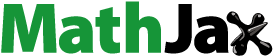
Abstract
Herein we designed, optimized, and characterized the Metformin Hydrochloride Transethosomes (MTF-TES) and incorporate them into Chitosan gel to develop Metformin Hydrochloride loaded Transethosomal gel (MTF-TES gel) that provides a sustained release, improved transdermal flux and improved antidiabetic response of MTF. Design Expert® software (Ver. 12, Stat-Ease, USA) was applied for the statistical optimization of MTF-TES. The formulation with Mean Particle Size Distribution (MPSD) of 165.4 ± 2.3 nm, Zeta Potential (ZP) of −21.2 ± 1.9 mV, Polydispersity Index (PDI) of 0.169 ± 0.033, and MTF percent Entrapment Efficiency (%EE) of 89.76 ± 4.12 was considered to be optimized. To check the chemical incompatibility among the MTF and other formulation components, Fourier Transform Infrared (FTIR) spectroscopy was performed and demonstrated with no chemical interaction. Surface morphology, uniformity, and segregation were evaluated through Transmission Electron Microscopy (TEM). It was revealed that the nanoparticles were spherical and round in form with intact borders. The fabricated MTF-TES has shown sustained release followed by a more pronounced effect in MTF-TES gel as compared to the plain MTF solution (MTFS) at a pH of 7.4. The MTF-TES has shown enhanced permeation followed by MTF-TES gel as compared to the MTFS at a pH of 7.4. In vivo antidiabetic assay was performed and results have shown improved antidiabetic potential of the MTF-TES gel, in contrast to MTF-gel. Conclusively, MTF-TES is a promising anti-diabetic candidate for transdermal drug delivery that can provide sustained MTF release and enhanced antidiabetic effect.
1. Introduction
Diabetes is a chronic metabolic illness distinguished by hyperglycemia having heterogeneous etiopathology which includes insulin resistance, lack of its secretion, or both (Patiño-Herrera et al., Citation2019). It is one of the major causes of mortality having several concomitant complications such as hypertension, varied lipid metabolism, and cardiovascular diseases (Torabian et al., Citation2022). Type 2 diabetes mellitus (T2DM) is characterized by irregular glucose metabolism caused by decreasing pancreatic β cells working with the classical indications of polyuria, polydipsia, and polyphagia (Udokang, Citation2012; San Tang, Citation2019). It is now a universal health problem accounting for almost 463 million people worldwide and spending about 11% (∼612.2 billion USD) of the global healthcare disbursement as reported by the International Diabetes Federation (IDF) and by 2045 the number of diseased patients is expected to escalate to 700 million people, updated last by 2019 (Kumar et al., Citation2017; Luo et al., Citation2022).
Myriad oral pharmacotherapies for the management of T2DM are available including Biguanides (Luft et al., Citation1978), meglitinides (Black et al., Citation2007), sulfonylureas (Del Prato & Pulizzi, Citation2006), dipeptidyl peptidase IV inhibitors (Demuth et al., Citation2005), α-glucosidase inhibitors (Van De Laar et al., Citation2005) and thiazolidinediones (Soccio et al., Citation2014), however, their unwanted side effects such as nausea, diarrhea, weight gain, hypoglycemia, and heart failure contribute significantly to their limited use (Cetin et al., Citation2013). Among them, Metformin (MTF) from the category of biguanides has been widely prescribed as a foundation drug for all patients with initially diagnosed T2DM (Momoh et al., Citation2013). MTF inhibits hepatic gluconeogenesis, suppresses glucose absorption by the intestine, and ameliorates glucose consumption by muscle cells (Migdadi et al., Citation2018). It belongs to the class III of the biopharmaceutical classification system (BCS) and is presented with high solubility and low permeability (Kim et al., Citation2022). Despite being widely used, MTF when administered orally is associated with a plethora of side effects and problems that reduce its therapeutic effectiveness entailing poor and partial gastrointestinal (GIT) absorption (40–60%) (Kenechukwu et al., Citation2022) and relatively rapid pre-systemic clearance which results in limited bioavailability and a short elimination half-life of nearly 6.2 h (Cesur et al., Citation2022). These pharmacokinetic characteristics of MTF culminate into frequent dosing, high dose, elevated incidence of dose-related adverse effects, and curtailed patient adherence (Kamboj & Verma, Citation2018). The GIT-related adverse effects of MTF may include diarrheal stomach pain, anorexia, vomiting, and nausea. It is also related to several other undesirable side effects such as lactic acidosis having symptoms like muscle pain, tremors, severe drowsiness, dizziness, fatigue, difficulty in breathing, and irregular heartbeat (Sankhyan & Pawar, Citation2013; Saini et al., Citation2016).
Transdermal drug delivery system (TDDS) promises significant advantages over the oral route as it is non-invasive, can circumvent the first pass effect and alleviates the GIT side effects (Kumar & Utreja, Citation2020). However, the transdermal bioavailability of therapeutics is constrained by the stratum corneum (SC) which exhibits the most resilient impediment to drug absorption through the skin (Ahad et al., Citation2018). Drug nanoencapsulation is growing as a potential substitute that provides deeper skin penetration, controlled release, and site-specific delivery of drugs (Rostamkalaei et al., Citation2019). Transethosomes (TES) are new-generation ultra-deformable lipid-based nanovesicles containing lipids, an edge activator, and ethanol. Contrary to the traditional liposomes, which are reluctant to penetrate the deeper layers of skin and stay restricted to the SC these ultra-deformable vesicles could penetrate deep and perform their work (Ahmed et al., Citation2021). They are characterized by elevated thermodynamic stability and loading capacity having properties of both ethosomes and transfersomes. TES have an edge over other drug delivery systems as they have ethanol in them (Costanzo et al., Citation2021). Ethanol improves drug penetration from the minute holes created in the SC as a result of lipid bilayer membrane fluidization, thus decreasing the strength of the lipid bilayer. They become ultra-deformable and very elastic due to the presence of an edge activator and its ability to destabilize the phospholipid bilayer (Song et al., Citation2012). On topical application, these nanocarriers not only save individuals from intrusive parental therapy but also prevent the undesirable side effects produced by the parenteral or oral route. Therefore, it is anticipated that these drug-laden vesicles will have a positive effect on therapeutic potential (Majumder et al., Citation2019).
Gels are believed to be potential delivery systems for retaining nanoparticles or medications at the intended application site. Owing to their mucoadhesive nature, they also lengthen the drug residence time. Retaining the TES at the desirable site for an extended time duration is challenging by their low viscosity (Rabia et al., Citation2020). Therefore, metformin-loaded transethosomes (MTF-TES) were encapsulated into chitosan gel for effective skin distribution and to achieve optimal conditions for transdermal drug delivery. Nowadays, there is a significant amount of interest in the utilization of chitosan in medical and pharmaceutical applications. Its intriguing inherent qualities are undoubtedly the key causes of this growing attention (Berger et al., Citation2004). Chitosan gel was used to incorporate MTF-TES into the gel to fabricate the MTF-TES laden gel (MTF-TES gel) as it is biodegradable, biocompatible, nontoxic, and possesses enhanced stability (Verma et al., Citation2020).
The present research work, nonetheless, aimed at the fabrication of MTF-TES and loading them into chitosan gel that would provide sustained release, ameliorates the incidences of unwanted side effects, and reduces the necessity of repeated dosing. By using Box Behnken design®, the formulation has been optimized systematically and after that, the characterizations were carried out. Moreover, the antidiabetic model was developed in which fasting blood glucose and body weight of the rats treated with MTF-TES were determined and were compared with the rats treated with free MTF which confirmed its enhanced antidiabetic activity.
2. Materials and methods
2.1. Reagents and chemicals
Metformin HCl was purchased from Sigma Aldrich (Darmstadt, Germany). A phospholipid Phospholipon 90 G was taken as a gift of research from Lipoid GmBH, Switzerland. Tween 80® as an Edge activator and LMW chitosan was taken from Saint-Priest, France. Sigma Aldrich also provides our lab Ethanol, Methanol, isopropyl alcohol, and Chloroform. Streptozotocin, a diabetes inducer, was purchased from Sigma Aldrich (Germany).
2.2. Equipment and apparatus
Equipment including weight balance (Make: OHAUS, USA, Model: PA 214 C), Transmission electron microscopy (Make: Philips, Japan, Model: CM10), Magnetic stirrer with Hot plate, FTIR (Model: United States Perkin-Elmer), Rotary Evaporator (Preparation: Germany, Heidolph GmBH, Model: Hei Vap Core) and Vortex mixer were used. Similarly, Franz-diffusion cell (Manufacturer: United States Permegear), Microtiter plate reader (Make: BioTek, United States), Centrifuge (Manufacturer: Germany HERMLE Labortechnik, Model: Z 216 MK), Zeta Sizer (Model: Nano ZS, UK), UV spectrophotometer (Manufacturer: United States), pH meter (Manufacturer: Unites States, Bante Instruments, Model: PHS 25 GW), and lyophilizer Manufacturer: Germany GmBH Martin Christ, Model: Alpha 1–2 LD Plus) were also employed in this study.
2.3. Animals
BALB/c mice approximately 30–35 g were purchased from NIH, Islamabad for use in the ex vivo penetration experiment through the skin. For in vivo antidiabetic assay, 6–8 weeks old, 20 Sprague Dawley rats were purchased from Riphah International University, Islamabad weighing 250 ± 30 g. The antidiabetic study was done after the approval of Quaid-i-Azam University ethical committee (BEC-FBS-QAU2022-385) as per the Guide for the Care and Use of Laboratory Animals (8th Edition) and ARRIVE (Version 2) guidelines. All the animals were housed and acclimated in the Quaid-i-Azam University animal house at 25.0 ± 2 °C with a 12 h/12 h light-dark cycle. Moreover, all the animals were fed with filtered tap water and standard feed.
2.4. Fabrication of MTF-TES
MTF-TES were fabricated by the thin film hydration method as reported earlier (Nayak et al., Citation2020). Generally, a thin organic layer was prepared by dissolving the Phospholipon 90 G (PL 90 G) in methanol. This blend was then placed in a flask in the rotary evaporator that functioned under vacuum; the temperature was kept at 60 °C and rotated at a speed of 100 rpm to make a layer of the thin film at the bottom of the flask. Erstwhile, tween 80® and MTF in 30% v/v hydroethanolic solution were used to prepare the hydration phase, and it was afterward used to hydrate the already formed thin film and again put on the rotary evaporator for 1 h at the previously stated temperature. Furthermore, to reduce the size of the prepared TES, extrusion was done from filters (3 times from 0.45 µm and 2 times from 0.22 µm filters) as illustrated in (Khalid et al., Citation2022). Various concentrations of hydroethanolic solution were formulated in pre-formulation studies and 30% were found to be the appropriate one. A hydroethanolic solution of 30% v/v was prepared by adding 3 ml ethanol in 7 ml distilled water which was used as an aqueous phase (Kumar et al., Citation2016).
2.5. Optimization of MTF-TES
Preformulation studies were performed to determine the upper and lower limits of independent variables and the blank formulations were also prepared. To statistically optimize the formulation, Box-Behnken design using Design Expert® software (Ver. 12) was applied and 14 runs were given by it. All the formulations were fabricated and the effect on the dependent variable (%EE, PDI, ZP, and P.S) by variation of independent factors (PL 90 G, Tween 80®, and Ethanol) was evaluated. All the factors; independent as well as dependent are stated in (Moolakkadath et al., Citation2018).
Table 1. Levels of independent and desired outcome of dependent factor employed in Box-Behnken design.
2.6. Characterization of fabricated MTF-TES
The characterization of optimized MTF-TES was done in terms of myriad factors including mean particle size distribution (MPSD), polydispersity index (PDI), and zeta potential (Z.P), and was determined using Malvern Zeta Sizer (Model: ZS90, Worcestershire, UK) which was placed in 25 ± 0.5 °C temperature-maintained environment. The study was performed in triplicate and the samples were formulated by diluting 10 µl MTF-TES into 990 µl deionized water. Standard error as a Mean ± S.D. was also depicted in the results (Dar et al., Citation2018).
2.7. Percent entrapment efficiency
The indirect method was used for the determination of entrapment efficiency. The formulated MTF-TES were centrifuged for 3 h. The temperature of −4 °C and the speed of 15,000 rpm were maintained to isolate the free dug. After centrifugation, samples were taken and the supernatant was separated and analyzed under a UV spectrophotometer (Model: HALO DB 20, Make: Dynamica, UK) at the wavelength of 234 nm. The following formula was used to check the EE:
Where, D1 is the amount of entrapped MTF inside the nano-vesicle and D2 is the amount of cumulative drug added (Dar et al., Citation2018).
2.8. TEM analysis
Surface morphology, uniformity, and segregation were evaluated using TEM as it is imperative to characterize this for nanosized particles. Before analysis, the sample was treated negatively with phosphotungstic acid and then the analysis was done at a voltage of 100 kV (Rizvi et al., Citation2019). Furthermore, the ImageJ software was employed to analyze the particle size from the TEM micrograph.
2.9. FTIR analysis
To check any incompatibility or chemical interaction between the formulation and its components FTIR was used (Bibi et al., Citation2022). For this, the optimized TES, PL 90 G, drug, and physical mixture of drug and PL 90 G was consigned to FTIR scanning as per the protocols, previously stated (El-Gizawy et al., Citation2020).
2.10. Fabrication of MTF-TES gel
The formulated MTF-TES were encapsulated within a hydrogel made of chitosan to be applied to the skin efficiently. Chitosan gel with different concentrations were prepared (ranging from 1.5 to 4%). Among them 2% chitosan gel was found to be the appropriate one, with respect to its optimum viscosity (Hurler et al., Citation2012). For the preparation of chitosan gel, 2% w/v of the low molecular weight (LMW) chitosan was added in 1% acetic acid solution and the formulation was then added with continuous stirring to fabricate the MTF-TES gel. To prepare a 1% acetic acid solution, 1 ml acetic acid was added to 99 ml distilled water (Ternullo et al., Citation2019).
2.11. Characterization of MTF-TES gel
The pH, clarity, homogeneity, and rheological properties of the MTF-TES gel were evaluated. A digital meter was used to determine the pH of MTF-TES GEL. Briefly, 30 ml distilled water was poured over 1 g of chitosan gel, was thoroughly dissolved, followed by assessing the pH in triplicate (Salim et al., Citation2020). Brookfield Viscometer (Model: DV3T, Middleborough, MA) was used to measure the flow behavior of the MTF-TES gel. To assess the impact of stress or strain, the chitosan gel viscosity was evaluated at various speeds (0.5, 1, 2, 3.5, 4, 5.4, 6, 9.5, 14, 22, 36, 58, 64, and 100 rpm) with the torque of (4.6, 9.1, 13.4, 17.9, 20.0, 22.6, 24.5 27.4, 35.3, 37.1 49.7, 59.3, 81.3, 85.9 and 94.3%) and flow patterns at 25 ± 2 °C. A stress-associated dependent or independent viscosity pattern was observed (Jamshaid et al., Citation2022). Visual examination was used to assess the consistency and homogeneity of the MTF-TES gel. The gel was examined to see whether or not there were any bunches or agglomerates, and any clumps or agglomerates were documented. To assess the transparency of the MTF-TES gel, visual observation was used (Aggarwal et al., Citation2012; Amin et al., Citation2013). To determine the Spreadability, the petri dish method was employed. With this technique, a circle of 1 cm2 was drawn upon the petri dish and the MTF-TES gel of 0.5 g was then placed on that circle. The petri dish was covered with a glass lid with a weight of 500 g placed over it. The precise area across which the gel was placed was finally calculated (Ahad et al., Citation2016). The diameter increment has been measured using the following equation.
Whereas, Si is the spread area in mm2 and symbol d denotes the spread diameter of the gel (Bachhav & Patravale, Citation2009). The formulated MTF-TES gel was put in a beaker with methanol and left there for 48 h while being stirred. After the specified time duration, all the collected formulations were evaluated, and using a UV spectrophotometer, (Patil et al., Citation2015) percent drug content was calculated using the following formula;
2.12. In vitro drug release
To determine the release pattern of MTF, the dialysis bag method was used. For this, the release of MTF from MTF-TES and MTF-TES gel was determined and their comparison with metformin HCl solution (MTFS) was done. MTFS, MTF-TES, and MTF-TES gel were all placed in individual dialysis bags, having the same quantity of each formulation carrying 3 mg of the drug, and then placed in beakers containing 50 ml of phosphate buffer solution (PBS). Then, the beakers were set in a shaking water bath and the temperature of the water shaker bath was maintained at 37 ± 0.5 °C and the speed at 100 rpm. At pH of 5.5 and 7.4, the in vitro drug release study was conducted. Before starting the study, the dialysis bag was soaked for 30 min in the phosphate buffer solution (PBS) to make sure it was completely moist (Nahar & Jain, Citation2009). Samples of 1 ml were removed at a predetermined time duration (0.5, 1, 2, 4, 6, 8, 12, and 24 h), and to sustain sink conditions promptly, the same quantity of PBS was added. The speed of the shaker was set at 100 rpm. Utilizing a UV visible spectrophotometer (Model: HALO DB 20, Make: Dynamica, UK), the concentration of the MTF in the samples was taken at the wavelength of 234 nm. Graphs were plotted between the time and % cumulative release of MTF. By running the data through various mathematical models using the DD Solver software, including first order, zero order, Higuchi Hixson-Crowell, and Korsmeyer-Pappas release models, it was possible to calculate the release kinetics of MTF from MTFS, MTF-TES and MTF-TES gel (Chaubey & Mishra, Citation2014; Yuan et al., Citation2022).
2.13. Ex vivo skin permeation study
2.13.1. Skin preparation for the study
Healthy BALB/c mice were euthanized for this experiment utilizing 4% isoflurane inhalation cylinders. This was followed by cardiac ex-plantation of deeply anesthetize animal as per protocols employed by previously published study (Batool et al., Citation2021). The skin of sacrificed mice was taken for the conduction of an ex vivo skin permeation study. Shaving was done from the abdomen to remove hair and the shaved skin was separated. The subcutaneous layer was carefully peeled, and any fat tissues that were still adhering were cleaned by using a cotton bud immersed in isopropyl alcohol. PBS was used to wash the skin, and the skin was then maintained at −20 °C until further process.
2.13.2. Permeation study
A Franz diffusion cell apparatus (consisting of two compartments) was used to perform the ex vivo skin permeation study. The major goal of this experiment was to check the permeation potential of MTF from various test samples. Among the donor chamber and the acceptor compartments, which contained the MTF-TES and the PBS, correspondingly, the removed BALB/c mice skin was placed. In individual cells, the identical configuration was built for MTFS-TES, MTF-TES gel, and plain MTF-loaded gel (MTF-gel). Samples of 1 ml were obtained at specific times (0.25, 0.5 1, 2, 4, 6, 8, 12, and 24 h), and the concentration of MTF was determined by measuring absorbance at the wavelength of 234 using a UV visible spectrophotometer. The cumulative quantity of MTF that permeated through the skin as a function of time was displayed (Zhu et al., Citation2008; Van Staden et al., Citation2020).
2.14. In vivo antidiabetic assay on Sprague Dawley rats
The antidiabetic potential of MTF-TES was determined by evaluating its blood sugar-lowering response in twenty STZ-induced diabetic Sprague Dawley rats. The fasting glucose level and body weight of all the rats were determined using a life care glucometer (Make: Lifecare GmBH) and weight machine (Sharma et al., Citation2010). All the animals were injected with 50 mg/kg/animal sterile solution of STZ in sodium citrate buffer (pH = 4.5), intraperitoneally followed by administration of 5% glucose solution using oral gavage. After 72 h of STZ injection, the fasting glucose level of all the animals was evaluated by pricking the ear vein, and the animals having blood glucose above 200 mg/dl were recruited and randomly divided into 4 experimental groups. Although, initially 20 rats were for the induction of disease, yet some of them didn’t showed the required blood glucose level and thus they were not included in the study. Thus, each group contained 4 Sprague-Dawley rats for this study. Group-1 was the negative control group treated with plain distilled water (placebo). Group-2 was the positive control group and was administered with plain metformin (100 mg/kg/d). However, group-3 was treated with a topical application of MTF-TES gel daily (containing 75 mg/kg/d of MTF per animal). Group-4 was treated with plain TES gel. The treatment was continued for 28 d. On days 7th, 14th, 21st, and 28th, the fasting blood glucose and its effect on body weight during the study course were evaluated (Lari et al., Citation2021). The study protocol is also tabulated in .
Table 2. In-vivo anti-diabetic study treatment and dosing.
2.15. Statistical analysis
All the data presented in this research study are presented as Mean ± S.D. with each experiment performed in triplicate. The formulation was optimized using the Design Expert® (Ver. 12). The TEM micrograph was analyzed by using ImageJ software. Furthermore, the statistical analysis including multiple comparison Tukey’s test, was performed using GraphPad Prism (Ver. 9.0.0) (Chen et al., Citation2017; Opatha et al., Citation2020).
3. Results and discussion
3.1. Fabrication and optimization of MTF-TES
As it was mentioned in the previous section, the Box-Behnken model was applied for the MTF-TES optimization. For this purpose, the Design-Expert® (Ver. 12) software was used. The MTF-TES were prepared by utilizing a thin-film hydration process. The results of all 14 experimental runs (generated by Box-Behnken design) are stated in . In addition, the model characteristic for Box-Behnken design including adequate precision, and adjusted and predicted R2 values are also mentioned in . The impact of the independent variable on the individual response factor (dependent factor) is discussed in the next sub-sections.
Table 3. Formulation chart of MTF-TES obtained by Box-Behnken design and the determined response factors.
Table 4. Summary of the Box-Behnken Design for the optimization of MTF-TES.
3.1.1. Impact of independent factors on MPSD
The MTF-TES vesicle’s MPSD is regarded as being one of the very important characteristics of a transdermal drug delivery system. The MPSD of the MTF-TES was substantially influenced by all the independent factors including, PL 90 G, tween 80®, and the concentration of ethanol with a p-value of <.0001, <.0048, and <.0225, respectively. As it can be observed in , a noticeable increase in particle size was accompanied by a decrease in concentration of tween 80® and an increase in PL 90 G amount. As it is depicted in the graphs, by increasing the concentration of PL 90 G, MPSD increased. This could be because of more bilayer formation ultimately leading to the formation of multilamellar vesicles occurs (Chen et al., Citation2017). Moreover, by increasing the concentration of tween 80® particle size was decreased. This behavior could most probably be because of the adsorption of surfactant on the surface which may prevent the growth of TES (Jamshaid et al., Citation2022). Moreover, it reduces the surface free energy resulting in the reduction in the agglomeration and hence decreases the particle size. As far as the effect of ethanol concentration is concerned, by increasing the concentration of ethanol, the MPSD of MTF-TES was decreased, because it also acts as a co-surfactant and reduces the interfacial tension and hence decreases the particle size (Opatha et al., Citation2020; Akram et al., Citation2022). In conclusion, there is a direct relation between MPSD with the concentration of PL 90 G, and an opposite was observed with an augmented concentration of ethanol and tween 80®.
3.1.2. Impact of independent factors on PDI
It is noteworthy that the PDI of nanoparticles measures their degree of uniformity. The stability of nanoparticles is interconnected with their PDI. A higher PDI (>0.5) is the indicator of non-uniformity which leads to the formation of unstable formulation. There is a marked effect of the concentration of independent factors including PL 90 G (p-value = .0007) and ethanol (p-value = .0196) on PDI. As shown in , a remarkable increase in PDI was linked with an increase in PL 90 G amounts. By increasing the concentration of PL 90 G, PDI increases because there is the formation of more non-uniform size, multilamellar, and dispersed vesicles. Owing to the effect on steric stabilization, ethanol reduces the MPSD and PDI of TES (Albash et al., Citation2019). Conclusively, there is an inverse relationship between the concentrations of ethanol and a direct relationship between PDI and PL 90 G.
3.1.3. Impact of independent factors on ZP
Determining the ZP of MTF-TES is a crucial factor to evaluate its stability owing to the fact that it determines the charge carried by the TES because as the repulsive charges of the formulation increase, its stability increases. All the independent variables; such as PL 90 G, tween 80® except the concentration of ethanol having the p-value of .0027 and <.0001, respectively, had a significant impact on the ZP of the MTF-TES. As shown in , a considerable increase in ZP was associated with a decrease in the concentration of tween 80® and an increase in the concentration of PL 90 G. By increasing the concentration of PL 90 G, ZP increases because the higher lipid content carries a more negative charge (owing to the presence of a negatively charged phosphate group). Furthermore, because of the particles and skin cells’ electrostatic interaction, the drug permeates the skin more readily (Khalid et al., Citation2022). As far as tween 80® concentration is concerned, by increasing the concentration of tween 80®, ZP decreases because the surfactant provide a shielding effect. Regarding the impact of ethanol content, there is no marked effect of the concentration of ethanol on ZP. In a nutshell, ZP directly incremented with the augmentation in the concentration of PL 90 G, but with an enhanced concentration of tween 80®, the results were the opposite i.e. ZP was reduced.
3.1.4. Impact of independent factors on %EE
The percentage of a drug that is successfully entrapped is the main factor for therapeutic effectiveness. %EE of the MTF-TES was markedly impacted by the concentration of PL 90 G, tween 80®, and ethanol, and the p-value was found to be .0012, .0401, and .0296, respectively. As shown in , a prominent increase in %EE was observed with a decreased tween 80® concentration and an increase in PL 90 G amounts. By increasing the concentration of PL 90 G, the %EE was increased, which could be because of the development of large-size particles which ultimately increased the space for the entrapment of the drug. Then, by increasing the concentration of tween 80®, particle size was decreased because the surfactant may get adsorbed on the surface and may prevent the growth of TES. Moreover, with a reduction particle size, less space may be available for the drug to be incorporated. Additionally, the surfactant may cause leakage of the drug from nanoparticles by rupturing them, hence reduced %EE may be observed (Moolakkadath et al., Citation2018). By increasing the concentration of ethanol, the %EE of MTF inside the MTF-TES increases because of the direct increment of MTF solubilization from 10% ethanol to 30% ethanol (Opatha et al., Citation2020). Overall, there is a direct correlation between %EE and PL 90 G as well as ethanol concentration, whereas tween 80® concentrations have an inverse relationship.
3.1.5. Optimized MTF-TES formulation
According to the results of Box-Behnken software, formulation TE7 was optimized with the MPSD value of 165.4 ± 2.3 nm, zeta potential of −21.6 ± 1.9 mV, and PDI of 0.169 ± 0.033, these results are displayed in , and 89.76 of %EE as depicted in , because the response factors were in our desired limits. Furthermore, the summary of the Box-Behnken design is also presented in .
3.2. TEM analysis for surface morphology assessment
Surface morphology, uniformity, and segregation were evaluated through TEM analysis. The fabricated MTF-TES TEM micrograph is depicted in . It revealed that the nanoparticles were spherical with round morphology and intact borders. These outcomes also supported the particle size determined using Zeta Sizer as the ImageJ software has predicted the average particle size of 162.50 nm, from the TEM micrograph.
3.3. FTIR analysis confirmed the drug-excipients chemical compatibility
To check any incompatibility or chemical interaction between the MTF-TES, MTF, PL 90 G, and physical mixture, an FTIR study was performed. According to , the MTF FTIR curve showed peaks at 3336 cm−1 and (C = N) at 1675 cm−1. Similarly, PL 90 G exhibited its peaks of (C = O) at 1739 cm−1. The peaks of both MTF and PL 90 G remained intact in the final formulation as depicted in the figure and no chemical and physical interaction between the formulation and its component was observed. Moreover, all the double and triple bonds of MTF and PL 90 G were reserved in the final formulation and physical mixture. These findings demonstrated the drug-excipient stability of MTF-TES. By this, we can conclude that our preparation is stable, and the biological and physicochemical characteristics of the excipients are preserved (Chen et al., Citation2017).
3.3.1. Characterization of MTF-TES gel
The pH, clarity, viscosity, Spreadability, homogeneity, and drug content of the chitosan-based transethosomal gel (MTF-TES) were evaluated. Chitosan was selected for application to the skin in accordance with the standard criteria defined for gels and in light of the suitable physicochemical qualities (Usman et al., Citation2016). The gel was clear and uniform. An important consideration is to examine the Spreadability test’s flow pattern for chitosan gel. The appropriate Spreadability of MTF-TES gel is crucial for effective topical application and the results have shown that the Spreadability of 336.7 ± 5.8% is appropriate for the topical application of gels. The Brookfield Viscometer was used to measure the flow behavior of the MTF-TES gel. To assess the impact of stress or strain, the chitosan gel’s viscosity was evaluated at various speeds (0.5, 1, 2, 3.5, 4, 5.4, 6, 9.5, 14, 22, 36, 58, 64, and 100 rpm) and flow patterns and the results have shown that the formulation has followed non-Newtonian flow (graph is shown in Figure S1, please refer to the supplementary file). The pH meter was used to determine the MTF-TES gel pH and the results have shown a pH of 5.36 ± 0.06 which is appropriate for the topical application of gels. All the assessed parameters of MTF-TES gel are presented in Table S1 (supplementary file)
3.4. Sustain release pattern of MTF-TES and MTF-TES gel
In vitro release profile of MTF was determined by plotting % cumulative drug release versus time as reported in . Release of MTF was determined from MTF-TES and MTF-TES gel and was compared with MTFS at pH 5.5 and 7.4. At pH 7.4, after 4 h, 93.67 ± 2.65% of the cumulative MTF was released from the MTFS, while only 50.94 ± 3.91% of the drug was released from MTF-TES gel followed by only 63.56 ± 2.08% drug release from MTF-TES. While, after 12 h, only 81.01 ± 2.51% of the cumulative drug was released from MTF-TES and 66.02 ± 3.23% was released from MTF-TES gel. Finally, at 24 hr time point, the MTF release was extended up to 87.43 ± 2.47% by MTF-TES, and 72.18 ± 3.21% MTF was released from MTF-TES gel. The MTF-TES has shown sustained release followed by a more pronounced effect in MTF-TES gel as compared to the MTFS because the ultra-deformable lipid-based nanoparticles exhibit a sustained drug release profile (Jamshaid et al., Citation2022) and by incorporating the drug into this drug delivery system the release of the drug has been delayed and by further loading them into the gel their release was further delayed because of the 3D structure of the gel which holds them for longer period of time as compared to the burst release as shown by the solution (Khan et al., Citation2022). At 5.5 pH, less percent cumulative drug release was occurred in contrast to the drug release observed at pH 7.4. According to the graph in , 95.86 ± 3.51% cumulative MTF was released from the MTFS; owing to the acidic nature of the medium, it showed less drug release as compared to pH 7.4 (where comparable drug release was encountered within 4 h), while only 37.03 ± 4.99% of the drug was released from MTF-TES. However, from MTF-TES gel, the cumulative drug release in the first 6 hr was found to be 22.05 ± 2.40%. The release percentage of the drug was finally extended to 39.59 ± 5.91% and 28.50 ± 3.62% drug release from MTF-TES and MTF-TES gel, respectively. Additionally, the kinetic release mathematical modeling, including Higuchi, Zero-order, Hixson Crowell, Korsmeyer-Peppas, and First order, was applied using a DD-solver (MS Excel Add-Ins). The Korsmeyer-Peppas has been determined as being the most accurate (best fit) release kinetic model at 7.4 pH based on the calculated regression coefficient values. In , the R2 values for each kinetic release model are listed. The ‘n’ value is crucial for the Korsmeyer-Peppas paradigm. Since the ‘n’ number for every both MTF-TFS and MTF-TFS gel was smaller than 0.45, thus it follows release patterns by non-fickian diffusion.
Figure 4. (A) Percent Cumulative MTF release from MTF soln, MTF-TES and MTF-TES gel at pH 7.4. (B) Percent cumulative MTF release from MTF soln, MTF-TES and MTF-TES gel at pH 5.5 (C) ex vivo cutaneous permeation of MTF from MTF soln, MTF-TES and MTF-TES gel.

Table 5. R2 values of MTF release kinetic of various kinetic model.
3.5. Improved ex vivo cutaneous permeation of MTF with MTF-TES and MTF-TES gel
To determine the permeation profiles of MTF under simulated conditions (at pH 7.4; showing blood pH across the skin), an ex vivo skin permeation study was conducted. An Ex vivo skin permeation experiment was used to evaluate the MTF-TES ability to pass the skin’s physical barriers. shows the cumulative cutaneous permeation of MTF from MTF gel, MTF-TES, and MTF-TES gel. As can be seen, MTF-TES has shown enhanced permeation followed by MTF-TES gel as compared to the MTFS. The preliminary cutaneous permeation (from 0.5 to 1 h) of MTF from MTFS, MTF-TES, and MTF-TES gel was nearly negligible. MTF began to penetrate inside the SC barrier after the afore-stated period. In 4 h negligible cumulative drug permeation occurred from the MTFS, however, an increased amount of drug (66.41 ± 11.41 µg/cm2) was permeated from MTF-TES gel. Moreover, a more increased amount of MTF (176.67 ± 15.29 µg/cm2) was permeated from MTF-TES. Upon extension to 24 h, only 177.78 ± 19.65 µg/cm2 of MTF was permeated from the MTFS, whereas, 893.41 ± 66.62 µg/cm2 of the cumulative drug was permeated from MTF-TES. Similarly, 793.94 ± 59.08 of MTF from MTF-TES gel was permeated across the skin barrier. The MTF-TES has shown enhanced permeation followed by a more pronounced effect in MTF-TES gel as compared to the MTFS because the ultra-deformable lipid-based nanoparticles exhibit a deformable nature (Song et al., Citation2012) which can help them in crossing the SC barrier. Another important effect is that the presence of ethanol causes the fluidization of the skin’s stratum corneum lipid molecules and improves the mobility of phospholipid chains to the deeper skin tissues (Mishra et al., Citation2019; Gupta et al., Citation2020). By incorporating the drug into an ultra-deformable system, the permeation of the drug was significantly enhanced as compared to the solution which showed poor permeation (Jamshaid et al., Citation2022). Similar sort of results are shown by the research studies previously carried out (Chen et al., Citation2017; Moolakkadath et al., Citation2018), in which the transethosomal nanocarriers are shown to have markedly incremented drug dermal penetration than the conventional gel systems. Following the calculation of the enhancement ratio, it was determined that the permeation of MTF from MTF-TES and MTF-TES gel was 4.38 and 3.86 times incremented, correspondingly, compared to the MTF gel. The permeation parameters including transdermal flux, enhancement ratio, and permeation coefficient are stated in .
Table 6. MTF cutaneous permeation profile from MTF-gel, MTF-TES and MTF-TES gel.
3.6. MTF-TES gel presented with marked antidiabetic effect in STZ-induced diabetic rats
Post successful optimization and in vitro characterization of MTF-TES gel, its therapeutic efficacy in terms of lowering fasting glucose level (FBG) level was assessed and compared with oral marketed MTF. As illustrated in , at day-0, animals of all the groups were highly hyperglycemic and the treatment was initiated. In both, MTF-TES gel-treated group and the oral MTF-treated group, the FBG started to be lowered as the treatment was initiated. After 28 d of treatment with MTF-TES gel, the FBG level lowered from 557.67 ± 28.50 mg/dl to 104.00 ± 16.70 mg/dl. It can clearly be seen from that results of 75 mg/kg/d of MTF-TES gel has produced better results, in terms of FBG reduction. Unlikely, as obtained with oral MTF after 28 d of treatment even with 100 mg/kg/d of oral MTF, the FBG was reduced to 171.33 ± 24.91 mg/dl, which is significantly higher as compared to the MTF-TES gel treated group. However, in the negative control group and plain TES gel-treated group, there was no significant effect encountered in FBG reduction in rats. STZ-induced diabetic rats were found to be associated with a marked reduction in body weight. The prevention in weight reduction of the animal was determined and compared for each experimental group. MTF-TES gel-treated group was presented with significantly different behavior as observed with the negative control group. After 28 d of treatment with MTF-TES gel the body weight was reduced from 263.33 ± 19.14 g to 232.01 ± 12.49 g, which was significantly high as compared to the oral MTF treated group, which showed weight reduction of 265.75 ± 21.25 g to 193.01 ± 16.51 g (). One of the most interesting findings encountered by the results of the antidiabetic assay was the efficacy of nano-formulation of MTF when compared with marketed oral MTF, even in lower doses. These results demonstrated the fact that the MTF-TES gel has markedly enhanced the transdermal permeation of MTF and produced a significantly variable lowering of FBG levels in comparison to that obtained from oral MTF. The pretext is that the nanoparticles optimize the aqueous solubility of MTF, which probably leads to enhanced retention time and slow excretion from the body (Usman et al., Citation2016). This causes comparable FBG lowering of oral MTF and MTF-TES gel (with low doses of MTF-TES gel).
4. Conclusion
Metformin hydrochloric acid-loaded TES were successfully fabricated with maximum entrapment efficiency and small particle size. MTF-TES were successfully loaded to Chitosan gel. In-vitro release and Ex-vivo permeation confirmed the potential of MTF-TES as a nanocarrier to deliver drug across the skin, with enhanced permeability. The results of in vivo antidiabetic assay demonstrated the significantly improved antidiabetic potential of MTF after its incorporation inside the TES system. By the assessment of all these findings, we can conclude that the MTF-TES gel system can be an optimal alternative for oral MTF tablets, however, further evaluation of pharmacokinetic profiling is required.
Author contributions
Kainat Nousheen, Humzah Jamshaid: Conception, design, investigation and drafting. Rabia Afza, Saif Ullah Khan, Maimoona Malik: Design, analysis and interpretation of the data, intellectual content evaluation. Zakir Ali, Sibgha Batool, Alam Zeb: Design approval, drafting of the paper, visualization, approval of the draft. Abid Mehmood Yousaf, Saud Alqahtani: Methodology, software, validation, revision of the manuscript critically for intellectual content. Salman Khan, Gul Majid Khan: Design, validation, resources, final approval of the version to be published. Fakhar ud Din, Ali H Almari: Conception, design, supervision, funding, final approval for submission. Moreover, all the authors agree to be accountable for all aspects of the work.
Ethical approval
The animal study was performed as per the Guide for the Care and Use of Laboratory Animals (8th Edition) and ARRIVE (Version 2) guidelines, after the approval of Quaid-i-Azam University ethical committee via approval number (BEC-FBS-QAU-2022-385). Further information on animal use is provided in Sections 2.3, 2.13 and 2.14.
Justification for use of animals
The cellular and molecular effects of a therapeutic agent could be predicted using in vitro systems and the usefulness cannot be underemphasized as it allows high throughput rapid screening of candidate compounds. However, a proper understanding of the complex physiological response needs a whole organism that could exhibit the signs and symptoms of diseases. Thus, animal studies have been introduced as a subset of test systems employed at the preclinical stage of drug development for the prediction of clinical effectiveness in humans. This has been possible due to the ability to correlate data generated from animal studies to humans.
Rodents (mice and rats) were used in this study, as they are the most commonly used animal models due to their short lifecycle and lifespan. These offer complementary benefits of cost and time savings as they can reproduce quickly, yielding faster results. Moreover, they have been reported to have 90-95% resemblance with human anatomy. As discussed earlier (Sections 2.3, 2.13 and 2.14) standard operating procedures were adopted to perform animal studies, including the use of minimal number with marginal pain and discomfort.
Supplemental Material
Download MS Word (82.6 KB)Acknowledgement
The authors are thankful to the Higher Education Commission of Pakistan, National institute of Health Islamabad Pakistan and Department of Pharmacy, Quaid-i-Azam University Islamabad Pakistan for their facilitation in conducting this research.
Disclosure statement
The authors declare no potential competing interest.
Data availability statement
The data that support the findings of this study are available from the corresponding author, [Fakhar Ud Din], upon reasonable request.
Additional information
Funding
References
- Aggarwal N, Goindi S, Mehta SD. (2012). Preparation and evaluation of dermal delivery system of griseofulvin containing vitamin E-TPGS as penetration enhancer. AAPS PharmSciTech 13:1–14. doi: 10.1208/s12249-011-9722-y.
- Ahad A, Al-Saleh AA, Al-Mohizea AM, et al. (2018). Formulation and characterization of phospholipon 90 G and tween 80 based transfersomes for transdermal delivery of eprosartan mesylate. Pharm Dev Technol 23:787–93. doi: 10.1080/10837450.2017.1330345.
- Ahad A, Aqil M, Kohli K, et al. (2016). The ameliorated longevity and pharmacokinetics of valsartan released from a gel system of ultradeformable vesicles. Artif Cells Nanomed Biotechnol 44:1457–63. doi: 10.3109/21691401.2015.1041638.
- Ahmed TA, Alzahrani MM, Sirwi A, et al. (2021). the antifungal and ocular permeation of ketoconazole from ophthalmic formulations containing trans-ethosomes nanoparticles. Pharmaceutics 13:151. Study doi: 10.3390/pharmaceutics13020151.
- Akram MW, Jamshaid H, Rehman FU, et al. (2022). Transfersomes: a revolutionary nanosystem for efficient transdermal drug delivery. AAPS PharmSciTech 23:1–18.
- Albash R, Abdelbary AA, Refai H, et al. (2019). Use of transethosomes for enhancing the transdermal delivery of olmesartan medoxomil: in vitro, ex vivo, and in vivo evaluation. Int J Nanomed 14:1953–68. doi: 10.2147/IJN.S196771.
- Amin S, Sarfenejad A, Ahmad J, et al. (2013). Nanovesicular transfersomes for enhanced systemic delivery of telmisartan. Adv Sci Eng Med 5:299–308. doi: 10.1166/asem.2013.1288.
- Bachhav YG, Patravale VB. (2009). Microemulsion based vaginal gel of fluconazole: formulation, in vitro and in vivo evaluation. Int J Pharm 365:175–9. doi: 10.1016/j.ijpharm.2008.08.021.
- Batool S, Zahid F, Ud-Din F, et al. (2021). Macrophage targeting with the novel carbopol-based miltefosine-loaded transfersomal gel for the treatment of cutaneous leishmaniasis: in vitro and in vivo analyses. Drug Dev Ind Pharm 47:440–53. doi: 10.1080/03639045.2021.1890768.
- Berger J, Reist M, Mayer JM, et al. (2004). Structure and interactions in covalently and ionically crosslinked chitosan hydrogels for biomedical applications. Eur J Pharm Biopharm 57:19–34. doi: 10.1016/s0939-6411(03)00161-9.
- Bibi M, Ud Din F, Anwar Y, et al. (2022). Cilostazol-loaded solid lipid nanoparticles: Bioavailability and safety evaluation in an animal model. J Drug Delivery Sci Technol 74:103581. doi: 10.1016/j.jddst.2022.103581.
- Black C, Donnelly P, McIntyre L, et al. (2007). Meglitinide analogues for type 2 diabetes mellitus. Cochrane Database Syst Rev 2007:CD004654. doi: 10.1002/14651858.CD004654.pub2.
- Cesur S, Cam ME, Sayın FS, et al. (2022). Metformin-loaded polymer-based microbubbles/nanoparticles generated for the treatment of type 2 diabetes mellitus. Langmuir 38:5040–51. doi: 10.1021/acs.langmuir.1c00587.
- Cetin M, Atila A, Sahin S, et al. (2013). Preparation and characterization of metformin hydrochloride loaded-Eudragit® RSPO and Eudragit® RSPO/PLGA nanoparticles. Pharm Dev Technol 18:570–6. doi: 10.3109/10837450.2011.604783.
- Chaubey P, Mishra B. (2014). Mannose-conjugated chitosan nanoparticles loaded with rifampicin for the treatment of visceral leishmaniasis. Carbohydr Polym 101:1101–8. doi: 10.1016/j.carbpol.2013.10.044.
- Chen Z, Li B, Liu T, et al. (2017). Evaluation of paeonol-loaded transethosomes as transdermal delivery carriers. Eur J Pharm Sci 99:240–5. doi: 10.1016/j.ejps.2016.12.026.
- Costanzo M, Esposito E, Sguizzato M, et al. (2021). Formulative study and intracellular fate evaluation of ethosomes and transethosomes for vitamin D3 delivery. Int J Mol Sci 22:5341. doi: 10.3390/ijms22105341.
- Dar MJ, Din FU, Khan GM. (2018). Sodium stibogluconate loaded nano-deformable liposomes for topical treatment of leishmaniasis: macrophage as a target cell. Drug Deliv 25:1595–606. doi: 10.1080/10717544.2018.1494222.
- Del Prato S, Pulizzi N. (2006). The place of sulfonylureas in the therapy for type 2 diabetes mellitus. Metabolism 55:S20–S27. doi: 10.1016/j.metabol.2006.02.003.
- Demuth H-U, McIntosh CH, Pederson RA. (2005). Type 2 diabetes—therapy with dipeptidyl peptidase IV inhibitors. Biochim Biophys Acta 1751:33–44. doi: 10.1016/j.bbapap.2005.05.010.
- El-Gizawy SA, Nouh A, Saber S, et al. (2020). Deferoxamine-loaded transfersomes accelerates healing of pressure ulcers in streptozotocin-induced diabetic rats. J Drug Delivery Sci Technol 58:101732. doi: 10.1016/j.jddst.2020.101732.
- Gupta R, Badhe Y, Rai B, et al. (2020). Molecular mechanism of the skin permeation enhancing effect of ethanol: a molecular dynamics study. RSC Adv 10:12234–48. doi: 10.1039/d0ra01692f.
- Hurler J, Engesland A, Poorahmary Kermany B, et al. (2012). Improved texture analysis for hydrogel characterization: gel cohesiveness, adhesiveness, and hardness. J Appl Polym Sci 125:180–8. doi: 10.1002/app.35414.
- Jamshaid H, Malik M, Mukhtiar M, et al. (2022). A cutback in imiquimod cutaneous toxicity; comparative cutaneous toxicity analysis of imiquimod nanotransethosomal gel with 5% marketed cream on the BALB/c mice. Sci Rep 12:14244. doi: 10.1038/s41598-022-18671-1.
- Kamboj VK, Verma PK. (2018). Preparation and characterization of metformin loaded stearic acid coupled F127 nanoparticles. Asian J Pharm Clin Res 11:212–7. doi: 10.22159/ajpcr.2018.v11i8.26444.
- Kenechukwu FC, Nnamani DO, Momoh MA, et al. (2022). Enhanced circulation longevity and pharmacodynamics of metformin from surface-modified nanostructured lipid carriers based on solidified reverse micellar solutions. Heliyon 8:e09100. doi: 10.1016/j.heliyon.2022.e09100.
- Khalid H, Batool S, Din F, et al. (2022). Macrophage targeting of nitazoxanide-loaded transethosomal gel in cutaneous leishmaniasis. R Soc Open Sci 9:220428. doi: 10.1098/rsos.220428.
- Khan AU, Jamshaid H, Ud Din F, et al. (2022). Designing, optimization and characterization of Trifluralin transfersomal gel to passively target cutaneous leishmaniasis. J Pharm Sci 111:1798–811. doi: 10.1016/j.xphs.2022.01.010.
- Kim JH, Song SH, Joo SH, et al. (2022). Formulation of a gastroretentive in situ oral gel containing metformin HCl Based on DoE. Pharmaceutics 14:1777. doi: 10.3390/pharmaceutics14091777.
- Kumar L, Utreja P. (2020). Formulation and characterization of transethosomes for enhanced transdermal delivery of propranolol hydrochloride. MNS 12:38–47. doi: 10.2174/1876402911666190603093550.
- Kumar L, Verma S, Singh K, et al. (2016). Ethanol based vesicular carriers in transdermal drug delivery: nanoethosomes and transethosomes in focus. NanoWorld J 2:41–51. doi: 10.17756/nwj.2016-030.
- Kumar S, Bhanjana G, Verma RK, et al. (2017). Metformin-loaded alginate nanoparticles as an effective antidiabetic agent for controlled drug release. J Pharm Pharmacol 69:143–50. doi: 10.1111/jphp.12672.
- Lari AS, Zahedi P, Ghourchian H, et al. (2021). Microfluidic-based synthesized carboxymethyl chitosan nanoparticles containing metformin for diabetes therapy: In vitro and in vivo assessments. Carbohydr Polym 261:117889. doi: 10.1016/j.carbpol.2021.117889.
- Luft D, Schmülling R, Eggstein M. (1978). Lactic acidosis in biguanide-treated diabetics. Diabetologia 14:75–87. doi: 10.1007/BF01263444.
- Luo C, He D, Yang H, et al. (2022). The effect of liraglutide on renal function in type 2 diabetes: a meta-analysis of randomized controlled studies. Afr Health Sci 22:267–74. doi: 10.4314/ahs.v22i3.28.
- Majumder J, Taratula O, Minko T. (2019). Nanocarrier-based systems for targeted and site specific therapeutic delivery. Adv Drug Deliv Rev 144:57–77. doi: 10.1016/j.addr.2019.07.010.
- Migdadi EM, Courtenay AJ, Tekko IA, et al. (2018). Hydrogel-forming microneedles enhance transdermal delivery of metformin hydrochloride. J Control Release 285:142–51. doi: 10.1016/j.jconrel.2018.07.009.
- Mishra KK, Kaur CD, Verma S, et al. (2019). Transethosomes and nanoethosomes: Recent approach on transdermal drug delivery system. Nanomedicine 2:33–54.
- Momoh M, Kenechukwu F, Attama A. (2013). Formulation and evaluation of novel solid lipid microparticles as a sustained release system for the delivery of metformin hydrochloride. Drug Deliv 20:102–11. doi: 10.3109/10717544.2013.779329.
- Moolakkadath T, Aqil M, Ahad A, et al. (2018). Development of transethosomes formulation for dermal fisetin delivery: Box–Behnken design, optimization, in vitro skin penetration, vesicles–skin interaction and dermatokinetic studies. Artif Cells Nanomed Biotechnol 46:755–65. doi: 10.1080/21691401.2018.1469025.
- Nahar M, Jain NK. (2009). Preparation, characterization and evaluation of targeting potential of amphotericin B-loaded engineered PLGA nanoparticles. Pharm Res 26:2588–98. doi: 10.1007/s11095-009-9973-4.
- Nayak D, Tawale RM, Aranjani JM, et al. (2020). Formulation, optimization and evaluation of novel ultra-deformable vesicular drug delivery system for an anti-fungal drug. AAPS PharmSciTech 21:140. doi: 10.1208/s12249-020-01681-5.
- Opatha SAT, Titapiwatanakun V, Chutoprapat R. (2020). Transfersomes: A promising nanoencapsulation technique for transdermal drug delivery. Pharmaceutics 12:855. doi: 10.3390/pharmaceutics12090855.
- Patil S, Kadam A, Bandgar S, et al. (2015). Formulation and evaluation of an in situ gel for ocular drug delivery of anticonjunctival drug. Cellul Chem Technol 49:35–40.
- Patiño-Herrera R, Louvier-Hernández JF, Escamilla-Silva EM, et al. (2019). Prolonged release of metformin by SiO2 nanoparticles pellets for type II diabetes control. Eur J Pharm Sci 131:1–8. doi: 10.1016/j.ejps.2019.02.003.
- Rabia S, Khaleeq N, Batool S, et al. (2020). Rifampicin-loaded nanotransferosomal gel for treatment of cutaneous leishmaniasis: passive targeting via topical route. Nanomedicine 15:183–203. doi: 10.2217/nnm-2019-0320.
- Rizvi SZH, Shah FA, Khan N, et al. (2019). Simvastatin-loaded solid lipid nanoparticles for enhanced anti-hyperlipidemic activity in hyperlipidemia animal model. Int J Pharm 560:136–43. doi: 10.1016/j.ijpharm.2019.02.002.
- Rostamkalaei SS, Akbari J, Saeedi M, et al. (2019). Topical gel of Metformin solid lipid nanoparticles: a hopeful promise as a dermal delivery system. Colloids Surf B Biointerfaces 175:150–7. doi: 10.1016/j.colsurfb.2018.11.072.
- Saini N, Sodhi RK, Bajaj L, et al. (2016). Intravaginal administration of metformin hydrochloride loaded cationic niosomes amalgamated with thermosensitive gel for the treatment of polycystic ovary syndrome: in vitro and in vivo studies. Colloids Surf B Biointerfaces 144:161–9. doi: 10.1016/j.colsurfb.2016.04.016.
- Salim MW, Shabbir K, Yousaf AM, et al. (2020). Preparation, in-vitro and in-vivo evaluation of rifampicin and vancomycin co-loaded transfersomal gel for the treatment of cutaneous leishmaniasis. J Drug Delivery Sci Technol 60:101996. doi: 10.1016/j.jddst.2020.101996.
- San Tang K. (2019). The current and future perspectives of zinc oxide nanoparticles in the treatment of diabetes mellitus. Life Sci 239:117011. doi: 10.1016/j.lfs.2019.117011.
- Sankhyan A, Pawar PK. (2013). Metformin loaded non-ionic surfactant vesicles: optimization of formulation, effect of process variables and characterization. Daru 21:7. doi: 10.1186/2008-2231-21-7.
- Sharma U, Sahu R, Roy A, et al. (2010). In vivo antidiabetic antioxidant potential of Stephania hernandifolia in streptozotocin-induced-diabetic rats. J Young Pharm 2:255–60. doi: 10.4103/0975-1483.66803.
- Soccio RE, Chen ER, Lazar MA. (2014). Thiazolidinediones and the promise of insulin sensitization in type 2 diabetes. Cell Metab 20:573–91. doi: 10.1016/j.cmet.2014.08.005.
- Song CK, Balakrishnan P, Shim C-K, et al. (2012). A novel vesicular carrier, transethosome, for enhanced skin delivery of voriconazole: characterization and in vitro/in vivo evaluation. Colloids Surf B Biointerfaces 92:299–304. doi: 10.1016/j.colsurfb.2011.12.004.
- Ternullo S, Schulte Werning LV, Holsæter AM, et al. (2019). Curcumin-in-deformable liposomes-in-chitosan-hydrogel as a novel wound dressing. Pharmaceutics 12:8. doi: 10.3390/pharmaceutics12010008.
- Torabian F, Akhavan Rezayat A, Ghasemi Nour M, et al. (2022). Administration of silver nanoparticles in diabetes mellitus: a systematic review and meta-analysis on animal studies. Biol Trace Elem Res 200:1699–709. doi: 10.1007/s12011-021-02776-1.
- Udokang N, Udobang J, Ekpenyong C, et al. (2012). Oral administration of aqueous leaf extract of Ocimum gratissimum ameliorates polyphagia, polydipsia and weight loss in streptozotocin-induced diabetic rats. Am J Med Med Sci 2:45–9.
- Usman F, Javed I, Hussain SZ, et al. (2016). Hydrophilic nanoparticles packed in oral tablets can improve the plasma profile of short half-life hydrophobic drugs. RSC Adv 6:94896–904. doi: 10.1039/C6RA11799F.
- Van De Laar FA, Lucassen PL, Akkermans RP, et al. (2005). α-Glucosidase inhibitors for patients with type 2 diabetes: results from a cochrane systematic review and meta-analysis. Diabetes Care 28:154–63. doi: 10.2337/diacare.28.1.154.
- Van Staden D, Du Plessis J, Viljoen J. (2020). Development of a self-emulsifying drug delivery system for optimized topical delivery of clofazimine. Pharmaceutics 12:523. doi: 10.3390/pharmaceutics12060523.
- Verma ML, Kumar S, Das A, et al. (2020). Chitin and chitosan-based support materials for enzyme immobilization and biotechnological applications. Environ Chem Lett 18:315–23. doi: 10.1007/s10311-019-00942-5.
- Yuan M, Niu J, Xiao Q, et al. (2022). Hyaluronan-modified transfersomes based hydrogel for enhanced transdermal delivery of indomethacin. Drug Deliv 29:1232–42. doi: 10.1080/10717544.2022.2053761.
- Zhu W, Yu A, Wang W, et al. (2008). Formulation design of microemulsion for dermal delivery of penciclovir. Int J Pharm 360:184–90. doi: 10.1016/j.ijpharm.2008.04.008.