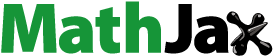
Abstract
Triptorelin is a first-line drug for assisted reproductive technology (ART), but the low bioavailability and frequent subcutaneous injection of triptorelin impair the quality of life of women preparing to become pregnant. We report silk fibroin (SF)-based microneedles (MNs) for transdermal delivery of triptorelin-loaded nanoparticles (NPs) to improve bioavailability and achieve safe and efficacious self-administration of triptorelin. Triptorelin was mixed into an aqueous solution of SF with shear force to prepare NPs to control the release and avoid the degradation of triptorelin by enzymes in the skin. Two-step pouring and centrifugation were employed to prepare nanoparticles-encapsulated polymeric microneedles (NPs-MNs). An increased β-sheet content in the conformation ensured that NPs-MNs had good mechanical properties to pierce the stratum corneum. Transdermal release of triptorelin from NPs-MNs was increased to ∼65%. The NPs-MNs exhibited a prolonged drug half-life and increased relative bioavailability after administration to rats. Surging levels of luteinizing hormone and estradiol in plasma and their subsequent prolonged downregulation indicate the potential therapeutic role of NPs-MNs in ART regimens. The triptorelin-loaded NPs-MNs developed in this study may reduce the physical and psychological burden of pregnant women using ART regimens.
1. Introduction
Infertility is caused by disorders of the male or female reproductive systems. An estimated 48 million couples and 186 million people worldwide suffer from infertility (WHO, Citation2020). Infertility has significant negative impacts upon the physical and mental health of couples, as well as societal impacts, especially for women, who are often burdened with social stigmatization and emotional stress.
To address the problem of infertility, various forms of assisted reproductive technology (ART) have been applied clinically since 1978. More than 8-million children have been born worldwide through ART interventions, such as in vitro fertilization (Adamson et al., Citation2019). In ART regimens, gonadotropin-releasing hormone agonists (GnRHa) have been employed to control luteinizing hormone (LH) secretion to improve pregnancy rates (Fields et al., Citation2013). Triptorelin is one type of GnRHa with high biological potency and has been widely used in ART.
Triptorelin is a synthetic decapeptide analog of GnRH in which the l-glycine of native GnRH is replaced with d-tryptophan at position-6. Triptorelin has 100-fold enhanced activity compared with GnRH. The mechanism of action of triptorelin is divided into two parts. The first part is called the ‘flare-up’ effect. The pituitary gland stimulated to synthesize and secrete LH and follicle-stimulating hormone, which results in increased secretion of gonadal hormones, including estradiol (E2) and testosterone. The second part is termed the ‘downregulation effect.’ The secreted gonadal hormones exert negative-feedback control on the pituitary gland, which results in inhibition of LH secretion, thereby further reducing the secretion of gonadal hormones (Bethesda, Citation2012).
Triptorelin has been administered in three regimens for ART: a long protocol utilizing the downregulation effect, a short protocol utilizing the flare-up effect and downregulation effect, and an ultrashort protocol utilizing the flare-up effect (Siristatidis et al., Citation2015). Triptorelin is commonly marketed as a long-acting injection suspension. However, in long protocols the detrimental effects of treatment depots on embryos in early pregnancy must be considered (Eftekhar et al., Citation2013). Therefore, a short-acting formulation of triptorelin (0.1 mg) has been developed for the clinical regime of daily subcutaneous administration for ART. Daily injections administered by clinical staff in different regimens over 2–15 days impose severe psychological and physical burdens on women receiving ART. In addition, peptides administered by subcutaneous injection can be degraded by enzymes in the skin, resulting in reduced amounts of effective drug entering the bloodstream (Ito et al., Citation2011), thereby exposing women receiving ART to the potential risks posed by greater-than-effective amounts of highly active drugs in their skin.
Microneedle (MN) delivery is an excellent alternative to subcutaneous injections because it is painless and self-administration is possible (Ingrole et al., Citation2021). MNs (50–2000 μm diameter) allow pain-free penetration of the stratum corneum and are beneficial for the transdermal delivery of biomolecules (Larrañeta et al., Citation2016). In particular, drug-loaded polymeric MNs of various types have been studied extensively because of their adequate drug-loading capacity and biodegradability (Singh et al., Citation2019; Sabri et al., Citation2020). Nonetheless, drug-loaded MNs alone are not able to address the enzymatic degradation of peptides in the skin.
With the rapid development of novel drug-delivery systems, nanoparticles (NPs) have been shown to act as ‘shield’ structures protecting incorporated drugs from enzymatic attack (Suchaoin & Bernkop-Schnürch, Citation2017). Recently, nanoparticles-encapsulated polymeric microneedles (NPs-MNs) have become attractive tools allowing synergistic therapeutic approaches for the treatment of diabetes mellitus, cancer and dermatological disease, as well as to aid immunity (DeMuth et al., Citation2012; Yu et al., Citation2015; Wang et al., Citation2016; Lin et al., Citation2019). Among the currently developed NPs-MNs, NPs are usually prepared first using a polymer, and then lyophilized and dispersed in another polymer (usually water-soluble) to prepare MNs. High temperatures or interactions between different polymers can also occur during MNs processing, and such features are not conducive to the stability of NPs (Lan et al., Citation2018; Permana et al., Citation2019).
Silk fibroin (SF) is a natural polymer with biocompatibility and biodegradability. It is the main structural protein of silk secreted by several types of arthropods (Nguyen et al., Citation2019). The SF derived from silkworm (Bombyx mori) cocoons has become a candidate for biomedical applications because of its abundant yields and unique material properties (Huang et al., Citation2018). B. mori SF (hereinafter referred to as ‘SF’) is an amphipathic protein composed of hydrophobic heavy chains (∼391 kDa), hydrophilic light chains (∼25 kDa), and the glycoprotein P25 (Huang et al., Citation2018). Amino-acid sequences in heavy and light chains (primary structure of SF) can form different secondary structures through hydrogen bonding, van der Waals forces or hydrophobic interactions (Nguyen et al., Citation2019). α-Helical and random-coil forms allow SF to be part of drug-delivery systems in water-based solutions without organic solvents (Kundu et al., Citation2013). Under inductive conditions, the β-sheet structure formed by highly repeated hexapeptide GAGAGS motifs in the hydrophobic chain provides the maneuverable mechanical strength and release kinetics of SF (Holland et al., Citation2019). SF with these properties has been considered to be a preferred natural polymer for the preparation of NPs or MNs (Pham & Tiyaboonchai, Citation2020; Qi et al., Citation2021; Ulloa Rojas et al., Citation2022; Wang et al., Citation2022). However, SF has not been used to prepare NPs-MNs as a polymer for NPs and MN tips. Yavuz et al. prepared MNs loaded with SF particles using SF as a tip polymer for the delivery of levonorgestrel. Phase separation was exploited to prepare particles (∼14.42 μm) in which the use of reagents such as poly(vinyl alcohol) led to residual organic solvent and further complex processes, which are not conducive to industrialization of the process (Yavuz et al., Citation2020).
Herein, we developed SF-based MNs for delivery of triptorelin NPs to increase the relative bioavailability and improve tolerability of triptorelin for patients undergoing ART (). For the first time, SF was used as the polymer material for NPs and the support material for MNs without the addition of other polymers. The water-based processing properties of SF were exploited to prepare triptorelin-loaded NPs, avoiding the incorporation of organic reagents and inorganic salts. The stability of NPs in rat-skin homogenates was determined to evaluate the protective effect of NPs on triptorelin against enzymatic degradation. NPs-loaded tips prepared using a casting method were supported on a soluble polyacrylic acid (PAA) backing, which resulted in the tips being implanted subcutaneously to release NPs after NPs-MNs application (Lu et al., Citation2022). NPs-MNs were characterized (conformational changes, mechanical properties, in vitro transdermal release, pharmacokinetic and pharmacodynamic studies) to assess the potential of reducing the dose and frequency of administration in ART by improving relative bioavailability.
2. Materials and methods
2.1. Materials
SF (molecular weight ∼100 kDa) was obtained from Simatech (Suzhou, China). Triptorelin acetate (hereafter termed ‘triptorelin’) was purchased from Bachem (Bubendorf, Switzerland). PAA (molecular weight ∼250 kDa, 35 wt.%) and lyophilized powder of aprotinin (from bovine lung, 3–8 TIU/mg solid) were sourced from MilliporeSigma (Burlington, MA). Methanol (HPLC grade) was purchased from Merck (Whitehouse Station, NJ). Ultrapure water was produced by the Milli-Q® IQ7000 purification system (Millipore, Bedford, MA).
The mouse fibroblast cell line L929 was provided by the Luye Pharma Group (Yantai, China). Female Sprague–Dawley rats (6 weeks, 200 g ± 20 g) were purchased from Pengyue Laboratory Animal Breeding (Jinan, China). Experiments were approved by the Animal Ethical and Welfare Committee of Yantai University and were in accordance with the guidelines for the use of experimental animals set by the European Union.
2.2. Preparation of triptorelin NPs
The SF solution (6% w/v) was prepared and stirred continuously for 30 min at 300 rpm using a magnetic stirrer (RO15; IKA, Staufen, Germany). An equal volume of triptorelin solution was injected into SF solution at a constant speed. The mixture was frozen at −20 °C for 2 h and centrifuged at 10,000 rpm for 30 min at room temperature. Then, NPs were redispersed in water and stored at 4 °C (Lammel et al., Citation2010; Zhang et al., Citation2019).
2.3. Optimization of the concentration of triptorelin solution
To optimize the physical stability of triptorelin NPs, different triptorelin concentrations (1, 2, 3, 4, and 5 mg/mL) were tested for SF solution. Parallel experiments were undertaken with formulations not containing triptorelin. The mean particle size of NPs prepared with different triptorelin concentrations was analyzed using a Zetasizer (Nano-zs90; Malvern Instruments, Malvern, UK). The accelerated stability testing of NPs was undertaken using a dispersion analyzer (LUMiSize 651; LUM, Berlin, Germany) (Zielińska et al., Citation2018; Collado-González et al., Citation2020). NPs were added at a specified height in the sample cell and submitted to a rotor speed of 4000 rpm at 25 °C. The instability index was calculated by SEPView® software (Detloff et al., Citation2013).
2.4. Morphology and encapsulation efficiency of NPs
The morphology of NPs was observed via transmission electron microscopy (TEM) using a JEM-1400 system (JEOL, Tokyo, Japan). The formulation was dripped onto a copper net coated with a carbon film. To determine the efficiency of triptorelin encapsulation, liquid chromatography–tandem mass spectrometry (LC–MS/MS) using a LC-30AD system (Shimadzu, Kyoto, Japan) coupled with a Triple Quad™ 5500 setup (AB Sciex, Framingham, MA) was undertaken to quantify the concentration of the free drug in the supernatant of preparations after centrifugation and purification (Han et al., Citation2014). The encapsulation efficiency of NPs was calculated using the following equation:
(1)
(1)
2.5. In vitro release of triptorelin from NPs
Equal volumes of triptorelin solution and NPs containing an identical amount of drug were transferred to dialysis bags (molecular weight cutoff = 10 kDa) immersed in phosphate-buffered saline (PBS; pH 7.4) at 37 °C under constant agitation (100 rpm). Released medium (500 μL) was collected at 5 and 30 min, as well as 1, 2, 4, 8, 24, 48, and 72 h, and replaced with a fresh PBS (pH 7.4). Several samples were quantified using LC–MS/MS (as described above) to calculate the cumulative released percentage of the drug using the following equation:
(2)
(2)
where Ci is the triptorelin concentration in PBS, i is the sampling time, Vi is the sampling volume, and W is the total amount of triptorelin in the formulation.
2.6. In vitro stability study with skin-tissue homogenates
Skin tissue from the back of Sprague–Dawley rats was removed and homogenized after addition of five times the volume of PBS (pH 7.4) (Ito et al., Citation2011). Each tissue homogenate was centrifuged at 3000 rpm for 15 min at 4 °C. Subsequently, the supernatant was collected and stored at −80 °C. The tissue supernatant was mixed with triptorelin solution and NPs at a 9:1 volume ratio and incubation undertaken at 37 °C. Samples were removed at 0, 5, and 30 min, as well as 1, 2, 4, and 6 h. Aprotinin and methanol were added to terminate the reaction. The triptorelin concentration in supernatants incubated at various times was determined using LC–MS/MS to calculate the percentage of remaining drug.
2.7. Fabrication of triptorelin NPs-MNs
The NPs-MNs were manufactured using a twin-casting method, as described previously (Lu et al., Citation2022). NPs were added to a polydimethylsiloxane (PDMS) mold (10 × 10 array) and centrifuged into the tip groove. After drying and formation, PAA solution was added and centrifugation was undertaken again. Both centrifugations were carried out at 2800 rpm for 30 min at 10 °C using a centrifuge (5810R; Eppendorf, Hamburg, Germany). Then, the NPs-MNs were dried for 72 h at 25 °C, then demolded and stored in a dehumidifier. Stereographic microscopy using a SZN71 system (Sunny Optical Technology, Beijing, China) was undertaken to observe the morphology of NPs-MNs. Scanning electron microscopy (SEM) using an XL G2 setup (Phenom Scientific, Amsterdam, The Netherlands) was employed to observe the fine structure of individual MNs.
2.8. Fourier-transform infrared spectroscopy of NPs-MNs
The NPs-MNs without PAA were prepared for Fourier-transform infrared (FTIR) spectroscopy using an infrared spectrophotometer (Nicolet IS10; Thermo Scientific, Waltham, MA). NPs-MNs were ground and laminated with KBr disks. Samples were analyzed under the following conditions: scan range = 400–4000 cm−1; scan time = 128 s; resolution = 4 cm−1. Secondary-structure content was assessed using PeakFit 4.12 (SeaSolve, Murrieta, CA) to resolve the amide-I region (1595–1705 cm−1) in the infrared spectrum (Yin et al., Citation2018). Peak absorption bands (in cm−1) were assigned as follows: 1605–1615 as tyrosine side chains; 1616–1637 and 1696–1703 as β-sheet structure; 1638–1655 as random-coil structure; 1656–1662 as alpha-helical bands; 1663–1696 as β-turns (Hu et al., Citation2006).
2.9. Mechanical characteristics of NPs-MNs
The mechanical characteristics of NPs-MNs were evaluated by a texture analyzer (TA-XT PLUS; Stable Micro Systems, Godalming, UK) in compression mode. The sensor probe of the analyzer was pressed against vertically placed NPs-MNs at 0.1 mm/s. When the trigger force reached 5 g, the curve of force versus displacement was recorded until the probe moved downward 0.4 mm from the tip of MNs. The initial distance from the probe and the tip of MNs was 5 mm.
The insertion properties of NPs-MNs were investigated using skin collected from Bama miniature pigs. NPs-MNs were pierced vertically into the skin by a spring-loaded applicator and removed immediately (He et al., Citation2018). The skin was stained using methylene blue solution and imaged via a stereomicroscope (SZN71; Sunny Optical Technology, Yuyao, China).
2.10. Drug encapsulation and transdermal release of NPs-MNs in vitro
The drug encapsulation of NPs-MNs was determined using a method based on the thermal dissolution of lithium bromide (Lu et al., Citation2022). Briefly, NPs-MNs were dissolved in a solution of lithium bromide (9.3 M) at 60 °C and centrifuged at 3000 rpm for 5 min at room temperature. The supernatant obtained was then analyzed using LC–MS/MS to ascertain the triptorelin content.
Transdermal release of triptorelin from the skin collected from Bama miniature pigs was conducted in vitro using modified Franz cells. The skin model was pretreated for 1 h in PBS (pH 7.4) and immobilized between the donor compartment and recipient compartment of the Franz cell (Qiu et al., Citation2016). NPs-MNs were inserted into the skin for 3 min using an applicator. The matrix was then removed to allow needle-tip implantation into the skin. PBS (pH 7.4) was injected slowly as a release medium into the receptor chamber, and then the donor chamber was sealed using sealing film. The stirrer bar within the diffusion cell was rotated at 600 rpm in the release medium, which was maintained at 32 °C. Samples were collected at 30 min, as well as 1, 2, 4, 8, 24, and 48 h, and replaced with fresh PBS (pH 7.4). The drug concentration in samples was determined using LC–MS/MS to calculate the cumulative percentage transdermal release according to EquationEquation (2)(2)
(2) .
2.11. Cytotoxicity of NPs-MNs in vitro
SF membranes of different concentrations were prepared to evaluate the cytotoxicity of materials used in NPs-MNs. SF solutions (4%, 6%, 8%, 10%, and 12% (w/v)) were prepared, placed in 96-well plates, and dried for 72 h at 25 °C. A mouse fibroblast cell line (L929) was cultured in RPMI 1640 medium (Gibco, Billings, MT) supplemented with 10% (v/v) fetal bovine serum and 1% (v/v) penicillin–streptomycin. L929 cells in the logarithmic growth phase were seeded in a 96-well plate, covered with SF membrane at 20,000 cells/well, and three parallel wells were setup for each group. Then, samples were incubated for 24 h or 48 h. MTT solution (20 μL; 5 mg/mL; Aladdin, Shanghai, China) was added, and the cells were incubated for 4 h. After rinsing cells with PBS thrice, dimethyl sulfoxide (200 μL) was added and the culture was agitated for 10 min. The optical density (OD) of each well was measured at 570 nm using Cytation 5 (BioTek, Winooski, VT). Cell viability was calculated according to the following equation using the OD of the well without a membrane as a control.
(3)
(3)
2.12. In vivo study of NPs-MNs
Six-week-old female Sprague–Dawley rats with similar physiological cycles were screened as animal models and divided randomly into two groups of eight. One group of rats was injected with triptorelin solution (9 μg/kg, s.c.) as a control. Another group of rats had their back shaved and were administered NPs-MNs (9 μg/kg). The substrate of NPs-MNs was removed after being pressed with a finger for 3 min. Blood from the intraocular orbital plexus was collected into heparinized centrifuge tubes. The drug was administered 0.5, 1, 2, 4, 8, 24, and 48 h after blood collection. Plasma samples were obtained by centrifugation and stored immediately at −80 °C until analyses. The triptorelin concentration in samples was determined using LC–MS/MS, and pharmacodynamics (levels of LH and E2) were analyzed by an enzyme-linked immunosorbent assay kit (Elabscience Biotechnology, Beijing, China) following manufacturer protocols.
2.13. Statistical analyses
Data are the mean ± SD and were documented using Excel® 2019 (Microsoft, Redmond, WA). Statistical analyses were undertaken using a two-tailed Student’s t-test or analysis of variance via Origin (OriginLab, Northampton, MA). p < .05 was considered significant.
3. Results and discussion
3.1. Optimization of the concentration of triptorelin solution
In previous studies, we developed SF-based, long-acting MNs for sustained transdermal delivery of triptorelin to meet the needs of clinical medications used in the long term (Lu et al., Citation2022). However, the potential toxicity of long-acting preparations in the first trimester precludes their use in ART regimens. NPs-MNs were studied to meet the drug-delivery needs (safety, efficacy, and convenience) of pregnant women. The increased content of β-sheets in SF contributes to NP formation. Usually, salts and organic solvents are employed to induce changes in the secondary structure of SF to prepare NPs (Pham & Tiyaboonchai, Citation2020). Unfortunately, complicated washing steps to remove unreacted reagents hamper industrial production. Compared with the infrared spectra of SF-MNs without triptorelin incorporation, triptorelin incorporation increased the β-sheet content of SF (Lu et al., Citation2022). Drug–SF interactions and gentle shear forces were exploited to develop methods for preparing NPs that avoided the use of organic solvents or inorganic salts, providing potential for industrial scale-up.
The concentration of triptorelin solution (a key parameter in the process) was optimized based on the particle size and instability index. NPs increased in size with an increasing concentration of triptorelin (). Although the mean particle size of SF micelles without triptorelin was the smallest (∼160 nm), the particle-size dispersion index was >0.4. When the concentration of triptorelin solution added was >3 mg/mL, the mean particle size of the nano-system showed a double peak. The obvious aggregation of particles was not conducive to the physical stability of the nano-system. The mean particle size of NPs was optimal at 200.26 ± 6.35 nm (polydispersity index: 0.199 ± 0.035) when prepared with a triptorelin solution at 2 mg/mL. The accelerated stability upon centrifugation of NPs was measured by a displacement analyzer. Three hundred profiles were recorded every 10 s at a measurement wavelength of 870 nm (). The change between the initial measurement curve (red) and final measurement curve (green) was large, thereby indicating that the sample was unstable. The calculated instability index of NPs prepared with 2 mg/mL triptorelin solution was the lowest (0.26 ± 0.035), which indicated good physical stability. NPs with good physical stability were prepared after formulation optimization to ensure that the process of being filled into molds to form MNs was reproducible.
Figure 2. Characterization of triptorelin nanoparticles (NPs). (A) Particle size and (B) instability index of NPs produced with different concentrations of triptorelin. (C) Typical transmission electron microscopy (TEM) images of NPs. (D) In vitro release of triptorelin solution and NPs in PBS (pH 7.4). (E) Stability of triptorelin solutions and NPs in homogenates of rat-skin tissue.

3.2. Morphology and encapsulation efficiency of NPs
The NPs were prepared using optimal process parameters and characterized in terms of essential physicochemical properties, including morphology and encapsulation efficiency. The TEM images in reveal the NPs obtained using an optimal process had a monodisperse spherical shape. The particle size of NPs (∼200 nm) was consistent with the results determined using the Zetasizer. Measurement of the amount of free drug in supernatants revealed triptorelin to be encapsulated in NPs with a high encapsulation efficiency of 97.23% ± 1.95%. The optimized low mass ratio of drug:SF (1:30) resulted in a high encapsulation efficiency of NPs, meeting the requirements of animal experiments and the design of MN size for a human daily dose. The prepared NPs with physical stability and high encapsulation ensured the morphology and drug loading of NPs-MNs.
3.3. In vitro release of triptorelin from NPs
Triptorelin release from NPs in vitro was determined at different time intervals in PBS (pH 7.4). As a control, triptorelin solution was infiltrated rapidly into the release medium and cumulative drug release reached 83.70% ± 3.22% in 2 h (). Triptorelin release from NPs increased slowly to ∼63% over 48 h until 72 h, thereby demonstrating a sustained-release effect of NPs. Simultaneously, ∼20% of triptorelin from NPs was released into the release medium within 2 h, thereby ensuring a flare-up effect and potential desensitization of the pituitary gland. The cumulative release of NPs reached a plateau at 48–72 h, indicating that the drug could not be released completely from NPs using PBS as the release medium. During NP preparation, the β-sheet content of SF increased, thereby resulting in the formation of domains of high crystallinity, which hindered the diffusion of drugs. Due to the slow degradation of insoluble SF in PBS, the drug could not be released completely within 72 h (Numata & Kaplan, Citation2010; Hines & Kaplan, Citation2011).
3.4. In vitro stability study with skin-tissue homogenates
The in vitro stability of NPs using homogenates of rat-skin tissues was tested to verify the protection of NPs against the degradation of triptorelin by enzymes. Triptorelin solution was rapidly hydrolyzed enzymatically in skin-tissue fluid. The percentage of degraded triptorelin within 5 min was 26.16% ± 5.74% (). The remaining triptorelin in the tissue fluid at 6 h could not be quantified by LC–MS/MS (lower limit of quantification: 0.01 ng/mL). Triptorelin in NPs was degraded by only 12.89% ± 4.87% within 1 h and the amount of triptorelin remaining at 6 h was ∼26%. Hence, NPs served as ‘shells’ to protect triptorelin from enzymatic attack.
3.5. Fabrication of triptorelin NPs-MNs
The NPs-MNs were fabricated using a micro-mold method for transdermal delivery of triptorelin NPs. NPs with an SF concentration of 6% (w/v) in the mold were dried and molded into a needle tip without the use of other polymers. This simplified and scalable preparation process avoids interactions between different polymers and drugs, thereby facilitating the stability of the drugs in MNs. The NPs-MNs were observed (by stereomicroscopy) to consist of a single conical needle of height ∼600 μm and base width ∼300 μm (). To observe the fine structure of NPs-MNs, blank microneedles (BK-MNs) without triptorelin were prepared using the same process as the control. Single MNs with or without triptorelin were observed without significant differences under SEM (). However, the cut surface of NPs-MNs was more grainy compared with that of BK-MNs, which may have been related to the change in the secondary structure of SF during NP formation.
3.6. FTIR spectroscopy of NPs-MNs
To assess the conformation and aggregated structure of NPs-MNs, FTIR spectroscopy was undertaken using PeakFit 4.12. Compared with BK-MNs, the amide-I region of NPs-MNs in the infrared spectrum was blue-shifted toward 1620 cm−1 (β-sheet region) (). The β-sheet content in the secondary structure of NPs-MNs increased to 19.4% ± 1.78%, whereas the content of α-helix/random coils decreased. These data indicated that the insoluble crystalline domains in the secondary structure of SF increased and the amorphous matrix decreased during NP formation. A high degree of ordering and high density of β-sheet are beneficial to enhancement of the mechanical properties of NPs-MNs and the sustained release of drugs (Nguyen et al., Citation2019). Unlike previous studies (Lu et al., Citation2022), there was no significant change in the content of β-turns in the conformation of NPs-MNs, possibly related to the different induction conditions of secondary-structure changes (Hu et al., Citation2006). The side-chain fraction in amide I was stable (∼2.6%).
3.7. Mechanical characteristics of NPs-MNs
To investigate the mechanical characteristics of NPs-MNs, the fracture strength was measured with a mechanical-test setup (). The BK-MNs were more likely to be pressed down by the probe than the NPs-MNs under identical measurement conditions (). A failure force of NPs-MNs >100 mN/MN could be inserted into skin by measurement with a texture analyzer (Raja et al., Citation2013), whereas BK-MNs failed as the probe pressure reached ∼50 mN (). NP formation increased the content of β-sheets in the secondary structure, thereby contributing to the mechanical properties of NPs-MNs (Chen et al., Citation2019).
Figure 5. Mechanical characteristics of nanoparticles-encapsulated polymeric microneedles (NPs-MNs). Mechanical-test setup (schematic) (A). Optical images of NPs-MNs before and after mechanical testing (B). Mechanical strength of all NPs-MNs (n = 6, *p < .05) (C). Single-needle mechanical test of blank microneedles (BK-MNs) and NPs-MNs (D). Optical images of porcine skin after puncture with BK-MNs (I) and NPs-MNs (II) (E).

The micro-conduits produced by MNs in porcine skin were visualized using methylene blue to verify the skin insertion of NPs-MNs. A complete array of blue spots (10 × 10) corresponding to the puncture sites of NPs-MNs were displayed on the skin surface (-II)), indicating that all MNs had been inserted into the skin. However, BK-MNs could not be inserted completely into the skin, consistent with the results of fracture-force determination ().
3.8. Drug encapsulation and transdermal release of NPs-MNs in vitro
Drug encapsulation in NPs-MNs (10 × 10 array) was determined to be 1.75 ± 0.16 μg, which was sufficient to deliver a daily therapeutic dose of triptorelin for in vivo experiments. Transdermal release of triptorelin from NPs-MNs into the diffusion cell was monitored for 48 h with MNs pierced into porcine skin (). Triptorelin barely penetrates into skin because it is a water-soluble macromolecular drug. Approximately, 65% of triptorelin in NPs-MNs was released into PBS of the receptor chamber within 24 h, indicating that the NPs-MNs piercing the stratum corneum improved the skin permeability of triptorelin. A possible reason for the incomplete transdermal release of triptorelin into the receiving chamber was the retention of triptorelin in porcine skin due to a lack of microcirculation in the skin ex vivo.
3.9. In vitro cytotoxicity of NPs-MNs
In vitro cytotoxicity was evaluated by analyzing the effect of drug-free SF membranes on the viability of L929 cells. The morphology of L929 cells in SF membrane-coated 96-well plates was observed using Cytation (). The cell viability of each group incubated for 24 h or 48 h was calculated to be ∼98% (). Cell viability was not dependent upon the SF concentration, thereby indicating that SF membranes did not have an obvious toxic effect on L929 cells.
Figure 7. Safety and in vivo studies of nanoparticles-encapsulated polymeric microneedles (NPs-MNs). Morphology of L929 cells in a 96-well plate covered with silk fibroin (SF) membrane (a). Cell viability of L929 cells incubated for 24 h or 48 h in a 96-well plate covered with SF membrane. (B). Images of rat skin after application of nanoparticles-encapsulated polymeric microneedles (NPs-MNs) (I), immediately (II) and 1 d (III) after removal of the MNs base (C). Mean plasma concentration–time profiles for triptorelin in rats administered NPs-MNs (9 μg/kg) and injected with triptorelin (9 μg/kg, s.c.) (n = 8). (D). Plasma concentration for luteinizing hormone (LH) (E) and estradiol (E2) (F) in rats administered NPs-MNs and injected (s.c.) with triptorelin (n = 8).

3.10. In vivo study of NPs-MNs
To investigate the in vivo behavior of NPs-MNs in female rats, the triptorelin concentration in plasma at different time intervals after administration was determined using LC–MS/MS. Obvious edema and erythema did not appear on rat skin administered with NPs-MNs, and the micropores and indentations caused by MNs healed within 1 d (). The plasma concentration of triptorelin in both groups reached a peak at 0.5 h, and then decreased gradually (). The triptorelin concentration in plasma could not be detected at 24 h after subcutaneous injection. However, triptorelin was released continuously from MNs into the plasma of NPs-MNs-treated rats until 48 h. Pharmacokinetic parameters were calculated using Phoenix Winnolin 6.3 (Certara, Princeton, NJ) and are listed in . The half-life of triptorelin encapsulated in NPs-MNs was prolonged and the AUC0–t was increased in vivo compared with subcutaneous injection. The relative bioavailability (Frel) of NPs-MNs was calculated using the following equation:
(4)
(4)
where AUC represents the area under the plasma concentration–time curve, subscripts T and R represent NPs-MNs and the reference formulation for subcutaneous injection, respectively, and D represents the dose administered.
Table 1. Pharmacokinetic parameters of triptorelin in rats administered NPs-MNs (9 μg/kg) and injected with triptorelin (9 μg/kg, s.c.) (n = 8).
The relative bioavailability of NPs-MNs was increased to 184.52% ± 0.17%, indicating that the formulation may reduce the dose required for administration in women preparing for pregnancy.
Plasma levels of LH and E2 were determined as pharmacodynamic parameters by enzyme-linked immunosorbent assays. The LH concentration in plasma in both groups of rats reached a peak at 0.5 h, and then continued to decline (). The inhibitory effect of NPs-MNs upon LH could be maintained for 48 h, whereas the plasma LH levels in rats of the subcutaneous-injection group returned to a normal physiological state at 48 h. Similarly, the inhibitory effect of NPs-MNs on E2 was also prolonged to 48 h compared with the subcutaneous-injection group (). However, the plasma E2 levels in NPs-MNs-treated rats peaked at 4 h, whereas the plasma E2 levels in the subcutaneous-injection group rats peaked at 2 h. The different effect of triptorelin in increasing E2 secretion between the two formulations may have been due to the sustained-release effect of NPs-MNs on the drug. Administration of NPs-MNs initially increased the secretion of LH and E2 into the plasma of female rats, followed by prolonged inhibition of secretion of LH and E2. These data suggested that NPs-MNs could provide a strategy to improve the drug tolerance of patients in the clinical application of ART.
Healthy animal models have been employed to evaluate the pharmacokinetics and pharmacodynamics of triptorelin as a highly active GnRHa (Asmus et al., Citation2013; Han et al., Citation2014; Varamini et al., Citation2017). To avoid the influence of the estrous cycle of female animals on sex hormones, rats with the same physiological cycle were selected to evaluate the regulatory effect of NPs-MNs on LH and E2 at 48 h. However, because of the effects of various factors (e.g. stress and mood) on estrogen, the daily application of NPs-MNs in 15-day regimens requires more stringent feeding conditions (even from birth) to screen female rats to obtain pharmacodynamic indices. Long-cycle pharmacodynamic evaluation of many samples will be carried out in future studies.
4. Conclusions
SF-based NPs-MNs were developed to deliver triptorelin for use in ART. Simple water-based treatment and drug incorporation methods were used to prepare SF-NPs. The nanoencapsulation avoided the degradation of triptorelin by peptidases in skin-tissue fluid. NPs-MNs with good mechanical properties delivered triptorelin via the transdermal route and increased the relative bioavailability of triptorelin. Pharmacodynamic studies revealed that the NPs-MNs exerted regulatory effects on the secretion of LH and E2 and prolonged the downregulation of their secretion. MN-facilitated delivery of triptorelin NPs holds promise for industrial scale-up and broad application in ART.
Author contributions
Xiaoyan Lu: conceptualization, methodology, investigation, writing – original draft writing – original draft, and writing – review and editing; Yiying Sun: software, resources, and writing – original draft; Meishan Han: formal analysis, investigation, and writing – original draft; Daoyuan Chen: investigation, methodology, and data curation; Xiaoyan He: project administration and data curation; Siqi Wang: methodology, investigation, and data curation; Kaoxiang Sun: conceptualization, writing – review and editing, supervision, project administration, and funding acquisition.
Ethical approval
All animal experiments were approved by the Animal Ethical and Welfare Committee of Yantai University (YTU-2012010) and were in accordance with the guidelines for the use of experimental animals set by the European Union. All animals were raised at constant temperature (25 ± 2 °C) and humidity (55 ± 5%) and fed with commercial aseptic food. All animals were employed to evaluate the therapeutic effects of triptorelin nanoparticle-loaded microneedles and every effort was made to minimize animal suffering and reduce the number of animals used in the experiments. The study has adhered to the ARRIVE (Animal Research: Reporting of In Vivo Experiments) guidelines.
Supplemental Material
Download MS Word (16.1 KB)Disclosure statement
The authors declare that they have no known competing financial interests or personal relationships that could have appeared to influence the work reported in this paper.
Data availability statement
All data and materials of this study can be obtained from the corresponding author upon reasonable request.
Additional information
Funding
References
- Adamson G, Dyer S, Chambers G, et al. (2019). ICMART preliminary world report 2015. Hum Reprod 37:1.
- Asmus LR, Tille JC, Kaufmann B, et al. (2013). In vivo biocompatibility, sustained-release and stability of triptorelin formulations based on a liquid, degradable polymer. J Control Release 165:199–11. doi: 10.1016/j.jconrel.2012.11.014.
- Bethesda M. (2012). Clinical and research information on drug-induced liver injury-triptorelin. In: LiverTox. Available at: https://www.ncbi.nlm.nih.gov/books/NBK548756/.
- Chen S, Liu M, Huang H, et al. (2019). Mechanical properties of Bombyx mori silkworm silk fibre and its corresponding silk fibroin filament: a comparative study. Mater Des 181:108077. doi: 10.1016/j.matdes.2019.108077.
- Collado-González M, Ferreri MC, Freitas AR, et al. (2020). Complex polysaccharide-based nanocomposites for oral insulin delivery. Mar Drugs 18:55. doi: 10.3390/md18010055.
- DeMuth PC, Moon JJ, Suh H, et al. (2012). Releasable layer-by-layer assembly of stabilized lipid nanocapsules on microneedles for enhanced transcutaneous vaccine delivery. ACS Nano 6:8041–51. doi: 10.1021/nn302639r.
- Detloff T, Sobisch D, Lerche JDLT. (2013). Instability index. Dispers Lett Techn 4:1–4.
- Eftekhar M, Rahmani E, Mohammadian F. (2013). Comparison of pregnancy outcome in half-dose triptorelin and short-acting decapeptyl in long protocol in ART cycles: a randomized clinical trial. Iran J Reprod Med 11:133–8.
- Fields E,Chard J,James D,Treasure T. (2013). Fertility (update): summary of NICE guidance. BMJ 346:f650. doi: 10.1136/bmj.f650.
- Han J, Zhang S, Liu W, et al. (2014). An analytical strategy to characterize the pharmacokinetics and pharmacodynamics of triptorelin in rats based on simultaneous LC–MS/MS analysis of triptorelin and endogenous testosterone in rat plasma. Anal Bioanal Chem 406:2457–65. doi: 10.1007/s00216-014-7616-z.
- He M, Yang G, Zhang S, et al. (2018). Dissolving microneedles loaded with etonogestrel microcrystal particles for intradermal sustained delivery. J Pharm Sci 107:1037–45. doi: 10.1016/j.xphs.2017.11.013.
- Hines DJ, Kaplan DL. (2011). Mechanisms of controlled release from silk fibroin films. Biomacromolecules 12:804–12. doi: 10.1021/bm101421r.
- Holland C, Numata K, Rnjak-Kovacina J, Seib FP. (2019). The biomedical use of silk: past, present, future. Adv Healthc Mater 8:e1800465. doi: 10.1002/adhm.201800465.
- Hu X, Kaplan D, Cebe P. (2006). Determining beta-sheet crystallinity in fibrous proteins by thermal analysis and infrared spectroscopy. Macromolecules 39:6161–70. doi: 10.1021/ma0610109.
- Huang W, Ling S, Li C, et al. (2018). Silkworm silk-based materials and devices generated using bio-nanotechnology. Chem Soc Rev 47:6486–504. doi: 10.1039/c8cs00187a.
- Ingrole RSJ, Azizoglu E, Dul M, et al. (2021). Trends of microneedle technology in the scientific literature, patents, clinical trials and internet activity. Biomaterials 267:120491. doi: 10.1016/j.biomaterials.2020.120491.
- Ito Y, Murano H, Hamasaki N, et al. (2011). Incidence of low bioavailability of leuprolide acetate after percutaneous administration to rats by dissolving microneedles. Int J Pharm 407:126–31. doi: 10.1016/j.ijpharm.2011.01.039.
- Kundu B, Rajkhowa R, Kundu SC, Wang X. (2013). Silk fibroin biomaterials for tissue regenerations. Adv Drug Deliv Rev 65:457–70. doi: 10.1016/j.addr.2012.09.043.
- Lammel AS, Hu X, Park SH, et al. (2010). Controlling silk fibroin particle features for drug delivery. Biomaterials 31:4583–91. doi: 10.1016/j.biomaterials.2010.02.024.
- Lan X, She J, Lin DA, et al. (2018). Microneedle-mediated delivery of lipid-coated cisplatin nanoparticles for efficient and safe cancer therapy. ACS Appl Mater Interfaces 10:33060–9. doi: 10.1021/acsami.8b12926.
- Larrañeta E, Lutton REM, Woolfson AD, Donnelly RF. (2016). Microneedle arrays as transdermal and intradermal drug delivery systems: materials science, manufacture and commercial development. Mater Sci Eng R Rep 104:1–32. doi: 10.1016/j.mser.2016.03.001.
- Lin S, Quan G, Hou A, et al. (2019). Strategy for hypertrophic scar therapy: improved delivery of triamcinolone acetonide using mechanically robust tip-concentrated dissolving microneedle array. J Control Release 306:69–82. doi: 10.1016/j.jconrel.2019.05.038.
- Lu X, Sun Y, Han M, et al. (2022). Silk fibroin double-layer microneedles for the encapsulation and controlled release of triptorelin. Int J Pharm 613:121433. doi: 10.1016/j.ijpharm.2021.121433.
- Nguyen TP, Nguyen QV, Nguyen VH, et al. (2019). Silk fibroin-based biomaterials for biomedical applications: a review. Polymers 11:1933. doi: 10.3390/polym11121933.
- Numata K, Kaplan DL. (2010). Silk-based delivery systems of bioactive molecules. Adv Drug Deliv Rev 62:1497–508. doi: 10.1016/j.addr.2010.03.009.
- Permana AD, Tekko IA, McCrudden MTC, et al. (2019). Solid lipid nanoparticle-based dissolving microneedles: a promising intradermal lymph targeting drug delivery system with potential for enhanced treatment of lymphatic filariasis. J Control Release 316:34–52. doi: 10.1016/j.jconrel.2019.10.004.
- Pham DT, Tiyaboonchai W. (2020). Fibroin nanoparticles: a promising drug delivery system. Drug Deliv 27:431–48. doi: 10.1080/10717544.2020.1736208.
- Qi Z, Cao J, Tao X, et al. (2021). Silk fibroin microneedle patches for the treatment of insomnia. Pharmaceutics 13:2198. doi: 10.3390/pharmaceutics13122198.
- Qiu Y, Li C, Zhang S, et al. (2016). Systemic delivery of artemether by dissolving microneedles. Int J Pharm 508:1–9. doi: 10.1016/j.ijpharm.2016.05.006.
- Raja WK, MacCorkle S, Diwan IM, et al. (2013). Transdermal delivery devices: fabrication, mechanics and drug release from silk. Small 9:3704–13. doi: 10.1002/smll.201202075.
- Sabri AH, Kim Y, Marlow M, et al. (2020). Intradermal and transdermal drug delivery using microneedles – fabrication, performance evaluation and application to lymphatic delivery. Adv Drug Deliv Rev 153:195–215. doi: 10.1016/j.addr.2019.10.004.
- Singh P, Carrier A, Chen Y, et al. (2019). Polymeric microneedles for controlled transdermal drug delivery. J Control Release 315:97–113. doi: 10.1016/j.jconrel.2019.10.022.
- Siristatidis CS, Gibreel A, Basios G, et al. (2015). Gonadotrophin-releasing hormone agonist protocols for pituitary suppression in assisted reproduction. Cochrane Database Syst Rev 2015:CD006919.
- Suchaoin W, Bernkop-Schnürch A. (2017). Nanocarriers protecting toward an intestinal pre-uptake metabolism. Nanomedicine 12:255–69. doi: 10.2217/nnm-2016-0331.
- Ulloa Rojas JE, Oliveira VL, de Araujo DR, et al. (2022). Silk fibroin/poly(vinyl alcohol) microneedles as carriers for the delivery of singlet oxygen photosensitizers. ACS Biomater Sci Eng 8:128–39. doi: 10.1021/acsbiomaterials.1c00913.
- Varamini P, Rafiee A, Giddam AK, et al. (2017). Development of new gonadotropin-releasing hormone-modified dendrimer platforms with direct antiproliferative and gonadotropin releasing activity. J Med Chem 60:8309–20. doi: 10.1021/acs.jmedchem.6b01771.
- Wang C, Ye Y, Hochu GM, et al. (2016). Enhanced cancer immunotherapy by microneedle patch-assisted delivery of anti-PD1 antibody. Nano Lett 16:2334–40. doi: 10.1021/acs.nanolett.5b05030.
- Wang Z, Yang Z, Jiang J, et al. (2022). Silk microneedle patch capable of on-demand multidrug delivery to the brain for glioblastoma treatment. Adv Mater 34:e2106606. doi: 10.1002/adma.202106606.
- WHO. (2020). Infertility. Available at: https://www.who.int/news-room/fact-sheets/detail/infertility.
- Yavuz B, Chambre L, Harrington K, et al. (2020). Silk fibroin microneedle patches for the sustained release of levonorgestrel. ACS Appl Bio Mater 3:5375–82. doi: 10.1021/acsabm.0c00671.
- Yin Z, Kuang D, Wang S, et al. (2018). Swellable silk fibroin microneedles for transdermal drug delivery. Int J Biol Macromol 106:48–56. doi: 10.1016/j.ijbiomac.2017.07.178.
- Yu J, Zhang Y, Ye Y, et al. (2015). Microneedle-array patches loaded with hypoxia-sensitive vesicles provide fast glucose-responsive insulin delivery. Proc Natl Acad Sci U S A 112:8260–5. doi: 10.1073/pnas.1505405112.
- Zhang H, Lai L, Wang Y, et al. (2019). Silk fibroin for CpG oligodeoxynucleotide delivery. ACS Biomater Sci Eng 5:6082–8. doi: 10.1021/acsbiomaterials.9b01413.
- Zielińska A, Martins-Gomes C, Ferreira NR, et al. (2018). Anti-inflammatory and anti-cancer activity of citral: optimization of citral-loaded solid lipid nanoparticles (SLN) using experimental factorial design and LUMiSizer®. Int J Pharm 553:428–40. doi: 10.1016/j.ijpharm.2018.10.065.