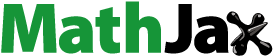
Abstract
Itraconazole (ICZ) was prepared in a self-microemulsifying (SM) gel. This gel was intended for use in the oral mucosa, where low volume and flow of saliva result in limited solubility and absorption of drugs that are poorly water-soluble. The drug-loaded gel formulation (ICZ-SM) was selected as a clear solution in the ternary phase diagram to improve the solubility of ICZ. Seven ratios (S1–S7) were prepared by mixing polyoxyl 35 castor oils (P35), a medium chain with a blend of mono-, di-, and triglycerides (MCT), and water. Phase separation of large-sized emulsions by countering with artificial saliva were observed in dilution tests for the formulation contained MCT, P35, and water at the ratios of 70:20:10 (S1), 10:80:10 (S3), and 20:60:20 (S4). Formulations in the ratios of 15:50:35 (S5) and 19:43:38 (S6) produced strong ICZ-SM gels, as shown by rheology tests, whereas the formulations at the ratios of 30:60:10 (S2) and 10:43:47 (S7) exhibited no elasticity. A model of zero-order kinetic (S5) and first-order kinetic (S6) were found to best fit the release kinetics of ICZ from the gels. Time-killing assays revealed that S5 and S6 required less time compared with S2 and the ICZ solution. Furthermore, S5 exhibited the highest increase in cell uptake compared with S2, S6, and the ICZ solution. These findings suggest that the ICZ-SM gel was a free polymer capable of delivering an ICZ for the treatment of oral candidiasis, and that ICZ-SM gels applied locally exhibit enhanced absorption into cells.
Introduction
Drugs are typically administered to the oral mucosa for the purpose of treating local infections and ulcers, or for systemic absorption (Sharma & Sharma, Citation2022). The pH of the oral cavity ranges from 5.6 to 7.9, and there is little enzymatic activity. The oral cavity area is walled by non-keratinized, stratified squamous epithelium (100–200 μm). One major limitation is the low volume and flow of saliva, which results in low solubility and absorption of a drug in the oral mucosa, particularly for drugs that are poorly water-soluble (Hua, Citation2019).
A solubilized form with sustained release of a drug may solve this problem, thus microemulsions may be applied. Surfactants/co-surfactants, oils, and water can be combined to create microemulsions with the concentration adjusted in the ternary phase diagram. There have been many studies using microemulsions for drug delivery through the skin (Said et al., Citation2017; Patel et al., Citation2021; Souto et al., Citation2022).
Celecoxib, dexibuprofen, or zaltoprofen microemulsions have been used for transdermal delivery to treat various inflammatory disorders (Shukla et al., Citation2018). The topical application of minoxidil-loaded microemulsion and nanoemulsion has been achieved (Abd et al., Citation2018). Microemulsions for transdermal use may diminish the side effects and increase drug permeation through the skin. In vitro permeability studies revealed that 90% of the drug is released continuously over the course of 24 hours. The Higuchi kinetic or zero-order model of released drug behavior provides a good explanation for the drug-release profile (Venugopal et al., Citation2023). To improve therapeutic efficacy and decrease first-past metabolism, a repaglinide-loaded microemulsion as a transdermal gel was produced (Aggarwal et al., Citation2013). Transdermal delivery avoided first-pass metabolism, the gel resulted in a controlled drug release over an extended period, and the microemulsion increased drug solubility. Microemulsions are stable on the surface of the body; however, once they enter the oral cavity, they may be continuously diluted with saliva, which impairs stability. The ability of microemulsions to be partially converted into nanoemulsions can increase drug bioavailability.
Previously, we developed a self-microemulsifying (SM) gel (polymer-free) by water titration, and monolaurin was incorporated into the formulation (Tubtimsri et al., Citation2022). Weak gel strength of the loaded monolaurin formulations was observed compared with the base formulation. In our preliminary studies, itraconazole (ICZ) was used as the active drug, and the strength of the gels was unchanged as determined by rheology. Therefore, ICZ was selected as a model drug for the present study. ICZ is a poorly water-soluble drug (1–4 ng/mL) with high membrane permeability (logP = 5.66), and it is classified as class II in the biopharmaceutical classification system (Triboandas et al., Citation2022). Reports list the bioavailability of the oral solution as approximately 55%; however, ICZ is very useful for the treatment of oral candidiasis that is resistant to fluconazole (Noël de Tilly & Tharmalingam, Citation2022). Therefore, we created a microemulsion gel formulation containing the poorly water-soluble drug (ICZ) for delivery to the oral mucosa to increase absorption and extend absorption time. The robustness of the sample dilution (size and size distribution) was determined to assess the self-emulsifying ability. A drug-release kinetic model was used to explain the profiles, and time-killing kinetics were established to compare the efficiency of each sample. An oral squamous cancer cell line (KON) was used to evaluate the absorption ability (Essawy et al., Citation2021). To assess the stability of the drug formulations and their physical properties, selected samples were stored for 3 or 6 months under ambient and accelerated conditions, and the properties were reevaluated.
Materials and methods
Materials
Itraconazole (ICZ) was purchased from Nosch Labs Private (Hyderabad, India). Polyoxyl 35 castor oil (P35) was obtained from BASF (Bangkok, Thailand). Caprylic and capric acid with a blend of mono-, di-, and triglycerides (MCT) were purchased from Sasol Germany GmbH (Hamburg, Germany). Candida albicans (DMST 5815) was obtained from the Department of Medical Sciences, Thailand. The culture media, Sabouraud dextrose broth (SDB), and Sabouraud dextrose agar (SDA) were purchased from Merck KGaA (Germany). The human oral squamous cell carcinoma KON (Lot NO. 01262007) derived from a cervical lymph node was acquired from the JCRB cell bank. Dulbecco’s Modified Eagle Medium (DMEM) was purchased from Corning (USA). All chemicals were of analytical grade.
Determination of ICZ solubility in a self-microemulsion (SM)
From our previous study (Tubtimsri et al., Citation2022), a dosage formulation for self-microemulsifying systems was established by titrating water into the oil phase (P35 and MCT) based on the construction of a ternary phase diagram. Seven mixture ratios distributed on the ternary phase diagram were selected by the appearance of a clear solution. Accurate weights of MCT and P35 were added to test tubes, and water was added. After shaking, a clear SM formed spontaneously. An excess amount of ICZ (100 mg) was added to 5 g of each mixture to determine the optimal solubility. The mixtures were vigorously shaken and incubated at 25 °C for 3 days. After centrifugation (3,500 rpm, 15 min), the supernatant was collected for ICZ content analysis by high-performance liquid chromatography (HPLC).
Preparation of ICZ-loaded SM (ICZ-SM)
Amounts of MCT, P35, and water were precisely weighed and placed into test tubes, and then ICZ powder was added. The test tube was then tightly closed, and the tube was vigorously shaken for 3 days at a constant temperature of 25 °C. The resulting clear ICZ-SM with various ratios of oil, surfactant, and water were used for further study.
ICZ content analysis
HPLC was used to determine (Weerapol et al., Citation2015) the ICZ content in the supernatant (Jasco model PU-2089 with a Jasco UV-2070 plus multi-wavelength UV–VIS detector, Jasco, Japan) using a Luna C18 column (5 μm, 4.6 × 250 mm) (Phenomenex®, USA). Acetonitrile:water:diethylamine was used as the mobile phase in a ratio of 63:37:0.05. The pH was adjusted to 2.45 with phosphoric acid, the flow rate was 1.0 mL/min, and the wavelength was 263 nm. The mobile phase was filtered through a 0.22 mm membrane and degassed using a sonicator prior to use.
Robustness of the diluted SM gel
To study the size of the emulsion when diluted with artificial saliva at various ratios (Jaiswal et al., Citation2014), the formulations (with and without ICZ) were measured after diluting with artificial saliva at ratios of 1:10, 1:50, and 1:100. Before photon correlation spectroscopy measurements (model Zetasizer Nano ZS, Malvern, England), the sample was allowed to stand for 30 min. The solution was artificial saliva consisting of 0.4 g/L NaCl, 0.5 g/L KCl, 0.6 g/L CaCl2, 1 g/L urea, and 0.69 g/L NaH2PO4. The pH was adjusted to 5.8 with hydrochloric acid.
Rheology of the ICZ-SM gels
To simulate the use of gels in the oral cavity, the storage modulus (G′) and loss modulus (G″) were measured. A rheometer (Kinexus Pro, Malvern Instrument, UK) was used to determine the rheological properties of the ICZ-SM gels. The amplitude sweep was studied at 25 °C and 37 °C. The frequency was fixed at 1 Hz, and the amplitude was varied from 0.1% to 100%. The amplitude or shear strain was obtained by examining the amplitude sweep, and the linear viscoelastic region (LVE) of each sample was determined by plotting the logarithmic correlation between the strain and modulus (Stojkov et al., Citation2021). The LVE region was selected for a certain shear strain value in this range to be used in subsequent flow behavior studies (Weerapol & Sriamornsak, Citation2020).
The temperature sweep was examined by setting a constant shear strain of 0.5%, a constant frequency of 1 Hz, and varying the temperature from 25 °C to 45 °C, which revealed the flow properties at various temperatures. The viscosity of the formulations was measured at 25 °C and 37 °C and analyzed by the power law. The viscosity was calculated according (Alves Barroso et al., Citation2022) to EquationEq. (1)(1)
(1) .
(1)
(1)
where
is the viscosity,
is the consistency coefficient,
is the shear rate, and
is the power law index.
Drug-release kinetics from the ICZ-SM gels
A flow-through cell apparatus (USP apparatus IV; CE7smart, Sotax AG, Zürich, Switzerland) was used to evaluate the in vitro release of ICZ from SM gels. A 5-mm ruby bead was positioned on top of a tablet cell (22.6 mm) in the flow-through cone of the cell, which was filled with 1 mm glass beads. The artificial saliva as dissolution medium (50 mL) was circulated at a flow rate of 8 mL/min. The temperature was set to 37 °C ± 0.5 °C, and the samples (5 mL) were collected at various time intervals and analyzed for ICZ content using HPLC. DDsolver (add-in program) was used to fit the model of the drug-release profiles for the selected formulations (Zhang et al., Citation2010).
Kinetics of time-killing by ICZ-SM gels
Time-kill kinetics were used to examine the therapeutic efficacy of the antifungal preparation. C. albicans was diluted to 1 × 104 CFU/mL after growing for 48 h at 37 °C in SDB. A culture tube was filled with 0.1 g of ICZ-SM gel, divided using a rotator, and gently stirred for 360 min at 37 °C and 200 rpm. Then, 100 µL of sample was periodically removed from each test tube and spread onto an SDA plate for 5, 15, 30, 60, 120, 240, and 360 min. Each plate was then incubated for an additional 48 h at 37 °C. ICZ solution (0.5 mg/g in polyethylene glycol (PEG) 200) and distilled water were used as positive and negative controls, respectively. The number of viable fungal colonies was counted at each time point (Latifah-Munirah et al., Citation2015; Cao et al., Citation2021). The area under the curve (AUC) of the viability curve was determined as the killing response to the ICZ-SM gels.
Evaluating the cellular uptake of ICZ-SM gels
The KON cell line, which is derived from human oral squamous cell carcinoma and originates from non-keratinocyte cells of the oral cavity, was used to study cellular uptake. DMEM (0.01% v/v L-glutamine solution and 10% v/v fetal bovine serum) were used as a medium in cell culture. The cells were seeded at a density of 3 × 104 cells/well in 24-well plates and incubated for 24 h at 37 °C in 5% CO2. The cells were treated with ICZ solution, S2, S5, and S6 formulations at a final concentration of 200 g/mL (below cytotoxicity concentration). The medium was removed after 6 h, the cells were washed three times with 1 mL of phosphate buffer saline, and lysed in 1 mL of lysis buffer (1 mM EDTA, 1% TritonX-100, 150 mM NaCl, 0.1% SDS, and 10 mM Tris-HCL). Then, 100 µL of cell lysate was collected in a microcentrifuge tube after 30 min. The tube was mixed with 900 µL of methanol. After precipitation, the supernatant was collected for ICZ analysis using HPLC.
Stability study
To assess the stability of ICZ in formulations and its physical properties, the selected ICZ-SM gels were kept at ambient (25 °C) and accelerated (40 °C/75% relative humidity) environments for 3 or 6 months. The physical properties of ICZ gels were tested after storage for 6 months (ambient condition). The experiments were performed in triplicate.
Statistical analysis
SPSS software for Windows (version 10.0) was used for statistical analysis (SPSS Inc., Chicago, IL, USA). The data were analyzed using a one-way analysis of variance and Tukey’s test at a 95% level of confidence.
Results and discussion
Preparation of ICZ-SM gels
From the results of our previous study, a clear appearance of the mixture was observed in the ternary phase diagram (Tubtimsri et al., Citation2022). The edges of clear areas in the ternary phase diagram were considered for use as the mixing ratios. The seven ratios of the mixture were selected to determine the solubility limit of ICZ in the formulation. The descending order of values for the solubilized ICZ (S1–S7) formulations is shown in . The highest solubility value of ICZ was 1.02 mg/g (S1), whereas the lowest solubility was 0.51 mg/g (S7). The trend of high solubility was dependent upon the mixture of MCT and P35. Based on these results, ICZ in a 0.5 mg/g formulation was used for further study; however, the solubility of IZC was 10-fold greater than the minimum inhibitory concentration (MIC) (Burgess et al., Citation2000). This result was consistent with a previous study (Souto et al., Citation2022), in which the amount of the oil phase affected the solubility of a lipophilic drug in an emulsion system or in lipid-based formulations. Thus, the ratio of oil and surfactant mixture influences the solubility of a lipophilic drug (Weerapol et al., Citation2014).
Table 1. The solubility of Itraconazole (ICZ) in different formulations. The data are presented as the mean ± SD (n = 3).
Robustness of dilution
The size of the SM gel diluted with saliva was determined to screen the self-dispersed emulsion from the SM gel (). An emulsion size larger than 200 nm and the appearance of a phase separation resulted in exclusion from further study. The high amount of MCT in S1 was separated after a 100-fold dilution with water, whereas a larger size emulsion (500–600 nm) was obtained after diluting S3 and S4. The ability of nanoemulsions to penetrate the epidermis depends on their size; therefore, S1, S3, and S4 were excluded from subsequent study (Su et al., Citation2017). The size of the formulation without ICZ before dilution was in the range of 42.89–250.98 nm. After loading ICZ, the size of the emulsion was larger compared with that without ICZ (289.02–4,205.23 nm). For the dilutions of S2 and S6 with or without ICZ, the size of the emulsion was similar. The solubilized ICZ was affected by the emulsion size after diluting S5 and S7. The loaded ICZ was slightly larger than without ICZ. When water was used to replace the artificial saliva, the results were similar (Table 1S). Consequently, the solubilized substances in the external phase (artificial saliva) may have no effect on the solute (ICZ) in the inner oil phase. The results of the dilution test were the same as that of previous studies (Solans & Solé, Citation2012). The microemulsion was diluted with water, and the water and surfactant combine to generate a spontaneous emulsion that encloses the oil droplets. After diluting with water, the surfactant concentration has an impact on the emulsion size. Nanoemulsions were observed when the surfactant content was >40% in the formulation.
Table 2. The size and size distribution of the emulsion before and after dilution of ICZ self- microemulsifying gels with artificial saliva. The data are presented as the mean ± SD (n = 3).
Rheology behavior of ICZ-SM gels
The viscoelasticity of the microemulsion was determined to evaluate the microstructure [G′ (elastic) and G″ (viscous)] of S5 and S6 with stain in the range of 0.1%–100% (). The G′ was higher than the G″ for both formulations and similar results were observed in S5 without ICZ (Tubtimsri et al., Citation2022), indicating a gel-like microstructure with an LVE of 0.1%–10% at 25 °C and 37 °C. The LVE of S6 at 25 °C was determined. A gel-like microstructure was also observed (G′ > G″) in the S6 formulation in the range of 0.1%–5% (25 °C) and 0.1%–25% (37 °C). In addition, G′ of S6 was higher compared with that of S5 at both temperatures, indicating a stronger microstructure for S5 compared with S6. For S2 and S7, no gel-like characteristics were observed in the test. These results were different compared with that of a previous study (Tubtimsri et al., Citation2022) in which monolaurin was loaded into SM. The G′ of the loaded monolaurin indicated a loss in gel strength compared with the blank formulation. In the present study, the gel strength did not decrease, probably because the drug did not affect the main gelling structure of the SM. Accordingly, the flow consistency (k) values of S2 and S7 were lower compared with those of S5 and S6, whereas the values of the power law index (n) for S2 and S7 were higher compared with those of S5 and S6 (). The results indicated that S5 and S6 were more viscous and flowed slower than S2 and S7. S5 exhibited the highest gel strength and viscosity. The power law analysis was also tested for the blank formulation, and the same results were observed. Based on the results, when oil, surfactant, and water are optimal, a strong interaction in the microstructure occurs with high elasticity and viscosity. In addition, we found that when using a higher proportion of P35, the microemulsion formed a stronger gel with a higher viscosity, and an oil phase located at the internal phase of the microemulsion (Alam & Aramaki, Citation2014).
Figure 1. Amplitude sweep curve of the relationship between G′, G″ (pa) and shear strain (%), (a) 25 °C and (b) 37 °C of ICZ self-microemulsifying gels.

Table 3. The viscosity of the ICZ self-microemulsifying gels was measured by power law analysis. The data are presented as the mean ± SD (n = 3).
The relationship of G′, G″ and the temperature (25 °C–45 °C) of S5 and S6 are presented in . The increase in temperature affected the viscoelasticity of the microemulsion, and G′ and G″ decreased while temperature increased. However, the elasticity as a gel-like property of the microemulsion remained, indicating that the varying temperature only slightly affected gel strength and the microstructure of SM was not damaged by varying temperature.
Released drug from the ICZ-SM gel
In the in vitro release test, S5 and S6 exhibited a sustained release from the microstructure. S2 and S7 were low viscosity samples with no gel-like characteristics, and the study used only S2 to represent low-viscosity samples, with no gel-like SM in the dissolution test. The in vitro ICZ release was carried out using a USP apparatus IV. At 360 min, more than 95% of the ICZ was released from the SM gels (). The burst release (95%) of the ICZ solution was observed at 30 min, and the complete release occurred at 60 min. A similar profile was observed for S2, in which 95% of the released ICZ occurred at 30 min. For samples S5 and S6, ICZ was >95% dissolved at 360 min. A remarkable difference in ICZ release was obtained at 180 min, and 80% and 56% were observed for S6 and S5, respectively. The kinetic model of ICZ release was used to compare all profiles (). The burst-release ICZ profile (S2) was fit to a first-order model (r2 = 0.9824–0.9836). The best fit model of S6 was a first-order model (r2 = 0.9579–0.9619), whereas S5 fit a zero-order model (r2 = 0.9780–0.9806). Furthermore, the release profiles of S5 and S6 were related to the model of Higuchi, in which a better relationship to the Higuchi model for S5 (r2 = 0.8872–0.8930) was observed compared with that of S6 (r2 = 0.8315–0.8331). These results suggest that S2 may not be useful for the sustained release ICZ (Laracuente et al., Citation2020). The ICZ release profiles calculated by various release models from S5 and S6 demonstrated the ability to sustain drug release from the microemulsion within 360 min. Similar results were found in a study of a curcumin topical gel (Scomoroscenco et al., Citation2021). The sustained release of curcumin was modified using a microemulsion (polymer-free) consisting of Tween 80 and Plurol®. This study showed that the curcumin release profiles from a microemulsion were satisfied by a zero-order and Higuchi model because of the homogeneous drug distribution. A spontaneous microemulsification method was used to create a gel preparation containing 0.05% tazarotene for acne treatment (Patel et al., Citation2016). The Higuchi model also fit with a sustained release microemulsion for modified permeation of the topical preparation. For the transdermal delivery of all-trans retinoic acid loaded into microemulsions (Subongkot & Ngawhirunpat, Citation2017), the release kinetics of each formulation were assessed. All of the release patterns for the microemulsion formulations were best suited to the zero-order model, based on the coefficient of determination (r2). Gatifloxacin microemulsion was developed by mixing diethylene glycol monoethyl ether, water, and Tween 80 (Kalam et al., Citation2016). For these formulations, the coefficient of variation was less for the first-order model and was higher for the zero-order model.
Figure 3. In vitro release profiles of selected ICZ self-microemulsifying gels (S2, S5, and S6) and ICZ solution (in PEG200) in simulated salivary fluid (pH 6.75). the data are presented as the mean ± SD (n = 3).

Table 4. Comparation of the drug-release model for ICZ self-microemulsifying gels (n = 3).
Kinetics of time-killing by ICZ-SM gels
Time-killing kinetic analysis was done to estimate the duration and rate of antibacterial activity enhanced by SM (Kalam et al., Citation2016; Khumpirapang et al., Citation2021). SDB and C. albicans were used to evaluate the antifungal activity of the optimized formulations (e.g. S2, S5, and S6) (). The antifungal activity (cell rupture) by surfactant was observed in formulations without ICZ (the base for S2, S5, and S6) and compared with negative control samples (Falk, Citation2019). After 360 min, the ICZ solution and S2 formulation did not completely kill the cells. The cells were reduced 50% by the ICZ solution, whereas a low level (0.0125 × 104 CFU/mL) remained following S2 treatment. Samples S5 and S6 exhibited high antifungal activity, attributed to the presence of a characteristic gel. The gel accumulates on the cell surface and is gradually dispersed into an emulsion, resulting in a high concentration on the surface of fungal cells (Barot et al., Citation2012). This leads to enhanced antifungal activity relative to that of S2. Our findings are in agreement with that of another group (Khumpirapang et al., Citation2021), which suggests that the rapid killing capacity of microemulsion formulations indicates a direct attack on the structural integrity of the fungal cell and may disrupt the membrane structure. Killing by the optimized formulation was caused by a synergistic effect of cells ruptured by the microemulsion and the pharmacodynamic activity of ICZ (Agrawal et al., Citation2021).
Figure 4. a. Time exposure viability curves for cultures of C. albicans (1 × 104 CFU/mL) after inoculation with ICZ self-microemulsifying gels, self-microemulsifying gels without ICZ (base), ICZ solution, and a negative control for 6 h. The data are presented as the mean ± SD (n = 3). b. AUC of viability curve of C. albicans (1 × 104 CFU/mL) after inoculation with ICZ self-microemulsifying gels without ICZ (base), ICZ solution, and negative control for 6 h. The data are presented as the mean ± SD (n = 3). (*p < .05).

The AUC of the viability curve of C. albicans was calculated () for statistical comparisons (Appiah et al., Citation2017). The antifungal activity of ICZ solution and SM with and without ICZ was significantly different compared with the negative control. A difference was observed between the ICZ solution and SM with or without ICZ. A lower viability was observed for the ICZ solution compared with the base of S2, S5, or S6, whereas an insignificant difference was found among the bases of S2, S5, or S6. High potency was observed in the selected formulations and in descending order from S5, S6, to S2, respectively, and the results were in accordance with the in vitro dissolution study.
ICZ cellular uptake
To achieve pharmacological effectiveness, drug permeability is essential. If a drug is applied topically, whether it is sufficiently concentrated to have a therapeutic impact on the local area depends on how much of the drug is absorbed into the cell (Hoppel et al., Citation2015; Nastiti et al., Citation2017). Oral cells are different from body skin cells, which are not composed of keratin. In this study, the human oral squamous cell carcinoma, KON cells, which are derived from non-keratinocyte cells in the mouth, were employed (Sekikawa et al., Citation2018; Pandey et al., Citation2022). After exposure to each formulation for 6 h, the percentage of cellular absorption of the ICZ solution and the ICZ-SM gels (S2, S5, and S6) was determined (). The percentage of drug uptake in each sample was significantly different among the ICZ-SM gels and different from the ICZ solution (p < .05). The percentages of cellular absorption for S2, S5, S6, and ICZ solution were 3.07% ± 0.14%, 10.31% ± 0.91%, 7.02% ± 0.43%, and 0.22% ± 0.02%, respectively. The highest ICZ cellular uptake was observed in the S5 treatment group at 47-, 3-, and 1.5-fold compared with ICZ solution, S2, and S6, respectively. The results correlated with the in vitro release study and kinetic killing test. S2 can protect ICZ in the emulsion and increase the absorption of the poorly water-soluble drug (Mahrhauser et al., Citation2015; Todosijević et al., Citation2015). A similar result was observed for the enhanced permeation of drug by the microemulsion base (Mahrhauser et al., Citation2014; Rajabalaya et al., Citation2017). The lipid in the stratum corneum was interrupted by lipid or fatty acids in the microemulsion, which caused increased disorder of the cell layer in the stratum corneum and the lipid of the cell. The higher temporary disorder in cells results in greater drug uptake. In addition, the viscosity or gel consistency of the microemulsion (S5 and S6), which is suitable for its retention on the surface and increases contact time, results in greater drug absorption (Naeem et al., Citation2015). The high concentration of surfactants in these gels can also lead them to interact with the lipids in the cellular microstructure, improving the permeability of the drug (Rajabalaya et al., Citation2017). Saliva could disperse the microemulsion in the buccal cavity, creating a potential additional site of drug absorption.
Stability study
The ICZ-SM gels showed no visible physical changes during the 3 to 6-month study period, as a turbid solution or appearance of phase separation were not evident in any sample. The ICZ content of approximately 100% was similar to that of a fresh preparation (). The rheological behavior of the ICZ-SM gels was comparable to that of the initial day. The viscosity and their components after storage were the same compared with the initial day (Table 2S). The size and size distribution of the emulsion before and after dilution of the ICZ-SM gels in stored samples were the same as fresh preparations (Table 3S). The dissolution profiles of the product after the storage period were comparable with the initial day (Fig 1S). The time-killing kinetic analysis of the product was found to be equivalent to that of the initial day (Fig. 2S).
Table 5. ICZ percentage Remaining after storage under accelerated (relative humidity 40 °C/75%) and ambient (25 °C) conditions (n = 3).
Conclusion
ICZ-SM gels were prepared by mixing ICZ powder with a microemulsion base at various ratios. In the dilution test, S1, S3, and S4 exhibited phase separation or a large emulsion size. Rheology of the ICZ-SM gels indicated that high gel strength for the S5 and S6 formulations, whereas S2 and S7 had low elasticity values. The release of ICZ from the gels was best fit with the model of zero-order kinetics (S5) and first-order kinetics (S6). The time-killing test revealed that S5 and S6 required less time compared with S2 and ICZ solution. Furthermore, enhanced cell uptake was observed for S5 with a higher adsorption compared with S2, S6, and the ICZ solution. Our findings indicated that the ICZ-SM gel is a free polymer capable of delivering ICZ for the treatment of oral candidiasis and has enhanced cellular absorption. Potential studies that would compare the therapeutic effectiveness of the developed formulations for oral candidiasis in mouse models are under consideration.
Authors’ contributions
Sukannika Tubtimsri: Conceptualization, methodology, investigation, supervision, project administration, data curation and writing—original draft preparation. Yotsanan Weerapol: Conceptualization, methodology, investigation, data curation supervision, project administration and writing—review and editing. All authors have read and agreed to the published version of the manuscript.
Ethical approval
There are no studies with human participants or animals performed by any of the authors in this article.
Funding statement
This study was not supported by any governmental, private, or nonprofit funding agencies.
Supplemental Material
Download MS Word (28.5 KB)Acknowledgments
The authors would like to express great thankfulness to BASF Thailand for a supplied sample of polyoxyl 35 castor oil.
Disclosure statement
No potential conflict of interest was reported by the authors.
Data availability statement
All data are included in the manuscript.
References
- Abd E, Benson HAE, Roberts MS, Grice JE. (2018). Minoxidil skin delivery from nanoemulsion formulations containing eucalyptol or oleic acid: enhanced diffusivity and follicular targeting. Pharmaceutics 10:1–9. doi: 10.3390/pharmaceutics10010019.
- Aggarwal N, Goindi S, Khurana R. (2013). Formulation, characterization and evaluation of an optimized microemulsion formulation of griseofulvin for topical application. Colloids Surf B Biointerfaces 105:158–166. doi: 10.1016/j.colsurfb.2013.01.004.
- Agrawal V, Patel R, Patel M, et al. (2021). Design and evaluation of microemulsion-based efinaconazole formulations for targeted treatment of onychomycosis through transungual route: ex vivo and nail clipping studies. Colloids Surf B Biointerfaces 201:111652. doi: 10.1016/j.colsurfb.2021.111652.
- Alam MM, Aramaki K. (2014). Liquid crystal-based emulsions: progress and prospects. J Oleo Sci 63:97–108. doi: 10.5650/jos.ess13101.
- Alves Barroso L, Grossi Bovi Karatay G, Dupas Hubinger M. (2022). Effect of potato starch hydrogel: glycerol monostearate oleogel ratio on the physico-rheological properties of bigels. Gels 8:694–694. doi: 10.3390/gels8110694.
- Appiah T, Boakye YD, Agyare C. (2017). Antimicrobial activities and time-kill kinetics of extracts of selected Ghanaian mushrooms. Evid Based Complement Alternat Med 2017:4534350. doi: 10.1155/2017/4534350.
- Barot BS, Parejiya PB, Patel HK, et al. (2012). Microemulsion-based gel of terbinafine for the treatment of onychomycosis: optimization of formulation using D-optimal design. AAPS PharmSciTech 13:184–192. doi: 10.1208/s12249-011-9742-7.
- Burgess DS, Hastings RW, Summers KK, et al. (2000). Pharmacodynamics of fluconazole, itraconazole, and amphotericin B against Candida albicans. Diagn Microbiol Infect Dis 36:13–18. doi: 10.1016/s0732-8893(99)00097-8.
- Cao R, Wan Q, Tan L, et al. (2021). Evaluation of the vital viability and their application in fungal spores’ disinfection with flow cytometry. Chemosphere 269:128700. doi: 10.1016/j.chemosphere.2020.128700.
- Essawy MM, El-Sheikh SM, Raslan HS, et al. (2021). Function of gold nanoparticles in oral cancer beyond drug delivery: implications in cell apoptosis. Oral Dis 27:251–265. doi: 10.1111/odi.13551.
- Falk NA. (2019). Surfactants as antimicrobials: a brief overview of microbial interfacial chemistry and surfactant antimicrobial activity. J Surfactants Deterg 22:1119–1127. doi: 10.1002/jsde.12293.
- Hoppel M, Juric S, Ettl H, Valenta C. (2015). Effect of monoacyl phosphatidylcholine content on the formation of microemulsions and the dermal delivery of flufenamic acid. Int J Pharm 479:70–76. doi: 10.1016/j.ijpharm.2014.12.048.
- Hua S. (2019). Advances in nanoparticulate drug delivery approaches for sublingual and buccal administration. Front Pharmacol 10:1328. doi: 10.3389/fphar.2019.01328.
- Jaiswal P, Aggarwal G, Harikumar SL, Singh K. (2014). Development of self-microemulsifying drug delivery system and solid-self-microemulsifying drug delivery system of telmisartan. Int J Pharm Investig 4:195–206. doi: 10.4103/2230-973X.143123.
- Kalam MA, Alshamsan A, Aljuffali IA, et al. (2016). Delivery of gatifloxacin using microemulsion as vehicle: formulation, evaluation, transcorneal permeation and aqueous humor drug determination. Drug Deliv 23:896–907. doi: 10.3109/10717544.2014.920432.
- Khumpirapang N, Klayraung S, Tima S, Okonogi S. (2021). Development of microemulsion containing Alpinia galanga oil and its major compounds: enhancement of antimicrobial activities. Pharmaceutics 13:265–265. doi: 10.3390/pharmaceutics13020265.
- Laracuente ML, Yu MH, McHugh KJ. (2020). Zero-order drug delivery: state of the art and future prospects. J Control Release 327:834–856. doi: 10.1016/j.jconrel.2020.09.020.
- Latifah-Munirah B, Himratul-Aznita WH, Mohd Zain N. (2015). Eugenol, an essential oil of clove, causes disruption to the cell wall of Candida albicans (ATCC 14053). Front Life Sci 8:231–240. doi: 10.1080/21553769.2015.1045628.
- Mahrhauser D, Hoppel M, Schöll J, et al. (2014). Simultaneous analysis of skin penetration of surfactant and active drug from fluorosurfactant-based microemulsions. Eur J Pharm Biopharm 88:34–39. doi: 10.1016/j.ejpb.2014.04.019.
- Mahrhauser DS, Kählig H, Partyka-Jankowska E, et al. (2015). Investigation of microemulsion microstructure and its impact on skin delivery of flufenamic acid. Int J Pharm 490:292–297. doi: 10.1016/j.ijpharm.2015.05.056.
- Naeem M, Ur Rahman N, Tavares GD, et al. (2015). Physicochemical, in vitro and in vivo evaluation of flurbiprofen microemulsion. An Acad Bras Cienc 87:1823–1831. doi: 10.1590/0001-3765201520130436.
- Nastiti CMRR, Ponto T, Abd E, et al. (2017). Topical nano and microemulsions for skin delivery. Pharmaceutics 9:37–37. doi: 10.3390/pharmaceutics9040037.
- Noël de Tilly A, Tharmalingam S. (2022). Review of treatments for oropharyngeal fungal infections in HIV/AIDS patients. Microbiol Res 13:219–234. doi: 10.3390/microbiolres13020019.
- Pandey M, Choudhury H, Ying JNS, et al. (2022). Mucoadhesive nanocarriers as a promising strategy to enhance intracellular delivery against oral cavity carcinoma. Pharmaceutics 14:795. doi: 10.3390/pharmaceutics14040795.
- Patel MR, Patel RB, Parikh JR, Patel BG. (2016). Novel microemulsion-based gel formulation of tazarotene for therapy of acne. Pharm Dev Technol 21:921–932. doi: 10.3109/10837450.2015.1081610.
- Patel P, Pol A, Kalaria D, et al. (2021). Microemulsion-based gel for the transdermal delivery of rasagiline mesylate: in vitro and in vivo assessment for Parkinson’s therapy. Eur J Pharm Biopharm 165:66–74. doi: 10.1016/j.ejpb.2021.04.026.
- Rajabalaya R, Musa MN, Kifli N, David SR. (2017). Oral and transdermal drug delivery systems: role of lipid-based lyotropic liquid crystals. Drug Des Devel Ther 11:393–406. doi: 10.2147/DDDT.S103505.
- Said M, Elsayed I, Aboelwafa AA, Elshafeey AH. (2017). Transdermal agomelatine microemulsion gel: pyramidal screening, statistical optimization and in vivo bioavailability. Drug Deliv 24:1159–1169. doi: 10.1080/10717544.2017.1365392.
- Scomoroscenco C, Teodorescu M, Raducan A, et al. (2021). Novel gel microemulsion as topical drug delivery system for curcumin in Dermatocosmetics. Pharmaceutics 13:505–505. doi: 10.3390/pharmaceutics13040505.
- Sekikawa S, Onda T, Miura N, et al. (2018). Underexpression of alpha-1-microglobulin/bikunin precursor predicts a poor prognosis in oral squamous cell carcinoma. Int J Oncol 53:2605–2614. doi: 10.3892/ijo.2018.4581.
- Sharma DK, Sharma P. (2022). Augmented glutathione absorption from oral mucosa and its effect on skin pigmentation: a clinical review. Clin Cosmet Investig Dermatol 15:1853–62. doi: 10.2147/CCID.S378470.
- Shukla T, Upmanyu N, Agrawal M, et al. (2018). Biomedical applications of microemulsion through dermal and transdermal route. Biomed Pharmacother 108:1477–1494. doi: 10.1016/j.biopha.2018.10.021.
- Solans C, Solé I. (2012). Nano-emulsions: formation by low-energy methods. Curr Opin Colloid Interface Sci 17:246–254. doi: 10.1016/j.cocis.2012.07.003.
- Souto EB, Cano A, Martins-Gomes C, et al. (2022). Microemulsions and nanoemulsions in skin drug delivery. Bioengineering (Basel) 9:158. doi: 10.3390/bioengineering9040158.
- Stojkov G, Niyazov Z, Picchioni F, Bose RK. (2021). Relationship between structure and rheology of hydrogels for various applications. Gels 7:255–255. doi: 10.3390/gels7040255.
- Su R, Fan W, Yu Q, et al. (2017). Size-dependent penetration of nanoemulsions into epidermis and hair follicles: implications for transdermal delivery and immunization. Oncotarget 8:38214–38226. doi: 10.18632/oncotarget.17130.
- Subongkot T, Ngawhirunpat T. (2017). Development of a novel microemulsion for oral absorption enhancement of all-trans retinoic acid. Int J Nanomedicine 12:5585–5599. doi: 10.2147/IJN.S142503.
- Todosijević MN, Savić MM, Batinić BB, et al. (2015). Biocompatible microemulsions of a model NSAID for skin delivery: a decisive role of surfactants in skin penetration/irritation profiles and pharmacokinetic performance. Int J Pharm 496:931–941. doi: 10.1016/j.ijpharm.2015.10.048.
- Triboandas H, Pitt K, Bezerra M, et al. (2022). Itraconazole amorphous solid dispersion tablets: formulation and compaction process optimization using quality by design principles and tools. Pharmaceutics 14:2398–2398. doi: 10.3390/pharmaceutics14112398.
- Tubtimsri S, Weerapol Y, Soontaranon S, et al. (2022). Monolaurin-loaded gel-like microemulsion for oropharyngeal candidiasis treatment: structural characterisation and in vitro antifungal property. AAPS PharmSciTech 23:87. doi: 10.1208/s12249-022-02235-7.
- Venugopal DC, Senthilnathan RD, Maanvizhi S, et al. (2023). Preparation and characterization of silymarin gel: a novel topical mucoadhesive formulation for potential applicability in oral pathologies. Gels 9:139. doi: 10.3390/gels9020139.
- Weerapol Y, Limmatvapirat S, Nunthanid J, Sriamornsak P. (2014). Self-nanoemulsifying drug delivery system of nifedipine: impact of hydrophilic-lipophilic balance and molecular structure of mixed surfactants. AAPS PharmSciTech 15:456–464. doi: 10.1208/s12249-014-0078-y.
- Weerapol Y, Limmatvapirat S, Takeuchi H, Sriamornsak P. (2015). Fabrication of spontaneous emulsifying powders for improved dissolution of poorly water-soluble drugs. Powder Technol 271:100–108. doi: 10.1016/j.powtec.2014.10.037.
- Weerapol Y, Sriamornsak P. (2020). Differences in viscoelasticity of ophthalmic polymer solution after sterilization. Walailak J Sci & Tech 17:686–697. doi: 10.48048/wjst.2020.6341.
- Zhang Y, Huo M, Zhou J, et al. (2010). DDSolver: an add-in program for modeling and comparison of drug dissolution profiles. AAPS J 12:263–271. doi: 10.1208/s12248-010-9185-1.