [kg/m3], which carries drug DOX molecules and is delivered through the intravascular release paradigm. Subsequently, the survival fraction of cancerous cells is calculated by solving a pharmacodynamic equation based on intracellular drug concentration. demonstrates a step-by-step flowchart of this process. An AMD Ryzen 9 5950 × 16-Core 3.40-GHz Processor with 64 GB RAM was used for the computational simulations.
2.8. Drug release rate from nanocarriers
Mathematical models of ultrasound-induced drug release are typically empirically derived from experimental data (Hornsby et al., Citation2022). Published TSL formulations in the literature (Tagami et al., Citation2012) still enable some DOX agents to be released gradually at 37 °C, although the release is much faster in mild hyperthermia range (at around 42 °C) (Kashkooli et al., Citation2020; Haemmerich et al., Citation2023). Release rate constants at different temperatures between 37 to 42 °C are given in for ultra-fast, fast, and normal TSL drug release. ThermoDOX with ultra-fast release is selected as the baseline in this study, and the fast and slow release will be investigated in section 4.6.
ThermoDOX is designed to rapidly release its content when heated. The composition of the liposome, the method used to prepare it, and the temperature at which it is heated all affect the actual release rate (Tagami et al., Citation2011). The release rate also needs to be very low in the circulation so that the nanocarrier remains in circulation for a prolonged period and side effects are minimized. According to the literature, ThermoDOX has good stability at body temperature with a release rate of 3 × 1 0−4 (s−1) (Centelles et al., Citation2018). ThermoDOX will be effective when it releases its contents rapidly at high temperatures. ThermoDOX can release its drug payload explosively at a temperature of 43 °C with a release rate of 0.3 (s−1) (Gasselhuber et al., Citation2012), in less than a minute at temperatures within their melting range at 39–40 °C (Needham & Dewhirst, Citation2001).
3. Validation of the computational model
A validation of the developed computational model is presented in this section. As the model consists of several physics modules, each is compared separately with the previously published experimental or computational results to confirm the accuracy of numerical calculation and computational approach. As shown in , we first compared our results for a geometry filled with water to LATS, a previously developed accurate software for linear acoustic and thermal simulations (Butt, Citation2011; Butt et al., Citation2011; Shaswary et al., Citation2021). Results show excellent agreement (with roughly a 1.33% discrepancy). Then, we selected a previously published study to assess the thermal field. Following the approach of Singh et al. (Singh et al., Citation2021), we first verified acoustic intensity in the geometry containing water and tissue (). Here, a concave transducer with a center frequency of 1 MHz, aperture diameter of 70 mm, and a 65 mm focal length was considered the FUS source with 15 W acoustic power and an exposure time of 5.6 s. In the following, the numerical methodology has been verified for the thermal field based on Pennes’ BHTE (). Results of temperature rise in the region of interest are in very good correspondence with Singh et al. (Singh et al., Citation2021), with a discrepancy of about 1.8%.
Figure 5. (a) and (b) A comparison of our results for acoustic fields with LATS in longitudinal and Lateral directions for water only. (c) and (d) Validation of acoustic and thermal fields of our simulation with Singh et al. (Singh et al., Citation2021), in which they applied FUS (15 W continuously for 5.6 s) on a domain containing water and muscle tissue. (e) Validation of the interstitial fluid flow (IFP and IFV) with those of literature (Soltani & Chen, Citation2011; Kashkooli et al., Citation2020; Souri et al., Citation2021). the yellow line and the contours in background (for spatial distribution of IFP and IFV) demonstrates our simulation results.

To validate the results of the present study for interstitial fluid flow (i.e. IFP and IFV), the studies conducted by Soltani and Chen (Soltani & Chen, Citation2011), Souri et al. (Souri et al., Citation2021), and Kashkooli et al. (Kashkooli et al., Citation2020) are examined for a tumor surrounded by healthy tissue, as demonstrated in . IFP is a clinically important measure, particularly in tumor treatment using chemotherapeutic agents (Baxter & Jain, Citation1989). Additionally, IFP has a crucial role in the transport of therapeutic agents within the tumor extracellular matrix. The elevated IFP is one of the main barriers against effective delivery of drug to solid tumors by creating outward convection in the tumor borders versus inward diffusion (Moradi Kashkooli & Soltani, Citation2021). Overall, according to , the highest IFP levels can be observed in the tumor due to the lack of a functional lymph drainage system in the tumor region as well as higher tumor permeability of vasculature (Jain & Baxter, Citation1988; Stylianopoulos et al., Citation2013). The results of the present study for the IFP prediction are in good agreement with those of Soltani and Chen (Soltani & Chen, Citation2011) and Souri et al. (Souri et al., Citation2021), as demonstrated in . The average maximum IFP inside tumor agrees reasonably well with those in the computational analysis of Boucher et al. (Boucher et al., Citation1990), and also with the experimental outcomes of Huber et al. (Huber et al., Citation2005) and Boucher and Jain (Boucher & Jain, Citation1992). According to Darcy’s law, the IFV amount is proportional to the gradient of IFP. Because the gradient of IFP is distributed uniformly throughout the tumor, the IFV values is very low within the central zones of the tumor (Zhao et al., Citation2007; Pishko et al., Citation2011). The maximum IFV in our simulations occurs close to the tumor boundary, where the IFP reduction is steep, which matches the values in the previously published experimental and computational studies (Butler et al., Citation1975; Pishko et al., Citation2011). In addition, the results of this study for IFV have good correspondence with those of Kashkooli et al. (Kashkooli et al., Citation2020), as shown in . The current study’s low IFV values are also in line with the recent in vivo investigation by Islam et al. (Islam et al., Citation2021). Therefore, the current model is considered reliable enough to predict the interstitial fluid flow characteristics in solid tumors.
4. Results and discussion
Results of the computational modeling of FUS heat-induced nano-sized DDSs are presented in this section. Acoustic and temperature profiles, drug transport in the tumor, and cellular drug uptake are shown in separate sections. Subsequently, two important factors in FUS-induced nano-sized DDSs, including the release rate of drug from TSLs and exposure duration of FUS, are also investigated in detail.
4.1. Acoustic and thermal field distribution
For a successful FUS-activated nano-sized DDS, ultrasonic energy should be delivered to a particular region of interest. In this work, the transducer’s focal point was placed at the tumor center. We presented acoustic intensity variations across the longitudinal direction in the entire water domain in section 3 in validation of the computational model. The distributions of ultrasound pressure and intensity throughout the simulated tissue domain for our main case study, which comprises water and tissue, are shown in . The maximum amplitudes of the focal acoustic pressure and intensity are roughly 2.12 MPa and 123 W/cm2, respectively. However, in addition to the values of maximum pressure and intensity amplitudes, the ultrasound beam’s sufficient coverage of the target region of interest is another significant factor affecting the release of drugs in the targeted area. In our case study, ultrasonic energy covers almost the entire tumor region volume, where the diameter of the tumor is 12 mm.
Figure 6. (a) the spatial distribution of acoustic intensity and pressure in the computational domain. It should be mentioned that since acoustic field is solved in a frequency domain, so the acoustic pressure and intensity profiles are not changing over time; (b) Spatiotemporal temperature distribution in tumor tissue (maximum value: 43 °C) considering temperature controller 15 min after appling FUS.

The temperature profile in the focal area is elliptical, consistent with the focal spot shape from the ultrasound transducer. The temperature is calculated by solving the BHTE. To prevent thermal damage to the healthy tissue and blood vessels, by adjusting the input power, a PI controller has been utilized to achieve the desired temperature range in the ROI, i.e. to regulate the temperature into the hyperthermia range. Temperature variations induced by FUS heating over time in blood microvessels and tumor are shown in . The maximum temperature of the tumor, which occurs at the focal point, quickly reaches 43 °C, and the mean temperature of the tumor reaches 40.4 °C, and it then remains at this level as long as the FUS transducer is on. The temperature drops gradually after the transducer is turned off. Since the focal area of FUS is directly positioned within the tumor and exposed to heating, tumor temperature increases faster than healthy tissue.
The spatial mean temperature of tissue at 20 min post-heating is demonstrated in . The maximum temperature occurs in the focal area, where the tumor is directly exposed to the FUS. The increase in temperature in the surrounding area results from heat exchange via convection and conduction mechanisms from the focal area; thus, healthy tissue temperature is less than tumor temperature. The difference (2–3 °C) in spatial mean temperature between the tumor and healthy tissues increases drug release in the tumor area while minimizing the bioavailability of the drug in surrounding healthy tissues.
4.2. Drug transport in tumor
Temporal profiles of TSLs in the systemic circulation, tumor microvessels, and tumor interstitium are shown in . By bolus intravenous administration of drug-loaded TSL, the concentration of TSL at the initial moments in intravascular space is equal to the injection dose. As shown in , the TSL concentration in systemic circulation gradually decreases, while TSLs experience different drug release kinetics in tumor microvessels, dependent on FUS heating time. The large vessel-wall pore size in the tumor site (50 nm (Stylianopoulos et al., Citation2015; Stylianopoulos & Jain, Citation2013)<d < 2 μm in diameter (McDonald & Baluk, Citation2002; Stylianopoulos & Jain, Citation2013; Stylianopoulos et al., Citation2015)) allows some TSLs to enter the TME through a passive mechanism (i.e. EPR effect), which increases TSL concentration in the tumor interstitium. This means that for large-size nanocarriers like TSLs, the concentration gradient term does not play a significant role in the transvascular exchange from microvessels into the interstitium due to the relatively low permeability. However, the convection term is much more significant since the IFP value in the periphery of the tumor is much lower than the intravascular pressure. Therefore, a small amount of TSLs enter the interstitium due to the plasma flow. For this reason, only a small number of TSLs had a chance to enter the central areas of the tumor, while the TSLs could enter the tumor periphery more readily. After a short time, due to the rapid drug release within tumor microvessels, almost no TSL could enter the tumor interstitium; however, after the microvessel cooling process at the end of the ultrasound exposure, some TSLs have the chance of entering the extracellular space. Subsequently, after reaching a maximum TSL concentration in the tumor interstitium, it gradually dropped to zero owing to the fast removal in the circulation system and increased drug release resulting from the temperature increase. In other words, the TSL concentration reaches its maximum and reduces progressively to zero due to drug release and reduced intravascular concentration ().
Figure 7. TSL concentration in (a) microvessels and (b) interstitium as a function of time. Note that the ultrasound exposure stops at t = 0.5 h.

Free DOX is constantly released from TSLs by applying FUS heating, leading to a fast rise in drug concentrations, both in intravascular and extracellular spaces, followed by a gradual decrease in concentrations of the drug after reaching their maximum (). This is due to cancer cell drug uptake and the reduction in intravascular TSL concentration over time (). These results also reflect that temporal variation in the concentration of free drug () and follows a similar trend as the concentration of protein-bound drug (), even though the concentration of the protein-bound drug is 2 to 3 times higher in magnitude than free drug concentration.
4.3. Cellular uptake of drug
Time-dependent variation of the drug’s intracellular concentration and tumor cells’ survival fraction are shown in . Concentration in intracellular space increases to its maximum value at about 3 h post-administration of DOX-loaded TSL. Then it gradually decreases to zero as time proceeds (). The transmembrane rate (i.e. the rate of drug intake by the cell) is weaker than the diffusion of the drug. Therefore, unused drugs are spread throughout the tumor over time, creating a high concentration of intracellular drug within the tumor after a few hours, while free drug reaches its peak after only half an hour. The maximum of intracellular drug concentration occurs a few hours after the extracellular space drug concentration peak because of the relatively slow transmembrane rate. The efficacy of treatment is assessed with regard to dynamic cell density predicted via a pharmacodynamics model (see , part 5). As shown in , the survival fraction of cancer cells begins to decrease post-administration immediately, but after 13 h, drug-induced cell killing declines until 15 h when the cell killing rate drops to zero, and proliferation of cells overcomes cell killing.
4.6. Effects of drug release rate from nanocarriers and FUS exposure time
Advanced DDSs with precise control over release kinetics are needed for next-generation therapies (Kashkooli et al., Citation2020; Sarmadi et al., Citation2022). According to biological, physicochemical, and mathematical principles, various methods can be used for spatiotemporal controlled release and delivery of therapeutic agents, which is necessary for optimizing DDSs. This will lead to increased treatment efficacy, enhanced patient convenience, and improved safety. Encapsulating drugs in TSLs offers great bioavailability and lowers side effects. The intravascular drug release provides a significant drug level in a comparatively small tumor volume. In the current study, drugs are released in the intravascular space, so nanocarriers do not need to aggregate in the tissue’s extracellular matrix. In less than a minute, intravenous administrations expose the entire body to TSLs (Dewhirst & Secomb, Citation2017). Here, the main question is, ‘what are the effects of different drug release rates on treatment efficacy?’. Therefore, we chose three different release rates of DOX from TSLs in response to heat induced by FUS, namely ultra-fast, fast, and slow release rates (see for details).
On the other hand, optimizing the FUS exposure time for intravascular drug release requires in-depth consideration of different factors. Since TSL circulation lasts for roughly 5 h (Gasselhuber et al., Citation2012; Liu & Xu, Citation2015), this study also aims to answer another question: ‘what is the optimum exposure time of applying FUS for intravascular release of drug?’. The importance of this parameter is that if the period is too prolonged, the patient’s convenience may be an issue, and if it is too short, the treatment approach may not reach its maximum therapeutic potential. Therefore, an optimized exposure time should be chosen. Various regimens, including continuous exposure of FUS for 10, 30, and 60 min following intravenous injection of TSLs, were investigated.
In this DDS, one of the main challenge is to maintain the needed temperature rise (39.5 ̊C to 43 ̊C for ThermoDox) over the whole tumor domain for a time period consistent with the therapeutic agents’ circulating duration (30–60 min when considering a long-circulating TSLs such as ThermoDox) (Lyon et al., Citation2017; Citation2021). As typically the temperature profile highly depends on the acoustic power, in this study due to the specific controller design, changing the acoustic power has no effect on the target temperature profile. To achieve an appropriate hyperthermia temperature range during the simulation, a feedback temperature controller is employed to regulate the input power based on the temperature specified in the region of interest (Tset in ) (Gasselhuber et al., Citation2012; Rezaeian et al., Citation2019; Zhan et al., Citation2019; Staruch et al., Citation2011). This controller’s function is to prevent tumor site temperature from rising above 43 °C in order to protect neighboring healthy tissues from irreversible damage.
In a preclinical setting, only a few studies have looked at thermal procedures, their combination with temperature-induced drug transport and delivery, and their influence on cancer treatment (Hijnen et al., Citation2017). Therefore, we have been examined nine scenarios to study the effects of drug release rate and simultaneously FUS exposure time (). Based on the literature, for clinical use, hyperthermia for 30 to 60 min following TSL injection appears to be an appropriate balance between achievable amounts of drug, technical challenges with maintaining controlled hyperthermia, and patient comfort (Grüll & Langereis, Citation2012; Jain et al., Citation2018). In general, the AUC of the free drug increases when the treatment time is prolonged, maintaining the concentration at a greater level. In 10 min exposure time of FUS, the AUC value is less than 30 and 60 min, because this time is too short for FUS exposure. But for 30 and 60 min FUS exposure, in which the exposure time is long enough for drug release, the higher the release rate, the higher the AUC value. The slow release rate always has the lowest amount of AUC for the different exposure times and scenarios. In the 10 min exposure, the ultrafast release rate provided a lower AUC than the fast release because it is an optimal pattern for intravascular release and has the lowest release rate in the body temperature. However, in 30 and 60 min FUS exposures, the ultrafast release has the highest AUC compared to fast and slow release rates. Although 60 min has a higher AUC compared to 30 min (251 vs. 233 [µg/mL·min]), 30 min of FUS exposure is more practical in the clinic in terms of patient comfort and clinical limitations. In addition, the difference in AUC value is not large. It should also be noted that there is no statistically significant variation in AUC between the various cases for slow release rate at the different FUS exposure times. Consequently, a useful therapeutic outcome is provided by 30 min of FUS exposure combined with ultrafast drug release from TSLs ().
4.7. Sensitivity analysis on drug transport parameters
In this section, we have conducted a further sensitivity analysis on the effective parameters to determine which diffusion and transmembrane rate had the highest impact on the concentration profiles. In , we have added the results of the sensitivity analysis on nanoparticle size, which is inversely related to the diffusion coefficient in interstitial space (Stylianopoulos et al., Citation2015; Kashkooli et al., Citation2022). Small nanoparticles have greater diffusion and accumulate better in the extracellular space; however, the released drugs within the microvascular network mainly impact free drug concentration in extracellular and intracellular spaces almost equally for 50 nm and 100 nm sizes. Here, we have assumed that the loading capacity for the 50 nm and 100 nm nanoparticles is the same, even though published evidence suggests that 100 nm nanoparticles have a higher loading capacity. Larger nanoparticles (for example, 750 nm) are removed quickly due to their higher clearance rate; hence, it causes a poor therapeutic response. On the other hand, the effect of the transmembrane rate of DOX (50% increase or decrease) on intracellular concentration level is shown in . Results clearly showed that the higher the value of the transmembrane rate of the drug, the higher the concentration level.
4.8. Potentials, limitations and future works
Computational models could offer a more complete description of nano-bio interactions, which is an important challenge for understanding larger-scale processes at the mechanistic level. In in vivo models, how drugs are distributed and delivered and their impact on cell death is challenging to explain empirically. Approaches such as mathematical modeling cannot capture all processes in an in vivo model, particularly spatial distribution variations in tumor tissue. Using computational models to assess therapeutic agent behavior at various transport phases, combined with limited animal model data, is a promising approach. In summary, while mathematical models are valuable tools, they cannot be parameterized and validated without complementary empirical measurements and thus cannot reach their full potential. In vivo models, on the other hand, are difficult to use in the study of nano-bio interactions, and it is difficult to gather quantitative information. As a result, linking computational and in vivo models could assist in clinical translation (Kashkooli et al., Citation2021). In the case of the present study, we have presented a comprehensive multi-scale and multi-physics computational model to investigate different transport phenomena starting from the injection to transport in different environments like the microvasculature and interstitial space and cells, and finally delivery to the cells. Additionally, we have modeled the ultrasound beam propagation physics and its effect on the tissue through the BHTE and evaluated the effect of different exposure parameters. This model can serve as a platform for analyzing the problem of ultrasound-activated nanosized DDS, developing new models, and for the qualitative and quantitative examination of ultrasound-mediated drug delivery to solid tumors.
Mathematical modeling, as a cost-efficient approach, can facilitate the analysis process through complete parametric studies to determine the most effective parameters and optimize their ranges to provide a guideline for the efficient design of both drug-loaded TSLs as treatment plans. However, four major assumptions have been made in the present study, including (i) A 2D-axisymmetric simulation model is utilized because of the computational cost of 3D models; (ii) Physical complexities of acoustic waves such as cavitation and non-linear propagation are not taken into account; (iii) Effects of temperature on tumor characteristics is complex and some of them are not included in the present study (e.g. the dependence of plasma clearance on temperature); (iv) Focal area is ideally located in the tumor, regardless of the actual geometry of microvasculature and some other characteristics of TME like necrotic core; (v) Main focus of the current study is on the thermal effects of ultrasound and any mechanical impacts (including sonoporation) were not explored. In a domain with uniform distribution of microvessels, a 2D-axisymmetric model can provide reasonable results. However, owing to the complexity of the different physics and the number of partial differential equations, 3D modeling is very time-consuming. Due to our model’s complexity and comprehensiveness, it includes many partial differential equations, tumor parameters, drug properties, and ultrasound exposure parameters. The proposed model has been verified in various parts, but since averaged and representative parameter values have been utilized in the numerical modeling, the results of this study can be mainly used for qualitative analysis instead of quantitative prediction. Multiple studies in the literature also demonstrated that for the focal intensities between 100 and 1000 W/cm2, the maximum negative pressure between 1 and 4 MPa, the complications like cavitation and highly non-linear propagation could be ignored with reasonable errors (Hallaj & Cleveland, Citation1999; Filonenko & Khokhlova, Citation2001; Sheu et al., Citation2011).
One of the most important concerns in intravascular drug release is the possibility of drugs penetrating surrounding healthy tissues. However, the drug concentration in the circulatory system in the present study is in a low range, which the published literature (Ten Hagen et al., Citation2021; Jadidi et al., Citation2022) suggests does not cause serious side effects (Figure S1). In Figure S1, we have also compared our results for the intravascular drug release paradigm with Souri et al. (Souri et al., Citation2021) for free chemotherapy, where the maximum of the drug in microvasculature is lower than for free chemotherapy. This reveals a lower potential of drug penetration into healthy tissues when compared to for free chemotherapy. It should also be mentioned that at some point, the concentration of DOX would be higher in tumor tissue, leading to a backflush of Dox to the vessels through diffusion. Indeed, due to the exchange term (), backflush to the bloodstream is also possible as the Pe number includes diffusion and convection terms. On the other hand, TSL compositions may be unstable at normal body temperatures in the human circulatory system (Tagami et al., Citation2011). The ideal TSL should be capable of releasing its contents only in response to a few degree temperature raise (Zou et al., Citation1993). Drug release at normal body temperature can result in the accumulation of the free drug in the bloodstream damaging healthy cells and increasing systemic side effects, including cardiotoxicity (Legha et al., Citation1982). Hence, future studies on developing TSLs should aim to minimize leakage of drug at normal body temperature, improve the release rate of drug from TSLs at predetermined temperature, and optimize hyperthermia treatment planning (Liu & Xu, Citation2015). For future works of our group, low-intensity pulsed ultrasound (LIPUS) and FUS are two therapeutic ultrasound exposure approaches which will be used to activate drugs from two common nanocarriers (ThermoDox and gold nanoparticles), both theoretically and experimentally. LIPUS transducers normally produce unfocused ultrasound beams with a low acoustic intensity range. In comparison, FUS’s focal intensity varies from a few W/cm2 to thousands W/cm2. It should be noted that ThermoDox’s primary mechanism governing drug release is temperature driven, while for gold nanocarriers, drug release is governed by both thermal and mechanical mechanisms. Additionally, ultrasound-activated DDSs using different administration approaches (e.g. intratumoral and intraperitoneal) of therapeutic agents will also be investigated.
5. Conclusions
Targeted DDSs using TSLs and an external field like ultrasonic energy for activating drug release is a promising therapeutic approach. However, several factors, including the formulation of the DDSs, the properties of the drugs, and the characteristics of the cancer cells and TME, influence the successful designs of DDSs. A multi-scale computational model of FUS-triggered nano-sized DDS to solid tumors is proposed in this study to evaluate treatment efficacy. The main aim of the presented computational modeling is to study and understand the significant interactions between the TME and FUS-triggered nano-sized DDS. The spatiotemporal distribution of drug-loaded TSLs, the temperature response of FUS heating, and cellular uptake of drug for a combination of FUS and DOX-loaded TSL delivery systems are studied through simulations. First, the acoustic and thermal fields are simulated. Using the temperature gradient in the tumor, drugs are released in different areas at a specific rate. The drug transport process in various tumor compartments is taken into account using fully-coupled equations of fluid flow in the interstitium and mass transport in tissue and cellular spaces. Eventually, distributions of acoustic and thermal fields, IFV, IFP, various concentrations, AUC, and the fraction of survival cells post-treatment are determined according to physiological data and biological factors. Results demonstrate that, in an intravenous administration of TSLs for intravascular release of drug, treatment should be prolonged until achieving the maximum free drug concentration. This will maximize tumor cell killing. The maximum concentration in the current study was reached at about 25 min. Applying 30 min FUS is the best option among different cases in terms of both therapeutic efficacy and patient comfort because it is more realistic and feasible for clinical trials. Additionally, the ultrafast release of drug DOX from TSLs has shown better compatibility with intravascular drug release, leading to higher therapeutic efficacy. Results also show the ability of the current approach to model a complex system, demonstrating this DDS’s capability for achieving a localized, targeted therapy along with improved delivery of drug in TME while maintaining a relatively small drug concentration level in healthy tissue. The study demonstrates how mathematical modeling and computational tools can be created synergistically to simulate the role of FUS in drug release from TSLs and drug transport in tumor tissue. The proposed framework for mathematical modeling can serve as a platform for future studies on FUS heat-induced nano-sized DDSs.
Authors’ contributions
Farshad Moradi Kashkooli: Conceptualization, Investigation, Methodology, Formal analysis, Software, Visualization, Validation, Discussion, Writing-original draft, Project administration _ Mohammad Souri: Methodology, Formal analysis, Software, Visualization, Validation, Discussion,Writing—review & editing _ Jahangir(Jahan) Tavakkoli: Investigation, Methodology, Resources, Discussion, Writing—review & editing, Supervision _ Michael C. Kolios: Investigation, Methodology, Resources, Discussion, Writing—review & editing, Supervision, Funding acquisition.
Ethical approval statement
NA.
List of Abbreviations
AUC | = | area under the curve |
BC | = | boundary condition |
BHTE | = | bio-heat transfer equation |
CDR | = | convection-diffusion-reaction |
DDSs | = | drug delivery systems |
DOX | = | doxorubicin |
EPR | = | enhanced permeability and retention |
FUS | = | focused ultrasound |
HIFU | = | high intensity focused ultrasound |
IFP | = | interstitial fluid pressure |
IFV | = | interstitial fluid velocity |
LATS | = | linear acoustic and temperature simulator |
LIPUS | = | low-intensity pulsed ultrasound |
NPs | = | nanoparticles |
PI | = | proportional–integral |
PML | = | perfectly matched layer |
TME | = | tumor microenvironment |
TSLs | = | temperature-sensitive liposomes |
Supplemental Material
Download MS Word (165.6 KB)Acknowledgements
This research was funded by Toronto Poly Clinic Inc., Ontario Research Fund - Research Excellence (ORF-RE #RE02-032), the Natural Sciences and Engineering Research Council of Canada (NSERC) Alliance grant (ALLRP 556270-20), and NSERC Discovery grants that were awarded to J. Tavakkoli (RGPIN-2022-03799) and M.C. Kolios (RGPIN-2022-04143). F.M. Kashkooli is also supported by an NSERC Banting Postdoctoral Fellowship, administered by the Government of Canada.
Data availability statement
The data supporting this work are accessible upon reasonable request from the corresponding author.
Disclosure statement
No potential conflict of interest was reported by the authors.
Correction Statement
This article has been corrected with minor changes. These changes do not impact the academic content of the article.
References
- Ahmadi A, Hosseini-Nami S, Abed Z, et al. (2020). Recent advances in ultrasound-triggered drug delivery through lipid-based nanomaterials. Drug Discov Today 25:1–21.
- Baxter LT, Jain RK. (1989). Transport of fluid and macromolecules in tumors. I. Role of interstitial pressure and convection. Microvasc Res 37:77–104.
- Baxter LT, Jain RK. (1990). Transport of fluid and macromolecules in tumors. II. Role of heterogeneous perfusion and lymphatics. Microvasc Res 40:246–63.
- Baxter LT, Jain RK. (1991). Transport of fluid and macromolecules in tumors: III. Role of binding and metabolism. Microvasc Res 41:5–23.
- Boissenot T, Bordat A, Fattal E, Tsapis N. (2016). Ultrasound-triggered drug delivery for cancer treatment using drug delivery systems: from theoretical considerations to practical applications. J Control Release 241:144–63.
- Boucher Y, Baxter LT, Jain RK. (1990). Interstitial pressure gradients in tissue-isolated and subcutaneous tumors: implications for therapy. Cancer Res 50:4478–84.
- Boucher Y, Jain RK. (1992). Microvascular pressure is the principal driving force for interstitial hypertension in solid tumors: implications for vascular collapse. Cancer Res 52:5110–4.
- Butler TP, Grantham FH, Gullino PM. (1975). Bulk transfer of fluid in the interstitial compartment of mammary tumors. Cancer Res 35:3084–8.
- Butt F. (2011). High performance computing for linear acoustic wave simulation, Ryerson University, 1–127.
- Butt F, Abhari A, Tavakkoli J. (2011). An application of high performance computing to improve linear acoustic simulation, Conference, Spring Simulation Multi-conference, SpringSim, Boston, MA. Volume 3: Proceedings of the 14th Communications and Networking Symposium (CNS), 71–8.
- Centelles MN, Wright M, So P-W, et al. (2018). Image-guided thermosensitive liposomes for focused ultrasound drug delivery: using NIRF-labelled lipids and topotecan to visualise the effects of hyperthermia in tumours. J Control Release 280:87–98.
- Chabner BA, Longo DL. (2011). Cancer chemotherapy and biotherapy: principles and practice. Wolters Kluwer; Lippincott Williams & Wilkins.
- Couture O, Foley J, Kassell NF, et al. (2014). Review of ultrasound mediated drug delivery for cancer treatment: updates from pre-clinical studies. Transl Cancer Res 3:494–511.
- Danhier F. (2016). To exploit the tumor microenvironment: since the EPR effect fails in the clinic, what is the future of nanomedicine? J Control Release 244:108–21.
- Dewhirst MW, Secomb TW. (2017). Transport of drugs from blood vessels to tumour tissue. Nat Rev Cancer 17:738–50.
- Dimcevski G, Kotopoulis S, Bjånes T, et al. (2016). A human clinical trial using ultrasound and microbubbles to enhance gemcitabine treatment of inoperable pancreatic cancer. J Control Release 243:172–81.
- Du J-Z, Du X-J, Mao C-Q, Wang J. (2011). Tailor-made dual pH-sensitive polymer–doxorubicin nanoparticles for efficient anticancer drug delivery. J Am Chem Soc 133:17560–3.
- Eikenberry S. (2009). A tumor cord model for doxorubicin delivery and dose optimization in solid tumors. Theor Biol Med Model 6:1–20.
- Eliaz RE, Nir S, Marty C, Szoka FC. (2004). Determination and modeling of kinetics of cancer cell killing by doxorubicin and doxorubicin encapsulated in targeted liposomes. Cancer Res 64:711–8.
- El-Kareh AW, Secomb TW. (2000). A mathematical model for comparison of bolus injection, continuous infusion, and liposomal delivery of doxorubicin to tumor cells. Neoplasia 2:325–38.
- El-Kareh AW, Secomb TW. (2003). A mathematical model for cisplatin cellular pharmacodynamics. Neoplasia 5:161–9.
- Filonenko E, Khokhlova V. (2001). Effect of acoustic nonlinearity on heating of biological tissue by high-intensity focused ultrasound. Acoust Phys 47:468–75.
- Gao W, Chan JM, Farokhzad OC. (2010). pH-responsive nanoparticles for drug delivery. Mol Pharmaceut 7:1913–20.
- Gasselhuber A, Dreher MR, Partanen A, et al. (2012). Targeted drug delivery by high intensity focused ultrasound mediated hyperthermia combined with temperature-sensitive liposomes: computational modelling and preliminary in vivo validation. Int J Hyperthermia 28:337–48.
- Gasselhuber A, Dreher MR, Rattay F, et al. (2012). Comparison of conventional chemotherapy, stealth liposomes and temperature-sensitive liposomes in a mathematical model. Plos ONE 7:e47453.
- Gasselhuber A, Dreher MR, Rattay F, et al. (2012). Comparison of conventional chemotherapy, stealth liposomes and temperature-sensitive liposomes in a mathematical model.
- Goh Y-MF, Kong HL, Wang C-H. (2001). Simulation of the delivery of doxorubicin to hepatoma. Pharm Res 18:761–70.
- Greene RF, Collins JM, Jenkins JF, et al. (1983). Plasma pharmacokinetics of adriamycin and adriamycinol: implications for the design of in vitro experiments and treatment protocols. Cancer Res 43:3417–21.
- Grüll H, Langereis S. (2012). Hyperthermia-triggered drug delivery from temperature-sensitive liposomes using MRI-guided high intensity focused ultrasound. J Control Release 161:317–27.
- Haemmerich D, Ramajayam KK, Newton DA. (2023). Review of the Delivery Kinetics of Thermosensitive Liposomes. Cancers 15:398.
- Hallaj IM, Cleveland RO. (1999). FDTD simulation of finite-amplitude pressure and temperature fields for biomedical ultrasound. J Acoust Soc Am 105:L7–12.
- Hijnen N, Kneepkens E, de Smet M, et al. (2017). Thermal combination therapies for local drug delivery by magnetic resonance-guided high-intensity focused ultrasound. Proc Natl Acad Sci U S A 114:E4802–E4811.
- Hornsby TK, Jakhmola A, Kolios MC, Tavakkoli J. (2023). A Quantitative study of thermal and non-thermal mechanisms in ultrasound-induced nano-drug delivery. Ultrasound Med Biol 49:1288–98.
- Hornsby T, Kashkooli FM, Jakhmola A, et al. (2022). Measuring drug release induced by thermal and non-thermal effects of ultrasound in a nanodrug delivery system. 2022 IEEE International Ultrasonics Symposium (IUS), IEEE, 1–4.
- Hornsby TK, Moradi Kashkooli F, Jakhmola A, et al. (2023). Multiphysics modeling of low-intensity pulsed ultrasound induced chemotherapeutic drug release from the surface of gold nanoparticles. Cancers 15:523.
- Huber PE, Bischof M, Jenne J, et al. (2005). Trimodal cancer treatment: beneficial effects of combined antiangiogenesis, radiation, and chemotherapy. Cancer Res 65:3643–55.
- Hynynen K. (2011). MRIgHIFU: a tool for image-guided therapeutics. J Magn Reson Imaging 34:482–93.
- Islam MT, Tang S, Tasciotti E, Righetti R. (2021). Non-invasive assessment of the spatial and temporal distributions of interstitial fluid pressure, fluid velocity and fluid flow in cancers. Vivo, IEEE Access 9:89222–33. In PP
- Izadifar Z, Babyn P, Chapman D. (2017). Mechanical and biological effects of ultrasound: a review of present knowledge. Ultrasound Med Biol 43:1085–104.
- Jadidi A, Shokrgozar MA, Sardari S, Maadani AM. (2022). Gefitinib-loaded polydopamine-coated hollow mesoporous silica nanoparticle for gastric cancer application. Int J Pharm 629:122342.
- Jain RK. (1987). Transport of molecules across tumor vasculature. Cancer Metastasis Rev 6:559–93.
- Jain RK, Baxter LT. (1988). Mechanisms of heterogeneous distribution of monoclonal antibodies and other macromolecules in tumors: significance of elevated interstitial pressure. Cancer Res 48:7022–32.
- Jain A, Tiwari A, Verma A, Jain SK. (2018). Ultrasound-based triggered drug delivery to tumors. Drug Deliv Transl Res 8:150–64.
- Karimi M, Ghasemi A, Zangabad PS, et al. (2016). Smart micro/nanoparticles in stimulus-responsive drug/gene delivery systems. Chem Soc Rev 45:1457–501.
- Kashkooli FM, Jakhmola A, Hornsby TK, et al. (2023). Ultrasound-mediated nano drug delivery for treating cancer: fundamental physics to future directions. J Control Release 355:552–78.
- Kashkooli FM, Rezaeian M, Soltani M. (2022). Drug delivery through nanoparticles in solid tumors: a mechanistic understanding. Nanomedicine (Lond) 17:695–716.
- Kashkooli FM, Soltani M, Rezaeian M, et al. (2020). Effect of vascular normalization on drug delivery to different stages of tumor progression: in-silico analysis. J Drug Delivery Sci Technol 60:101989.
- Kashkooli FM, Soltani M, Souri M, et al. (2021). Nexus between in silico and in vivo models to enhance clinical translation of nanomedicine. Nano Today 36:101057.
- Kashkooli FM, Soltani M, Souri M. (2020). Controlled anti-cancer drug release through advanced nano-drug delivery systems: static and dynamic targeting strategies. J Control Release 327:316–49.
- Kim C, Guo Y, Velalopoulou A, et al. (2021). Closed-loop trans-skull ultrasound hyperthermia leads to improved drug delivery from thermosensitive drugs and promotes changes in vascular transport dynamics in brain tumors. Theranostics 11:7276–93.
- Kneidl B, Peller M, Winter G, et al. (2014). Thermosensitive liposomal drug delivery systems: state of the art review. Int J Nanomedicine 9:4387–98.
- Legha SS, Benjamin RS, Mackay B, et al. (1982). Reduction of doxorubicin cardiotoxicity by prolonged continuous intravenous infusion. Ann Intern Med 96:133–9.
- Liu C, Xu XY. (2015). A systematic study of temperature sensitive liposomal delivery of doxorubicin using a mathematical model. Comput Biol Med 60:107–16.
- Löke DR, Helderman RF, Franken NA, et al. (2021). Simulating drug penetration during hyperthermic intraperitoneal chemotherapy. Drug Deliv 28:145–61.
- Lyon PC, Gray MD, Mannaris C, et al. (2018). Safety and feasibility of ultrasound-triggered targeted drug delivery of doxorubicin from thermosensitive liposomes in liver tumours (TARDOX): a single-centre, open-label, phase 1 trial. Lancet Oncol 19:1027–39.
- Lyon PC, Gray M, Mannaris C, et al. (2019). Results of first-in-man proof of concept study of ultrasound-triggered drug delivery in liver tumours. Clin Radiol 74:e20.
- Lyon PC, Griffiths LF, Lee J, et al. (2017). Clinical trial protocol for TARDOX: a phase I study to investigate the feasibility of targeted release of lyso-thermosensitive liposomal doxorubicin (ThermoDox®) using focused ultrasound in patients with liver tumours. J Ther Ultrasound 5:1–8.
- Lyon PC, Mannaris C, Gray M, et al. (2021). Large-volume hyperthermia for safe and cost-effective targeted drug delivery using a clinical ultrasound-guided focused ultrasound device. Ultrasound Med Biol 47:982–97.
- McDonald DM, Baluk P. (2002). Significance of blood vessel leakiness in cancer. Cancer Res 62:5381–5385.
- Mi P. (2020). Stimuli-responsive nanocarriers for drug delivery, tumor imaging, therapy and theranostics. Theranostics 10:4557–88.
- Mihcin S, Melzer A. (2018). Principles of focused ultrasound. Minim Invasive Ther Allied Technol 27:41–50.
- Moradi Kashkooli F, Soltani M. (2021). Evaluation of solid tumor response to sequential treatment cycles via a new computational hybrid approach. Sci Rep 11:1–15.
- Moradi Kashkooli F, Soltani M, Momeni MM, Rahmim A. (2021). Enhanced drug delivery to solid tumors via drug-loaded nanocarriers: an image-based computational framework. Front Oncol 11:2252.
- Mura S, Nicolas J, Couvreur P. (2013). Stimuli-responsive nanocarriers for drug delivery. Nat Mater 12:991–1003.
- Namakshenas P, Mojra A. (2023). Efficient drug delivery to hypoxic tumors using thermosensitive liposomes with encapsulated anti-cancer drug under high intensity pulsed ultrasound. Int J Mech Sci 237:107818.
- Needham D, Dewhirst MW. (2001). The development and testing of a new temperature-sensitive drug delivery system for the treatment of solid tumors. Adv Drug Deliv Rev 53:285–305.
- Papaioannou L, Avgoustakis K. (2022). Responsive nanomedicines enhanced by or enhancing physical modalities to treat solid cancer tumors: preclinical and clinical evidence of safety and efficacy. Adv Drug Deliv Rev 181:114075.
- Pishko GL, Astary GW, Mareci TH, Sarntinoranont M. (2011). Sensitivity analysis of an image-based solid tumor computational model with heterogeneous vasculature and porosity. Ann Biomed Eng 39:2360–73.
- Rezaeian M, Sedaghatkish A, Soltani M. (2019). Numerical modeling of high-intensity focused ultrasound-mediated intraperitoneal delivery of thermosensitive liposomal doxorubicin for cancer chemotherapy. Drug Deliv 26:898–917.
- Roohi R, Baroumand S, Hosseinie R, Ahmadi G. (2021). Numerical simulation of HIFU with dual transducers: the implementation of dual-phase lag bioheat and non-linear Westervelt equations. Int Commun Heat Mass Transfer 120:105002.
- Sarmadi M, Ta C, VanLonkhuyzen AM, et al. (2022). Experimental and computational understanding of pulsatile release mechanism from biodegradable core-shell microparticles. Sci Adv 8:eabn5315.
- Schultz CW, Ruiz de Garibay G, Langer A, et al. (2021). Selecting the optimal parameters for sonoporation of pancreatic cancer in a pre-clinical model. Cancer Biol Ther 22:204–15.
- Schutt DJ, Haemmerich D. (2008). Effects of variation in perfusion rates and of perfusion models in computational models of radio frequency tumor ablation. Med Phys 35:3462–70.
- Sedaghatkish A, Rezaeian M, Heydari H, et al. (2020). Acoustic streaming and thermosensitive liposomes for drug delivery into hepatocellular carcinoma tumor adjacent to major hepatic veins; an acoustics–thermal–fluid-mass transport coupling model. Int J Therm Sci 158:106540.
- Seynhaeve A, Amin M, Haemmerich D, et al. (2020). Hyperthermia and smart drug delivery systems for solid tumor therapy. Adv Drug Delivery Rev 163-164:125–44.
- Shaswary E, Assi H, Yang C, et al. (2021). Noninvasive calibrated tissue temperature estimation using backscattered energy of acoustic harmonics. Ultrasonics 114:106406.
- Sheu TW, Solovchuk MA, Chen AW, Thiriet M. (2011). On an acoustics–thermal–fluid coupling model for the prediction of temperature elevation in liver tumor. Int J Heat Mass Transf 54:4117–26.
- Singh G, Paul A, Shekhar H, Paul A. (2021). Pulsed ultrasound assisted thermo-therapy for subsurface tumor ablation: a numerical investigation. J Therm Sci Eng Appl 13:041007.
- Sirsi SR, Borden MA. (2014). State-of-the-art materials for ultrasound-triggered drug delivery. Adv Drug Deliv Rev 72:3–14.
- Solovchuk M, Sheu TW, Thiriet M. (2013). Simulation of nonlinear Westervelt equation for the investigation of acoustic streaming and nonlinear propagation effects. J Acoust Soc Am 134:3931–42.
- Soltani M, Chen P. (2011). Numerical modeling of fluid flow in solid tumors. PloS One 6:e20344.
- Soltani M, Souri M, Moradi Kashkooli F. (2021). Effects of hypoxia and nanocarrier size on pH-responsive nano-delivery system to solid tumors. Sci Rep 11:1–12.
- Souri M, Moradi Kashkooli F, Soltani M. (2022). Analysis of magneto-hyperthermia duration in nano-sized drug delivery system to solid tumors using intravascular-triggered thermosensitive-liposome. Pharm Res 39:753–65.
- Souri M, Soltani M, Kashkooli FM, et al. (2022). Towards principled design of cancer nanomedicine to accelerate clinical translation. Materials Today Bio 13:100208.
- Souri M, Soltani M, Kashkooli FM, Shahvandi MK. (2022). Engineered strategies to enhance tumor penetration of drug-loaded nanoparticles. J Control Release 341:227–46.
- Souri M, Soltani M, Moradi Kashkooli F. (2021). Computational modeling of thermal combination therapies by magneto-ultrasonic heating to enhance drug delivery to solid tumors. Sci Rep 11:1–12.
- Staruch R, Chopra R, Hynynen K. (2011). Localised drug release using MRI-controlled focused ultrasound hyperthermia. Int J Hyperthermia 27:156–71.
- Stylianopoulos T, Economides E-A, Baish JW, et al. (2015). Towards optimal design of cancer nanomedicines: multi-stage nanoparticles for the treatment of solid tumors. Ann Biomed Eng 43:2291–300.
- Stylianopoulos T, Jain RK. (2013). Combining two strategies to improve perfusion and drug delivery in solid tumors. Proc Natl Acad Sci U S A 110:18632–7.
- Stylianopoulos T, Soteriou K, Fukumura D, Jain RK. (2013). Cationic nanoparticles have superior transvascular flux into solid tumors: insights from a mathematical model. Ann Biomed Eng 41:68–77.
- Tagami T, Ernsting MJ, Li S-D. (2011). Optimization of a novel and improved thermosensitive liposome formulated with DPPC and a Brij surfactant using a robust in vitro system. J Control Release 154:290–7.
- Tagami T, May JP, Ernsting MJ, Li S-D. (2012). A thermosensitive liposome prepared with a Cu2+ gradient demonstrates improved pharmacokinetics, drug delivery and antitumor efficacy. J Control Release 161:142–9.
- Tan Q, Zou X, Ding Y, et al. (2018). The influence of dynamic tissue properties on HIFU hyperthermia: a numerical simulation study. Appl Sci 8:1933.
- Ten Hagen TL, Dreher MR, Zalba S, et al. (2021). Drug transport kinetics of intravascular triggered drug delivery systems. Commun Biol 4:1–17.
- Ter Haar G. (2016). HIFU tissue ablation: concept and devices. In: Escoffre JM, Bouakaz A, eds Therapeutic ultrasound. Advances in experimental medicine and biology. Vol 880. Cham: Springer 3–20.
- Tharkar P, Varanasi R, Wong WSF, et al. (2019). Nano-enhanced drug delivery and therapeutic ultrasound for cancer treatment and beyond. Front Bioeng Biotechnol 7:324.
- Ughachukwu P, Unekwe P, Pump E. (2012). Mediated resistance in chemotherapy. Ann Med Health Sci Res 2:191–8.
- Van Durme R, Crevecoeur G, Dupré L, Coene A. (2021). Model-based optimized steering and focusing of local magnetic particle concentrations for targeted drug delivery. Drug Deliv 28:63–76.
- Wang C-H, Li J, Teo CS, Lee T. (1999). The delivery of BCNU to brain tumors. J Control Release 61:21–41.
- Zhan W. (2014). Mathematical modelling of drug delivery to solid tumour. PhD Thesis, Department of Chemical Engineering, Imperial College London, London, England.
- Zhan W, Gedroyc W, Xu XY. (2019). Towards a multiphysics modelling framework for thermosensitive liposomal drug delivery to solid tumour combined with focused ultrasound hyperthermia. Biophys Rep 5:43–59.
- Zhan W, Wang C-H. (2018). Convection enhanced delivery of liposome encapsulated doxorubicin for brain tumour therapy. J Control Release 285:212–29.
- Zhang L, Lin Z, Zeng L, et al. (2022). Ultrasound-induced biophysical effects in controlled drug delivery. Sci China Life Sci 65:896–908.
- Zhao J, Salmon H, Sarntinoranont M. (2007). Effect of heterogeneous vasculature on interstitial transport within a solid tumor. Microvasc Res 73:224–36.
- Zou X, Dong H, Qian S-Y. (2020). Influence of dynamic tissue properties on temperature elevation and lesions during HIFU scanning therapy: numerical simulation. Chinese Phys B 29:034305.
- Zou Y, Yamagishi M, Horikoshi I, et al. (1993). Enhanced therapeutic effect against liver W256 carcinosarcoma with temperature-sensitive liposomal adriamycin administered into the hepatic artery. Cancer Res 53:3046–51.