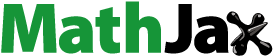
Abstract
Oral cancer is one of the leading causes of death worldwide. Oral precancerous lesions (OPL) are the precursors of oral cancer, with varying degrees of progression. Tetrahydrocurcumin (THC) is a major metabolite of curcumin with superior anticancer properties against various types of cancer. However, THC’s clinical outcome is limited by its poor aqueous solubility. Herein, we developed novel mucoadhesive biopolymer-based composite sponges for buccal delivery of THC, exploiting nanotechnology and mucoadhesion for efficient prevention and treatment of oral cancer. Firstly, THC-nanocrystals (THC-NC) were formulated and characterized for subsequent loading into mucoadhesive composite sponges. The anticancer activity of THC-NC was assessed on a human tongue squamous carcinoma cell line (SCC-4). Finally, the chemopreventive activity of THC-NC loaded sponges (THC-NC-S) was examined in DMBA-induced hamster OPL. The selected THC-NC exhibited a particle size of 532.68 ± 13.20 nm and a zeta potential of −46.08 ± 1.12 mV. Moreover, THC-NC enhanced the anticancer effect against SCC-4 with an IC50 value of 80 µg/mL. THC-NC-S exhibited good mucoadhesion properties (0.24 ± 0.02 N) with sustained drug release, where 90% of THC was released over 4 days. Furthermore, THC-NC-S had a magnificent potential for maintaining high chemopreventive activity, as demonstrated by significant regression in the dysplasia degree and a decline in cyclin D1 (control: 40.4 ± 12.5, THC-NC-S: 12.07 ± 5.2), culminating in significant amelioration after 25 days of treatment. Conclusively, novel THC-NC-S represent a promising platform for local therapy of OPL, preventing their malignant transformation into cancer.
1. Introduction
Oral and oropharyngeal cancer are pathologies of public health concern, representing the sixth most common malignancies with approximately 500,000 cases globally (Alqahtani et al., Citation2020). Furthermore, the 5-year mortality rate for oral cancer is close to 50% (Roi et al., Citation2020). Oral squamous cell carcinoma (OSCC) accounts for over 90% of these cancers (Gangwar et al., Citation2022). Most OSCC arise from precancerous lesions in the oral mucosal lining (Oyapero et al., Citation2020; Habibi et al., Citation2023). Oral precancerous lesions (OPL) are morphologically altered tissues in which oral cancer is more likely to occur; they often present as either white or red patches, known as leukoplakia and erythroplakia, respectively (Shaji et al., Citation2022). OPL has gained attention because of the increased risk of cancer development in the oral cavity. It has been reported that 15.8–48.0% of patients with OSCC have a history of oral leukoplakia (Ou et al., Citation2022).
The main treatments for OSCC are surgery, radiotherapy, and chemotherapy, either alone or in combination (Nandini et al., Citation2020). Whereas, OPL has a number of treatment options including drug therapy, surgery, and laser ablation; none of them are curative (Chen et al., Citation2021). Therefore, continued research for safer and more efficient chemoprevention and treatment is clearly needed to improve efficiency and reduce treatment costs (H. Wang et al., Citation2012). Cancer chemoprevention through OPL management is a potential strategy to enhance quality of life, prevent malignant transformation, and dramatically decrease mortality rates (Ou et al., Citation2022). However, oral cavity physiology can represent a real barrier for effective drug accumulation (Freag et al., Citation2018). Therefore, conventional topical approaches may fail to ensure adequate drug delivery for effective prevention and treatment of oral cancer. Herein, local mucoadhesive drug delivery systems offer high drug concentrations in the affected tissue with limited systemic toxicity for oral cancer prevention and treatment. Meanwhile, a mucoadhesive delivery system is necessary for prolonged as well as close contact between the drug and affected buccal mucosa (Freag et al., Citation2018).
Recently, sponges have been extensively studied as promising platforms for buccal drug delivery. Unlike buccal gels that drain easily after applying, sponges can keep their swollen gel structure, allowing a longer residence time and more efficient therapy. Additionally, sponges have a higher drug loading capability than thin films because of their high surface to volume ratio and porous nature (Ossama et al., Citation2021). Biopolymer-based sponges have additional advantages such as abundance, biodegradability, safety, biocompatibility, and cost-effectiveness (Rudhziah et al., Citation2019; Yanteng Zhao et al., Citation2019; Panatarani et al., Citation2023; Peleg-Evron et al., Citation2023). Amongst these biopolymers, seaweed kappa-carrageenan (κc) is an anionic polymer and consists of linear-sulfated polysaccharides of D-galactose and 3, 6-anhydro-D-galactose () (Keshk et al., Citation2023). One of its most important characteristics is mucoadhesiveness through hydrogen bond interaction with the mucus layer (Yermak et al., Citation2020). The synergistic effect between different polymers is attractive due to their potential to improve and control the rheological properties of dosage forms (Balasubramanian et al., Citation2018). Interestingly, κc can be used either alone or combined with other polymers such as chitosan, pectin, xanthan gum (XG), gellan gum, and hydroxypropylmethyl cellulose (HPMC), yielding soft, elastic, and flexible gels. XG is a promising mucoadhesive biopolymer, it has polyanionic properties due to carboxylic groups and has very good bioadhesive strength () (Yahoum et al., Citation2023). The combination of κc with XG showed advantageous properties regarding mucoadhesion and sustained drug release. κc/XG combination was utilized in mucoadhesive vaginal gels (Andrade et al., Citation2014); nevertheless, it has not been investigated in buccal delivery yet.
Figure 1. Chemical structures of (a) κc and (b) XG, (c) Schematic Illustration of the preparation of THC-S and THC-NC-S.

Tetrahydrocurcumin (THC), a polyphenolic compound, is the main metabolite of curcumin (Yu et al., Citation2023). THC demonstrates curcumin-like therapeutic activities, with superior antioxidant (Gao et al., Citation2022), anti-inflammatory (Lin et al., Citation2022), anti-diabetic (Tsai et al., Citation2021), and anti-aging effects (Xu et al., Citation2022). Additionally, it is a chemopreventive agent against various malignancies (Lai et al., Citation2020; Tang et al., Citation2021). Furthermore, it possesses anticancer activity against breast cancer (Truong et al., Citation2022), cervical cancer (Yoysungnoen et al., Citation2015), colon cancer (Lai et al., Citation2011), liver cancer (W. Liu et al., Citation2017), lung cancer (Song et al., Citation2018), and osteosarcoma (Y. Zhang et al., Citation2017).
Several molecular targets of THC have been identified, including targets that make it effective against cancers such as anti-proliferative activity by cell cycle arrest and decreasing proliferative molecule (cyclin D1, PCNA), anti-oxidative activity by increasing radical scavenging activity and antioxidant enzyme (Miranda et al., Citation2023), inhibition of metastasis by decreasing invasion, migration, cell adhesion and angiogenesis, induction of programmed cell death by increasing apoptosis and autophagy and increase of immune function by increasing phagocytosis and NK cells activity (Lai et al., Citation2020).
Although, THC has better stability than curcumin in physiological condition (Zhu et al., Citation2023), its therapeutic applications are limited by its low aqueous solubility, which is a key process for bioaccessibility (Pandey et al., Citation2020; Loron et al., Citation2021; Jongjitphisut et al., Citation2023). Several researchers developed various systems to increase THC aqueous solubility, such as solid lipid nanoparticles (Saini et al., Citation2022), nanostructured lipid carriers (Truong et al., Citation2022), nanoemulsions (Tang et al., Citation2021), nanofiber (Ravikumar et al., Citation2018), polymer drug conjugate (Tang et al., Citation2021) and inclusion complexes (Loron et al., Citation2021).
Nanocrystals (NC) have the potential for high drug loading and ease of preparation without the need for carriers or a high surfactant content (Q. Wang, Yang, et al., Citation2022; Paredes da Rocha et al., Citation2023). NC achieve therapeutically relevant bioavailability by increased rates of dissolution and saturation velocity as well as improving adhesiveness to cell membranes (X. Zhang et al., Citation2020; Lopez-Vidal et al., Citation2022). Thus, it’s a fundamental issue to develop integrated systems for local THC delivery capable of controlling drug release inside the oral cavity for prolonged periods with high accumulation into the affected tissues to fulfill potential oral cancer prevention and treatment.
In the present study, for the first time, we aimed to develop κc/XG mucoadhesive nanocomposite sponge loaded with THC nanocrystals (THC-NC) as an innovative buccal mucoadhesive dosage form to enhance THC’s chemopreventive and anticancer activities against oral cancer via local treatment. Gathering the privileges of cell adhesion and drug availability, which are represented by NC, and prolonging residence time, which is achieved by mucoadhesive sponges, is expected to improve THC efficacy. A complete characterization of the developed THC-NC and THC-NC loaded sponges (THC-NC-S) was performed. THC-NC’s cytotoxic effects against the human tongue squamous carcinoma cell line (SCC-4) were evaluated. Additionally, biological chemopreventive activity of THC-NC-S was assessed on a DMBA-induced OPL in hamster model using clinical, histological and immunohistochemical parameters.
2. Materials and methods
2.1. Materials
THC (98%) was purchased from Carbosynth Ltd. (Compton, UK). κc and XG powder were commercially obtained from Tokyo Chemical Industry Co., Ltd. (Tokyo, Japan). Hydroxypropyl methylcellulose (HPMC E15, HPMC K4M and HPMC K15M), polyvinyl alcohol (PVA, 98 KDa) and methylcellulose (MC) were purchased from Zhengzhou Tianying Chemicals Co., Ltd. (Yixing, China). Polyvinyl pyrrolidone K25 (PVP-K25, 30 KDa), poloxamer 188 (PLX-188) and poloxamer 407 (PLX-407) were obtained from BASF (Ludwigshafen, Germany). Oral squamous cell carcinoma (OSCC) was obtained from the American Type Culture Collection (ATCC). 3-[4, 5-dimethylthiazol-2-yl]-2, 5-diphenyl tetrazolium bromide (MTT) was purchased from Serva (Heidelberg, Germany). Penicillin and streptomycin solutions (100 U/mL each) were purchased from BioWhittaker® (Lonza, Belgium). The carcinogen 7, 12-Dimethylbenz[a]anthracene (DMBA; > 95%), Dulbecco’s modified eagle medium (DMEM), Fetal Bovine Serum (FBS) and bovine serum albumin were purchased from Sigma Aldrich Chemie GmbH (Steinheim, Germany). Cyclin D1 was purchased from Thermo Scientific (Waltham, MA, USA). Absolute ethanol, sorbitol, and mannitol were obtained from ADWIC (Cairo, Egypt). All other reagents and chemicals are analytical grade.
2.2. Preparation of tetrahydrocurcumin nanocrystals (THC-NC)
THC-NC were prepared using an antisolvent precipitation-ultrasonication technique (Chavan et al., Citation2020). Briefly, 40 mg of THC was dissolved in 1 mL of absolute ethanol (solvent phase). THC solution was then poured dropwise into 20 mL of distilled water containing different stabilizers (PVP-K25, PLX-188, and PVA) as an antisolvent phase, with continuous magnetic stirring at 1200 rpm in an ice bath for 1 min. Afterwards, the dispersion was sonicated at 4 °C under different conditions in terms of sonication amplitude and time (Sonoplus HD 3100; BANDELIN, Berlin, Germany). Different formulation variables, including stabilizer type, THC to stabilizer ratio, sonication amplitude, sonication time, and solvent to antisolvent (S to AS) ratio were screened (see in Supplementary Table S1). The composition and characterization parameters of THC-NC formulations were presented in .
Table 1. Composition and characterization of THC-NC formulations.
2.3. Lyophilization of THC-NC
Selected THC-NC was subjected to lyophilization utilizing a lyophilizer (Cryodos-50; Telstar, S.A., Terrassa, Spain) at −55 °C and 0.4 mbar for 24 h, using mannitol (0, 3%, and 5% w/v) as cryoprotectant to examine the effect of cryoprotectant content on the reproducibility of THC-NC after reconstitution.
2.4. Characterization of THC-NC
2.4.1. Particle size (PS), polydispersity index (PDI), and zeta potential (ZP)
PS, PDI, and ZP of THC-NC were measured for fresh formulations as well as lyophilized formulations after reconstitution by Dynamic light scattering (DLS) technique using Malvern Zetasizer NanoZS (Malvern Instruments, UK). Dispersions were 30-fold diluted with filtered distilled water before analysis. All samples were measured in triplicates and results were represented as mean value ± standard deviation (SD).
2.4.2. Scanning electron microscopy (SEM)
The surface morphology of THC coarse powder and lyophilized THC-NC were visualized utilizing SEM microscopy (100 CX; JEOL, Tokyo, Japan) at different magnification levels (×300 and ×11,000). Samples were fixed on metal supports and coated with gold palladium under an argon atmosphere, and images were acquired using i-scan 2000 software.
2.4.3. Solid state properties
The thermal behaviors of THC, lyophilized THC-NC, and its physical mixture (THC, PLX-188, and PVP K-25) were assessed using Differential scanning calorimetry (DSC) (DSC-6, Shimadzu, Tokyo, Japan). The sealed samples were heated at a constant rate of 10 °C/min from 25 to 200 °C under nitrogen at a flow rate of 60 mL/min. The crystalline state of THC and lyophilized THC-NC were evaluated using a powder X-ray diffractometer (PXRD) (XRD7000; Shimadzu, Japan). Cu Kα1 radiation and step scan models were 30 kV and 30 mA, respectively. Diffractograms were plotted in the angular range of 1°–100° with a 0.02° step size. In order to investigate possible interactions between THC-NC components, Fourier transform infrared spectroscopy (FTIR) study was performed. FTIR spectra of THC, PVP K-25, PLX-188, and lyophilized THC-NC were measured by an FTIR spectrometer (Cary 630, Agilent, USA). Samples (2–4 mg) were positioned to cover the diamond window and scanned between 700 and 4000 cm−1. Spectra obtained represent a mean of 20 individual scans of 2 cm−1 spectral resolution.
2.4.4. Stability study
Selected lyophilized THC-NC formulation was stored at 4 °C for 10 months. It was analyzed with respect to PS, PDI and ZP after reconstitution.
2.5. Preparation of THC loaded sponges (THC-S) and THC-NC loaded sponges (THC-NC-S)
Sponges were prepared employing a simple casting/freeze-drying technique (Kassem et al., Citation2015) with slight modifications. Herein, different polymers (κc, XG, HPMC K4M, and HPMC K15M) were used either alone or combined at different ratios, as shown in . Firstly, polymers were dispersed in hot distilled water at 70 °C under continuous stirring till yielding a homogenous hydrogel. Afterwards, before drug loading, the temperature was reduced to 35 °C to maintain NC integrity (Z. Zhang et al., Citation2016). THC or lyophilized THC-NC were added to the hydrogel with continuous stirring. The final concentrations of THC and total polymers in the hydrogel were kept at 0.1% and 1% w/v, respectively. Sorbitol (SL, 0.5% w/v) was added as a plasticizer. Finally, to obtain sponges, casting hydrogel was poured into circular molds (13 mm diameter and 4 mm depth), frozen at −20 °C for 24 h followed by lyophilization at −55 °C under 0.4 mbar for 24 h (). Plain sponges were prepared without drug addition, as previously mentioned. The compositions of different sponges were declared in .
Table 2. Composition of different sponge formulations.
2.6. Characterization of THC-S and THC-NC-S
2.6.1. Physical appearance
The plain sponges were physically evaluated regarding shape, flexibility, brittleness, separation from, or stickiness to the mold walls, and integrity. Sponges with acceptable physical properties were subjected to further characterization.
2.6.2. SEM
The external surface and cross section of the selected plain sponges, THC-NC-S, and THC-S were examined by SEM (100 CX; JEOL, Tokyo, Japan) at different magnification levels (×50, ×85 and ×11,000). Samples were prepared as aforementioned in Section 2.4.2.
2.6.3. Folding endurance
Determination of folding endurance was done by repeatedly folding one sponge with 3.8 ± 0.2 mm thickness and 12.7 ± 0.3 mm diameter in the same place until it broke, cracked, or had been folded 300 times. The number of times that the sample could be folded in the same place without breaking or cracking was considered folding endurance (Momin et al., Citation2016).
2.6.4. Porosity
The porosity of the selected sponges was determined in terms of total pore volume (Atia et al., Citation2019; Ossama et al., Citation2021) using EquationEquation 1(1)
(1) :
(1)
(1)
where the theoretical and practical volumes of the sponge were calculated from EquationEquations 2
(2)
(2) and Equation3
(3)
(3) , respectively:
(2)
(2)
(3)
(3)
Knowing that m and ρ were the mass and the density of each component/sponge. The practical volume of sponge was calculated using the sponge’s dimensions measured with a micrometer.
2.6.5. Swelling behavior
Swelling behavior of the selected sponges was assessed by measuring their water uptake potential. Sponges were immersed in 40 mL of phosphate buffer (PB, pH 6.8) at 37 °C. The sponges were removed at predefined time intervals (50, 100, 150, 200, 250, and 300 min) and weighed after blotting excess water on filter paper (Youssef et al., Citation2021). The percentage swelling was calculated from EquationEquation 4(4)
(4) :
(4)
(4)
where Wd was the dry weight and Ww was the weight after immersion in PB.
2.6.6. Ex vivo mucoadhesion assessment
Mucoadhesive properties of the selected plain sponges and THC-NC-S to fresh chicken pouch membrane were tested using the texture analyzer CT3 (Brookfield, Middleboro, USA) equipped with a 5 kg load cell (Mohammed et al., Citation2022; Pérez Zamora et al., Citation2022). The sponge was attached to the cylindrical probe using double-sided adhesive tape. The chicken pouch was equilibrated for 15 min at 37.0 ± 0.5 °C before placing over the tissue holder accessory of texture analyzer. A magnetic stirrer was placed on the base of the accessory, and the whole accessory was placed in a beaker filled with PB (pH 6.8) at 37 °C. The probe with attached sponge was then moved downward to contact with soaked tissue at a specific applied load (50 gm) and maintained for specified time in contact (120 sec). The probe was subsequently withdrawn at a specified test speed. The strength required to separate the sponge from the tissue was measured in (gm). The mucoadhesive force in newton (N) units was then calculated from EquationEquation 5(5)
(5) :
(5)
(5)
2.6.7. Solid state properties
FTIR spectra of κc, XG, SL, and selected THC-NC-S were recorded using an FTIR spectrometer. Additionally, the thermal behavior of THC-S, THC-NC-S, and their physical mixtures were analyzed using DSC analysis, as described in Section 2.4.3.
2.6.8. Drug content
Individual sponges (n = 6) of THC-S and THC-NC-S were placed in a glass beaker containing 30 mL of methanol. After which, the mixture was sonicated and filtered. The THC concentration was quantified spectrophotometrically at 282 nm. The actual amount of THC in the sponge was determined based on the data obtained against a predetermined calibration curve of THC in methanol (r = 0.999045). THC content was calculated from EquationEquation 6(6)
(6) :
(6)
(6)
2.6.9. In vitro release study
To select a suitable release medium, solubility of THC in different media (distilled water, PB (pH 6.8) and PB (pH 6.8) with different sodium lauryl sulfate (SLS) concentrations (0.25% and 0.5%)) was done by the shake flask method, as previously reported by Elnaggar et al. (Elnaggar et al., Citation2017; Shehata et al., Citation2022). A membraneless method was used to evaluate THC release profiles from different sponge formulations compared to THC-NC (Atia et al., Citation2019). A sponge containing 0.5 mg of THC was placed in a stainless-steel cup (15 mm diameter and 5 mm depth) and covered with polyester gauze to prevent gel escape without interfering with drug release. The gauze was fixed to the cup through a specially designed stainless-steel ring. The cup was placed in 20 mL of PB (pH 6.8) containing 0.25% SLS to achieve sink conditions (Mahajan et al., Citation2023). Then, beakers were placed in horizontal shaking water bath at 100 rpm, and 37 ± 0.5 °C. At predetermined intervals, 1 mL samples were removed and replaced with an equal volume of fresh release media equilibrated at the same temperature. The released THC concentration was analyzed spectrophotometrically at 280 nm against a blank medium. The correction of release medium dilution was taken into consideration.
2.7. Cell line study
A human tongue squamous carcinoma cell line (SCC-4) (ATCC® CRL-1624™) was employed for assessment of the anticancer activity of the developed THC-NC. Experiments were performed at the CERRMA (Center of Excellence for Research in Regenerative Medicine and its Applications), faculty of medicine, Alexandria University, Egypt. Cells were grown in Dulbecco’s modified eagle medium (DMEM)-high glucose with L-glutamine, enriched with 10% v/v fetal bovine serum (FBS) and antibiotics (100 U/mL penicillin and 100 μg/mL streptomycin). Cells were kept at 37 °C in an incubator with a 5% CO2 atmosphere.
2.7.1. Cellular cytotoxicity assay
Cellular cytotoxicity was assessed using the MTT assay (Shehata et al., Citation2022). Blue formazan crystals with maximum absorbance at 570 nm were produced from the reduction of MTT (a yellow compound) in the mitochondria of viable cells. SCC-4 cells were seeded at a density of 5*103 cells/well in a 96-well plate (Corning Costar Corp., MA, USA) with 100 µL of culture media containing 10% FBS. Cells were permitted to adhere for 48 h, followed by treatment with different concentrations (10–150 µg/mL) of free THC in DMSO (Free THC) and (3–100 µg/mL) of THC-NC. After 24 h of incubation, 100 µL of fresh media encompassing 10 µL MTT solution (5 mg/mL) were added and incubated for 4 h in a CO2 incubator. Finally, media were removed and formazan crystals were dissolved in 100 µL DMSO. Absorbance was recorded at 570 nm using a microplate reader. The viability of cells was calculated using EquationEquation 7(7)
(7) :
(7)
(7)
where control cells were treated with culture media only. Moreover, a DMSO control group was also tested to exclude the effect of this solvent on cell viability. The cellular cytotoxicity of different concentrations was expressed as % cell viability against concentration and used to calculate the IC50 (concentration required to kill 50% of the cells) using GraphPad Prism 7 software. Results were expressed as mean ± SD (n = 6). Detailed information regarding cellular cytotoxicity assay could be found in Supplementary data.
2.8. In vivo study
An in vivo study was performed to evaluate the therapeutic potential of the developed formulations on OPL to assess the extent of chemoprevention in suppressing cancer development.
2.8.1. Experimental animals
Sixty-four Albino Syrian male hamsters (Mesocricetus auratus) five weeks old with an average weight of 80–120 g, were obtained from VACSERA, Cairo, Egypt. The hamsters were acclimatized for two weeks in the Animal House Unit of Medical Research Institute, Alexandria University. They were lodged in show box cages (Technoplast, Italy), one per cage, at a room temperature of 23 ± 1 °C with a relative humidity of 50 ± 5% and a 12 h light/dark cycle (Abdel Hamid et al., Citation2021). All procedures were conducted according to the established principles of animal experiments in the UK Directive of 1986; 86/609/EEC. Experimental protocols were approved by the Alexandria University Ethics Committee (IRBNO:00010556-IORG0008839).
2.8.2. Study design and induction of the OPL
The animal study’s grouping and methodology were presented in , illustrating the sequential procedures. The OPL were induced chemically using the chemical carcinogen 7, 12-Dimethylbenz[a]anthracene (DMBA) carcinogenesis model, where the hamsters’ left buccal pouches (HBPs) were painted with paraffin oil of a 0.5% w/v DMBA concentration using a brush number 4. This step was repeated 3 times per week for 14 weeks or upon observing clinical changes of the oral mucosa (M. Liu et al., Citation2021; Alqalshy et al., Citation2022).
After developing OPL, the hamsters were randomly split into four main cohorts (16 animals each) according to the proposed treatment: untreated as negative control, blank receiving plain sponge, THC-S treated, and THC-NC-S treated groups. Each cohort was further divided according to the duration of treatment into 10 days and 25 days intervals. Each group received the proposed treatment topically: one sponge (0.5 mg THC, 3.8 ± 0.2 mm thickness, and 12.7 ± 0.3 mm diameter) daily for the predetermined treatment intervals. During therapeutic application, animals were lightly sedated by intraperitoneal (IP) injection of a low-dosed combination of 0.1 mL/100 g ketamine hydrochloride and 0.05 mL/100 g xylazine hydrochloride. Upon completion of treatment periods, hamsters were sacrificed by an overdose of ketamine hydrochloride (>30 mg/kg), given IP. Cautious assessment of animal was then performed to confirm death and the remains of animals’ bodies were handled by special authorities (Council, Citation2010). The HBPs were extracted and processed for different histological methods of assessment (Abdel Hamid et al., Citation2021; Essawy et al., Citation2021). All animal experiments were performed following the ARRIVE guidelines.
2.8.3. Histopathological analysis
After sacrifice, the HBPs were dissected, and the tissue biopsies were immersed in 10% formaldehyde for fixation and stained with hematoxylin and eosin (H&E) to visualize the presence of dysplastic criteria in the epithelial lining. Sections were examined in randomly picked microscopic fields under light microscopy (Olympus BX41).
2.8.4. Immunohistochemical and morphometric analysis
To test the effect of THC-NC on the cell cycle progression, we stained the tissue sections with cyclin D1 using a 1:100 dilution ratio of rabbit monoclonal anti-cyclin D1 antibody. Immunohistochemical staining was done using the labeled streptavidin-biotin method (Shin et al., Citation1995). Evaluation was done by two different pathologists in randomly picked microscopic fields at ×400 magnification power. The intensity of the cyclin D1 immunostaining was calculated as the mean area percentage in five different representative microscopic fields. Data were analyzed quantitatively using ImageJ 1.46 r software.
2.9. Statistical analysis
All in-vitro data were presented as the mean of triplicates ± SD and analyzed using Student’s t-test (SPSS software, version 20; IBM, Chicago, IL, USA). Cell line data were presented as the mean of six replicates ± SD. In vivo data were analyzed by one-way ANOVA utilizing the Tukey multiple comparisons test at a significance level of p < .05 (GraphPad Prism software, version 7).
3. Results and discussion
The current study aimed at developing a novel mucoadhesive nanoplatform to improve THC buccal availability for amelioration of OPL to suppress cancer development and progression and for cancer therapy as well. THC-NC was first prepared by an antisolvent precipitation-ultrasonication technique exhibiting good physicochemical attributes. After that, THC-NC loaded mucoadhesive sponges were developed based on natural biopolymers.
3.1. Preparation and Evaluation of THC-NC
Several THC-NC formulations were prepared for selecting the process parameters (sonication amplitude/time and S to AS ratio) and material attributes (stabilizer type and concentration) in an attempt to find the most suitable THC-NC formulation for subsequent loading into the mucoadhesive biopolymer-based sponge. Formulations were compared in terms of PS, PDI and ZP.
3.1.1. Process parameters
To prepare NC, the proper design of the experiment was inescapable, so process parameters should be cautiously adjusted to support nanoscale production. Herein, the effect of different process conditions including sonication amplitude/time and S to AS ratios, was studied using PLX-188 as a stabilizer, and the THC concentration was 2 mg/mL at THC: PLX-188 1:1 w/w (Supplementary Figure S1).
Precipitation was assisted by ultrasonication for PS reduction and nucleation control (Areen Alshweiat, Citation2020).Ultrasonic amplitudes of 40% and 60% were applied for 10 min at a S to AS ratio of 1:20 (Supplementary Table S1). At 40% amplitude, PS was in the micro-range (1155.62 ± 15.28 nm), while at 60% amplitude, PS was significantly decreased (p = .005) to approximately 912.07 ± 73.36 nm. It was evident that the crystal size decreased with the increase in ultrasonic power amplitude. This finding might be attributed to the intermolecular collisions induced by the cavitation generated by ultrasonic waves that led to particle attrition and fracture and subsequently reduced the PS (Prasad & Dalvi, Citation2020). A similar observation was reported by Allam et al. (Citation2017).
Furthermore, sonication time had a crucial effect on PS at 60% amplitude; its influence on THC-NC was depicted in Supplementary Table S1. The prolongation of sonication time from 5 to 30 min (10 min/on and 2 min/off) resulted in a corresponding highly significant decrease in PS (p < .001) from 1661.71 ± 15.72 nm to 626.09 ± 13.51 nm, respectively. This observation could be due to the prolonged time of mixing between the solvent and the antisolvent solution by the probe sonicator, which caused a rapid nucleation rate and a reduction in PS. Therefore, the selected sonication parameters were 60% sonication amplitude for 30 min applied in a pulsatile manner.
Selecting a S to AS ratio was one of the most important aspects of the precipitation process. Upon increasing the S to AS volume ratio from 1:10 to 1:20, the PS of THC-NC was highly significant decreased (p < .001) showing a 50% PS reduction from 1208.91 ± 27.48 nm to 626.09 ± 13.51 nm, respectively. These results could be explained by the low amount of antisolvent, which lead to less space available for the same amount of THC to form NC and a decreased nucleation rate, resulting in large crystals and aggregation (Mehanna et al., Citation2016). However, when the S to AS ratio further increased from 1:20 to 1:40, there was no remarkable additional reduction in the PS, possibly due to equilibration of nucleation and growth kinetics with increasing the antisolvent volume.
After that, the effect of stabilizer type and THC: stabilizer ratio on the PS of THC-NC was studied using an S to AS ratio of 1:20 at a sonication amplitude of 60% for 30 min.
3.1.2. Screening of the stabilizer type and THC: stabilizer ratio
Stabilizers are vital for preventing the aggregation of high-surface energy NC. Stabilizer type and concentration have great potential for developing stable NC (Jacob et al., Citation2020). Different drugs required different stabilizers, and no single stabilizer system was appropriate for all drugs and preparation processes (Allam et al., Citation2017). To produce stable NC, steric and/or electrostatic barriers should be created to avoid instability processes such as particle aggregation, sedimentation, and/or crystal growth due to Ostwald ripening (A. Alshweiat et al., Citation2018; Santos et al., Citation2020).
The selected stabilizer should have a high affinity to the surface of specific drug particles. THC is a non-polar substance; therefore, the ionic stabilizers are not suitable or enough to provide high affinity to surface of THC particles. However, the nonionic stabilizers show high affinity for hydrophobic groups of THC by means of their hydrophobic domains at the surface.
In this study, preliminary investigations were carried out on the most common stabilizers used in the preparation of NC like HPMC E15, MC, PLX-407, PVP K-25, PVA, and PLX-188 (Pınar et al., Citation2023; Shahine et al., Citation2023). Based on visual investigation, PLX-188, PVP-K25, and PVA were found to be the most promising stabilizers for producing homogenous colloidal dispersion. Furthermore, these stabilizers were tested in three different ratios (THC: stabilizer; 1:1, 1:0.5, and 1:0.25 w/w) to study the effect of concentration of stabilizer on PS, PDI and ZP.
As shown in , by increasing the PLX-188 concentration from 1:0.25 to 1:1 (THC: PLX-l88), there was a non-significant change in PS (p = .357) and PDI (p = .094). This might be attributable to the fact that at a 1:0.25 ratio, a sufficient amount of stabilizer was adsorbed onto the particle surface, providing a barrier to agglomeration. However, after a certain concentration, the adsorption reached equilibrium due to the limited surface area, so no change in PS would be observed. This observation was congruent with that reported by Y. Wang et al. (Citation2017), where PLX-188 was used as a stabilizer with curcumin. Also, the ratio 1:0.25 favorably showed a highly significant negative ZP of −39.31 ± 1.07 (p < .001) compared to the ratio 1:1, indicating the physical stability of the prepared NC with a low probability of aggregation and crystal growth.
PVP-K25 was also employed owing to its capability to be adsorbed on the drug particle surface to stabilize them by providing steric repulsions (Zhou et al., Citation2018). When the concentration of PVP-K25 was increased from 1:0.25 to 1:0.5 (THC: PVP-K25), there was a highly significant decrease in PS (from 841.77 ± 16.88 to 537.38 ± 4.92 nm) (p < .001) which could be attributed to the adsorption of an adequate amount of stabilizer, providing a complete coverage of drug particles essential for steric repulsion. This was in agreement with Mohamed et al. (Citation2019), who reported that when the PVP-K25 concentration was increased, the PS of gliquidone NC was decreased. However, increasing the PVP-K25 concentration from 1:0.5 to 1:1 (THC: PVP-K25), resulted in a highly significant increase (p < .001) in PS. This could be attributed to the viscosity-imparting property of PVP-K25. Increasing the aqueous phase viscosity decreased the impact of shear stress applied to the system; this might decrease the extent of size reduction, and this result was in harmony with Allam et al. (Citation2017).
On the other hand, when PVA concentration was increased from 1:0.25 (F7) to 1:1 (F9) (THC: PVA), PS was significantly increased (p < .05) from 1177.82 ± 53.56 nm to 1767.92 ±99.31 nm, respectively. This result was due to the higher PVA concentration, which could increase the aqueous phase viscosity. This could provide a higher resistance to the diffusion of the drug-solvent phase into the external aqueous phase, thus resulting in larger crystals (I. S. Ahmed et al., Citation2020). Additionally, F9 showed the lowest ZP: −20.53 ± 2.89 mV. This change in PS and ZP might be attributed to the deposition of extra PVA onto the surface of particles (Y. Zhao et al., Citation2014; Kumar & Siril, Citation2016).
According to the obtained results, the formulation (F5) with 1:0.5 (THC: PVP-K25) had the smallest particle size even though it precipitated at 4 °C in just 24 h. When the ratio of THC: PVP-K25 in the formulation (F6) was increased to 1:1, PS increased, but stability didn’t improve. This may be because PVP-K25 only formed a thin protective outer layer on the surface of the THC particle, which was insufficient to ensure the stability of the particle (Panich et al., Citation2021). As a result of their effects on THC-NC stability, combinations of polymer and surfactant are taken into consideration to improve the PVP-K25 property (Soroushnia et al., Citation2022). The addition of PLX-188 as a surfactant in low concentration could result in the best stabilization. As a result of its strongly adsorbed anchor blocks, which permit a high degree of adsorption, PLX-188 was joined into a thin protective layer of PVP-K2,5 which allowed a high degree of adsorption (Jain et al., Citation2013). This outcome agreed with Soroushnia et al. (Soroushnia et al., Citation2022).
A cluster and a deep hydrophilic layer were created by the combination of PLX-188 and PVP-K25 in the ratio 1:0.25:0.5 (THC: PLX-188: PVP-K25), which led to the production of a physically stable THC-NC dispersion at 4 °C for 7 days without any changes in its physical appearance or the formation of precipitates. These findings led to the selection of F10 for further investigation and to the study of the impact of various mannitol concentrations (0, 3, and 5% w/v) during the lyophilization process.
3.2. Lyophilization of THC-NC
To overcome particle growth during long-term storage of NC containing high drug concentrations, lyophilization is recommended to increase the shelf-life and facilitate their application (Allam et al., Citation2017). Mannitol was used as a cryoprotectant at a concentration of (0,3, and 5% w/v) to avoid particle interaction and aggregation of NC (Li et al., Citation2018). Supplementary Table S2 shows PS, PDI, and ZP of the selected lyophilized formulation (F10) using different concentrations of mannitol, where cryoprotectant concentration played a role in the lyophilization process efficiency.
As demonstrated in Supplementary Table S2, after reconstitution, there was a non-significant difference (p > 0.05) in PS and PDI of lyophilized F10 before and after lyophilization, which indicated the resistance of F10 to the freeze-drying stress (I. S. Ahmed et al., Citation2020). It also showed a highly significant increase (p < .001) in ZP values, indicating the physical stability of NC with a low probability of aggregation and crystal growth (Ball et al., Citation2017). Furthermore, lyophilization with or without mannitol didn’t affect PS, PDI, and ZP. Therefore, a formulation without mannitol (F10 M0%) was selected for further investigation.
3.3. Characterization of THC-NC
3.3.1. Scanning electron microscopy (SEM)
The surface morphology of coarse powder THC and THC-NC (F10 M0%) is shown in . THC showed irregular crystals at micrometer size with aggregates of non-uniform PS. However, NC were found to be well-defined rod-like structures at the nanoscale.
Figure 3. Characterization of THC-NC: (a) SEM micrographs of THC and THC-NC at different magnification levels (×300 and ×11,000), (b) DSC comparing thermograms of THC-NC to its physical mixture and THC in terms of thermal behavior, (c) PXRD comparing diffractograms of THC-NC to THC in terms of crystallinity, (d) FTIR spectra of THC, PLX-188, PVP K-25 and THC-NC.

3.3.2. Assessment of THC-NC’ solid state properties
DSC thermogram of THC () exhibited the presence of a sharp endothermic peak at 100.17 °C (ΔH = 124.14 J/g), corresponding to THC’s melting temperature. A physical mixture thermogram showed that THC's endothermic peak was still present. However, a DSC thermogram of THC-NC showed that the THC peak had disappeared, indicating that the THC-NC was in an amorphous state (Pukale et al., Citation2021).
The X-ray diffractogram of THC () revealed well-defined, narrow, sharp, and intense peaks at 2Φ = 18.17°, 20.06°, 23.44°, 25.53°, 27.20°, and 28.61° (Trivedi et al., Citation2020). Meanwhile, the THC-NC diffractogram manifested a broad diffraction peak, indicating the amorphous state of the developed THC-NC and hence further confirming the DSC results.
FTIR study was employed for investigating possible interaction between THC and PLX-188, PVP-K25 stabilizers during NC formation. The IR spectrum of THC () displayed a strong broad absorption band for the hydroxyl group (O–H) at 3408 cm−1, C–Hsp2 stretching at 3064 and 3003 cm−1, and C–Hsp3 stretching at 2961, 2933, and 2844 cm−1. An intense band at 1595 cm−1 was assigned to the vibrations of the carbonyl bond (C = O), accompanied by a small shoulder at 1664 cm−1 which was ascribed to the keto-enol tautomerism of THC. A strong, sharp vibrational band at 1509 cm−1 for aromatic ring C = C stretching, 1262 cm−1 for enol C–O stretching, 1031 cm−1 for –C–O–CH3 stretching, medium absorption bands at 1449 cm−1 for C–H bending of the methyl groups, 1404 cm−1 for O–H bending, and 1113 cm−1 for C–OH (Trivedi et al., Citation2020; Truong et al., Citation2022).
PLX-188 absorption bands were exhibited for C–H stretch aliphatic at 2877 cm−1, O–H bending vibration at 1341 cm−1 and C–O (ester) stretching vibration at 1097 cm−1 (Ha et al., Citation2012). Furthermore, the IR spectra of PVP-K25 showed a characteristic absorption band at 1643 cm−1 corresponding to the pyrrolidone C=O group. Other significant bands at 1289 cm−1, and 1271 cm−1 were assigned to the stretching vibrations of C-O and C-N bonds, respectively, while the bands at 1459 cm−1 and 1422 cm−1 referred to the bending modes of the CH2 groups and the absorption peak at 1371 cm−1 due to the C–H bond (Kéri et al., Citation2018). Nevertheless, THC-NC spectrum manifested disappearance of the peak at 1404 cm−1 characteristic to phenolic O–H of THC and a peak at 1097 cm−1 characteristic to C–O in PLX-188 which might be ascribed to possible hydrogen bonding formation between THC and PLX-188.
3.3.3. Stability study of lyophilized THC-NC
The stability of lyophilized THC-NC (F10 M0%) was assessed at 4 °C for 10 months in terms of PS, PDI, and ZP after reconstitution. As shown in , there was non-significant difference (p > 0.05) in PS and PDI up to 10 months of storage at 4 °C with minimal non-significant decrease in ZP during the storage, suggesting good storage stability and maintaining their physicochemical properties over storage. This stability can be attributed to the steric stabilization provided by complete surface coverage of THC particles by PLX-188 and PVP-K25. Additionally, lyophilization is a widely used method to enhance the stability and extend the shelf life of various preparations by removing the total water content from the drug formulation (Moustafa et al., Citation2023).
Table 3. Stability data at 4 °C of THC-NC (F10 M0%).
3.4. Preparation and characterization of THC-S and THC-NC-S
3.4.1. Screening of different polymers for sponge formulation
Composite sponges were prepared by a simple casting/freeze-drying technique using κc as a primary polymer combined with XG, HPMC K15M, or HPMC K4M as a secondary polymer. Plain sponges were evaluated regarding their physical integrity and mechanical properties of the final lyophilized products. Among different plain sponges, S2 (κc/HPMC K4M), S6 (κc/XG) and S9 (κc/HPMC K15M) (0.8/0.2% w/v; 3.8 ± 0.2 mm thickness/12.7 ± 0.3 mm diameter) showed acceptable physical properties such as good appearance, a smooth, flexible, non-sticky surface, and were easily separated from the mold. Such characteristics make these formulae suitable for easy application.
When the ex vivo mucoadhesion test was used to distinguish between the selected three sponges, the S2 sponge (κc/HPMC K4M) showed a low mucoadhesion force value of 0.04 ± 0.01 N. This could be explained by the lower hydration and swelling properties of HPMC K4M owing to its low viscosity grade (Mohammed et al., Citation2022). Therefore, S6 (κc/XG) and S9 (κc/HPMC K15M) were found to be good candidates for subsequent drug loading in S10, and S12 sponge formulations.
3.4.2. SEM
SEM micrographs of selected of plain sponges (S6, S9), THC-NC-S (S10, S12), and THC-S (S11, S13) were taken under different magnification powers as presented in . All the developed sponges had a good porous structure with varying degrees. For plain sponges, S9 (κc/HPMC K15M) possessed a more homogenous and well-defined porous structure, while S6 (κc/XG) showed a denser structure (). This might be attributed to the high intrinsic viscosity of XG even at low concentrations owing to its high molecular weight and hydrogen bonding interactions (Patel et al., Citation2020). For HPMC K15M containing sponge, HPMC K15M macromolecules might separate water molecules and prevent water molecules from accumulation during freezing, resulting in higher porosity (Wu et al., Citation2010).
Figure 4. SEM micrographs of plain sponges: (a) S6 and (b) S9, THC-NC-S: (c) S10 and (d) S12, THC-S: (e) S11 and (f) S13, (1): Surface view at low magnification (×50), (2): surface view at higher magnification (×11,000), (3): cross sectional view at intermediate magnification (×85).

Upon loading of THC-NC, sponge morphology was affected, as shown in , c1 and d1. THC-NC-S exhibited a more dense structure compared to their plain sponges. It was clear that THC-NC embedded in the polymer matrix affected the porous structure, as previously mentioned with chitosan sponge loaded with nanoemulsion (Rosso et al., Citation2021). Additionally, at higher magnification power (×11,000), micrographs () showed that THC-NC were immersed into the polymer matrix in a rod-like shape. This could be due to the compatibility of THC-NC with the polymers used.
3.4.3. Folding endurance
Folding endurance of buccal sponges is closely related to their flexibility, which is an essential physical characteristic required for ease of application on the buccal mucosa. The selected plain sponges (S6, S9) and THC-NC-S (S10, S12) showed good flexibility without any cracks or pin holes formed during folding at many times above 300-fold, which indicated their good mechanical properties and high flexibility, which is highly desired as it will prevent them from breaking during packaging or administration (Alhallak et al., Citation2023).
3.4.4. Porosity
A sponge porous structural pattern developed during freeze-drying as a result of ice sublimation. The porosity of the selected sponges is shown in . The porosity values were harmonious with the results obtained from SEM and emphasized them.
3.4.5. Swelling behavior
Adequate hydration of a mucoadhesive system is a critical property for the uniform and controlled release of therapeutic and effective mucoadhesion (Hazzah et al., Citation2015). Swelling values of S6, S9, S10, and S12 were shown in ; different swelling behaviors were seen, although the same concentration of κc was incorporated in all selected sponges. This could be attributed to the presence of different polymers in the sponge polymer blend such as HPMC K15M or XG, which influenced hydration and hydrogen bonding to different degrees. According to Meng et al. (Citation2021), several parameters could affect the swelling rate, such as hydrophilicity, the structure of polymer networks, and the interactions among polymer chains.
For plain sponges S6 (κc/XG) and S9 (κc/HPMC K15M), there was no statistically significant difference (p = .091) in the swelling, but S6 exhibited superior swelling capability, which might be imputed to the XG structure (polyanion) owing to carboxyl group ionization. This ionization improved hydrogen bonding with water and enhanced hydration and swelling capacity (M. A. Ahmed et al., Citation2021). A similar observation was reported by Pérez Zamora et al. (Citation2022), who reported that the charges along a polymer facilitated the entry of water in sufficient quantity to cause hydration and swelling. THC-NC-S showed less swelling % than the plain ones keeping the same order where of S10 sponge was significantly higher than (p < .05) S12 sponge in swelling activity.
3.4.6. Ex vivo mucoadhesion assessment
The mucoadhesive force is a relevant parameter frequently employed to describe the maximum force needed to detach mucoadhesive delivery systems from buccal mucosa. The higher the mucoadhesive force, the longer the residence time. Mucoadhesive forces of the plain sponges (S6, S9) and THC-NC-S (S10, S12) were compared. S6 (κc/XG) showed a significant higher mucoadhesive force than S9 (κc/HPMC K15M) (p < .05), where mucoadhesive forces were recorded to be 0.24 ± 0.02 N and 0.14 ± 0.01 N for S6 and S9, respectively. Furthermore, upon loading of THC-NC, THC-NC-S mucoadhesion values showed nonsignificant change compared to the plain ones.
The mucoadhesive properties of the developed sponges were attributed to their κc content due to its ability to interact with mucus layer by hydrogen bond formation involving sulfate and hydroxyl groups (Yermak et al., Citation2020). Furthermore, the formation of coordinate bond between the sulfate group in κc and the NH2 groups in mucin was also expected to lead to stronger mucoadhesion forces (Kianfar et al., Citation2014). Additionally, there were cavities in the macromolecular structure of κc probably contributing to its greater swelling on the mucin surface and its increased binding (Sokolova et al., Citation2013).
For XG containing sponges, the greater mucoadhesion was related to XG’s nature as an anionic polyelectrolyte containing glucuronic acid and pyruvate in its side chains (Shiledar et al., Citation2014). Furthermore, XG was involved in hydrogen bonds through its free COO- groups and/or its large number of OH- groups with mucin glycoproteins. Moreover, XG was reported to exhibit better mucoadhesion performance than other common mucoadhesive polymers such as tragacanth gum, chitosan, and HPMC (Cazorla-Luna et al., Citation2021). Regarding HPMC K15M containing sponges, they exhibited lower mucoadhesivity compared to XG sponges; this might be imputed to their low hydrogen-bonding capability and inability to interact electrostatically with mucin due to their nonionic nature (Bertram & Bodmeier, Citation2006).
3.4.7. Assessment of THC-NC-S’ Solid state properties
FTIR was used to study the change in the functional groups. The IR spectra of κc () showed characteristic absorption bands at 1235 cm−1 and 841 cm−1 which were attributed to S = O stretching vibrations of the sulfated groups and D-galactose-4-sulfate, respectively. Additionally, the band at 923 cm−1 demonstrated the existence of C-O-C in the 3-anhydro-D-galactose for κc (Balasubramanian et al., Citation2018; Farias & Boateng, Citation2020). IR spectra of XG showed an absorption band at 3380 cm−1 that was corresponding to OH stretching. The peak appearing at 2885 cm−1 could be ascribed to C–H. The band at 1716 cm−1 was due to the C = O stretching vibrations. Additionally, the peaks at 1619 cm−1 and 1426 cm−1 were due to the asymmetrical and symmetrical vibrations of COO– groups (Zheng et al., Citation2019; Said et al., Citation2021). The spectra of SL showed bands at 3317 cm−1 which was attributed to O-H stretching mode, 2937 cm−1 that was due to C-H stretching mode, and it was evident that polyols had peaks in the range of 1500–1200 cm−1 (Pourfarzad et al., Citation2018). Furthermore, the IR spectrum of THC-NC-S (S10) (κc/XG) was found to be a superposition of the parent components, which indicated that there was no apparent interaction.
Figure 6. (a) FTIR spectra of κc, XG, SL and THC-NC-S (S10), (b) DSC comparing thermograms of THC-S (S11), THC-NC-S (S10) and their physical mixtures to THC and THC-NC in terms of thermal behavior, (c) Release profile of THCmfromnTHC-NC-SmS10 (κc/XG),kS12 (κc/HPMC K15M),kTHC-Sk(S11(κc/XG)), S13 (κc/HPMC K15M), and THC-NC in PB (pH 6.8), containing 0.25% of SLS using membraneless method, (d) Cell viability of SCC-4 after exposure to THC-NC and free THC for 24 h.

In , DSC thermogram of THC-NC-S (S10) (κc/XG) showed the disappearance of the endothermal peak of THC, indicating that THC-NC maintained its amorphous nature in the sponge. On the contrary, THC-S (S11), where the sharp endothermic peak at 100.17 °C was still detected. Furthermore, the PXRD diffractogram of THC-NC-S (S10) confirmed the presence of THC-NC in its amorphous state, as shown in Supplementary Figure S2.
3.4.8. Drug content
The percentage drug content in the selected THC-NC-S (S10) (κc/XG) and (S12) (κc/HPMC K15M) was found to be 98.47 ± 0.34%, and 96.73 ± 0.14% respectively. While the % drug content in THC-S (S11) (κc/XG) and (S13) (κc/HPMC K15M) was found to be 98.55 ± 0.25%, and 97.62 ± 0.38% respectively. The THC content was observed to be within an acceptable range for all the selected sponges, which indicated uniform drug distribution.
3.4.9. In vitro release study
The solubility of THC in different media at 37 °C is represented in . THC showed poor solubility in water and PB (pH = 6.8). As shown in , the solubility of THC was remarkably improved by the addition of small concentrations of SLS (0.25%), but when it was added in high concentrations of 0.5%, it showed a remarkable enhancement in the solubility of THC. Consequently, the lower concentration of SLS (0.25%) was chosen to minimize any undesirable effects of SLS (Atia et al., Citation2019). So, PB (pH = 6.8) containing 0.25% SLS was selected as an in vitro release medium to achieve sink conditions as previously reported in many publications (Shehata et al., Citation2022; Mahajan et al., Citation2023).
Table 4. Solubility of THC in different media at 37 °C.
In , the THC release rate from THC-NC-S (S10 and S12) was compared with THC-S (S11 and S13) and free THC-NC, as well. The release profile of THC-NC clearly showed a fast release of 95% within 15 min, which was contributed to the smaller size of NC that provided an overall increased surface area (Kalam et al., Citation2022). While THC-NC-S showed biphasic pattern of drug release with an initial rapid release up to 8 h and followed by a sustained slower phase where 90% of THC was released over 4 days. Initial rapid release could be attributed to the escape of drug molecules present closer to the surface once they come in contact with the release medium immediately. The sustained release behavior of sponges occurred due to polymer hydration and the formation of swollen gel structures, which consequently increases the diffusion path length for THC-NC, hence retarding its release (Freag et al., Citation2018). Furthermore, the thick gel layer formed on the swollen sponge surface was capable of preventing matrix disintegration and controlling additional water penetration, limiting THC release (Atia et al., Citation2019). Nevertheless, incorporation of THC powder in THC-S showed dramatic slowing in drug release where only 15% of THC released within 8 h and reached 30% over 4 days. These observations confirmed that THC-NC-S showed a significant (p ≤ 0.05) improvement in THC release rate compared to THC-S. This might be attributed to the low solubility of THC compared to the developed THC-NC (C. Wang, Wang et al., Citation2022).
3.5. Cellular cytotoxicity assay
The cellular cytotoxicity of THC-NC against SCC-4 cells for 24 h was studied compared to Free THC using the MTT assay, and the results are shown in . After that, IC50 was determined to compare the activity of different formulations. It was found that the IC50 values were 149 µg/mL and 80 µg/mL for free THC and THC-NC, respectively. This verified that THC-NC showed highly significant toxicity (p < .001) to SCC-4 cells with a 1.9-fold decrease in IC50 compared to free THC. This could be due to the higher cellular uptake of THC-NC via endocytosis, as the nano-sized particles could easily escape phagocytosis and penetrate more efficiently into the cancer cells (Farooq et al., Citation2020). Moreover, NC improved THC’s water solubility. which led to an abundant amount of cellular THC being available to treat cancer cells.
3.6. In vivo study
The Syrian male hamsters were used for induction of oral precancerous condition, as anatomically hamsters have sac-like structures known as buccal pouch. The cheek pouches have been called immunologically privileged sites, where precancerous and tumors can be induced without being rejected by the hamster immune system (Vairaktaris et al., Citation2008). Additionally, molecular and cellular changes in the multistep process of oral carcinogenesis in hamsters are thought to represent similar changes to human oral cancer (Yapijakis et al., Citation2019).
3.6.1. Clinical assessment
Clinically, the standard pink color of the HBPs turned mainly into non-scrapable leukoplakic lesions after 14 weeks of DMBA painting, alternating with a few erythroplakia. Sequential histological examination of the oral lesions displayed the premalignant hallmarks of epithelial dysplasia (Supplementary Figure S4(a,b)).
After 10 days of treatment, the white OPL treated with THC-NC-S remained static compared with the untreated and plain sponge-treated groups, where the white lesions evolved into mixed erythroleukoplakia with the concomitant appearance of small intraoral polyps. In the former THC-NC-S group, the lesions neither changed clinically nor metrically. Meanwhile, the leukoplakia treated with THC-S continued to increase in size.
After 25 days of THC-NC-S topical treatment, the proliferative white lesions receded to simple leukoplakia. The architecture and resiliency of the mucosa returned to almost normal. The clinical picture differed in the THC-S treated lesions, where some leukoplakic lesions turned into papillary red patches. Meanwhile, red masses appeared after 25 days of treatment with plain sponges similar to the oral polyps in the untreated group ( and Supplementary Figure S4(c)).
Figure 7. The clinical outcome of the treatment modalities with the concomitant immunohistochemical analyses. (a) Clinically, the OPL in HBPs between THC-S and THC-NC-S groups are almost similar in size and shape after ten days of treatment (white dotted circles). Meanwhile, the plain sponge group reveals mixed erythroleukoplakic lesions (orange dotted circles), whereas small intraoral polyps appear in the untreated (control) group (blue dotted circle). with time, the potency of the NC treatment accentuates after 25 days, where the OPL constrains (white circle). Meanwhile, some white lesions in the THC-S group evolve into erythroplakia (red dotted circle). on the other hand, a plain sponge had no therapeutic potentiality, manifested by the development of OC as those developed in the control group (black dotted circles). (b) immunoexpression of cyclin D1 shows a decrease in the immunostaining in both THC-S and THC-NC-S groups after 25 days of application (sections are ×400 magnification). However, invasive carcinomas with intense cyclin D1 expression are seen in control, and hamsters receiving plain sponges especially showed this at higher magnification inserts (sections are ×200 magnification, inserts are ×400 magnification). (c) a representative graph illustrating the mean area percent difference during 10- and 25-days’ time intervals for the four groups with statistically significant decrease in cyclin D1 expression noted at 25 the days’ time-point (*p < .001) denote by asterisks.

Therefore, these findings showed that the THC-NC-S improved the white lesions more effectively in comparison to the THC-S and plain sponge. This superior therapeutic efficacy of THC-NC-S could be due to THC-NC improved THC adhesion and accumulation into the affected tissues(X. Zhang et al., Citation2020), while THC-NC-S had potent mucoadhesive activity and high porosity, allowing for the persistence of THC in the buccal cavity for a prolonged period of time.
3.6.2. Histopathological analysis
Histologically, using H&E, after 10 days of treatment, moderate epithelial dysplasia with dysplastic criteria involving more than half of the epithelium thickness was seen in both THC-S and THC-NC-S. However, in untreated and plain sponge groups, there is severe epithelial dysplasia with loss of cohesiveness in more than 2/3 of the epithelial thickness, with some areas showing carcinoma in situ. Furthermore, 25 days after application, tissue sections obtained from the treated groups revealed regression in the degree of dysplasia. Meanwhile, the untreated and plain sponge showed evident point of invasion with early invasive OSCC with evident points of invasion of the malignant epithelial cells into the underlying lamina propria (Supplementary Figure S4(c)). Plain sponge didn’t show any improvement in lesions, compared to THC-NC-S or THC-S. This confirmed that THC-NC-S halted the development of malignant lesions which might be attributed to increasing THC availability inside the OPL with the aid of the mucoadhesive properties of the sponge, preventing its detachment from the lesion and increasing its retention time.
3.6.3. Immunohistochemical and morphometric analysis
The cell cycle progression was assessed by immunohistochemical analysis of cyclin D1. At 10 days, there was a decrease in cyclin D1 mean area percent in groups receiving THC-S and THC-NC-S, which was not statistically significant (control: 40.4 ± 12.5, plain: 39.7 ± 10, THC-S: 31.7 ± 8, THC-NC-S: 29.4 ± 5) (p = .64). However, 25 days after daily application of the treatment, a highly statistically significant decrease in the immunoexpression of cyclin D1 was observed in both groups with THC-S and THC-NC-S (control: 50.4 ± 11.5, plain: 45.4 ± 10.5, THC-S: 30 ± 4.5, THC-NC-S: 12.07 ± 5.2) (p < .0001). In line with our clinical observations, the THC-NC-S formulation showed a statistically significant decrease when compared with THC-S (THC-S: 30 ± 4.5, THC-NC-S: 12.07 ± 5.2) (p < .009) (). Therefore, THC-NC-S were found to be superior in decrease immunoexpression of cyclin D1 compared to THC-S. This result helped to explain the clinical efficacy of THC-NC.
The obtained results exhibited that effective curative outcomes of OPL could be achieved using a low dose of THC over only 25 days by employing a daily buccal mucoadhesive sponge loaded with THC-NC. The novel system developed resulted in a significant amelioration of OPL in all treated animals, inhibiting the risk of malignant transformation. However, remarkable decrease in size and thickness of lesion and pain was reported after topical application of THC gel applied 5 times daily over 12 weeks on human volunteers. Additionally, remarkable improvement was seen histopathologically in only 3 out of 8 patients (Chhaparwal et al., Citation2018). These findings verified that the novel THC-NC integrated with a mucoadhesive sponge enabled THC to be retained at high concentration inside the OPL, hindering its rapid clearance and thus maximizing its chemopreventive activity. Collectively, the developed THC-NC improved the therapeutic effect of THC on the SCC-4 cell line. Moreover, loading THC-NC into mucoadhesive κc/XG composite sponges effectively prevented the development of cancerous lesions.
3.7. Conclusion
In the present study, mucoadhesive buccal nanocomposite sponge loaded with THC-NC were successfully developed for localizing the anticancer THC into the oral cancerous and precancerous lesions. THC-NC were prepared and evaluated to enhance THC physicochemical properties along with its adhesion and accumulation into the affected lesions. Efficient loading of THC-NC into composite κc: XG sponges was investigated, capitalizing on their mucoadhesion properties to buccal mucosa. Successful loading of THC-NC into sponges was confirmed by SEM images. Moreover, the developed sponges could sustain THC release. Interestingly, THC-NC exhibited great anticancer potential in the SCC-4 cell line. Besides, THC-NC-S showed significant healing of oral precancerous lesions on a DMBA-hamster model compared to THC-S which was evident from clinical histological assessment. In addition, THC-NC-S effectively suppressed cyclin D1, which is implicated in oral cancer pathophysiology. Therefore, THC-NC-S represented a promising local drug delivery system in the treatment of early stages of cancer of the oral cavity preventing malignant transformation. Further clinical studies are needed to evaluate the effectiveness of THC-NC-S in the treatment of oral cancer.
Ethical approval
The Syrian male hamsters were used for induction of oral precancerous condition, as anatomically hamsters have sac-like structures known as buccal pouch. The cheek pouches have been called immunologically privileged sites, where precancerous and tumors can be induced without being rejected by the hamster immune system. Additionally, molecular and cellular changes in the multistep process of oral carcinogenesis in hamsters are thought to represent similar changes to human oral cancer.
All institutional and national guidelines for the care and use of laboratory animals were followed. All procedures were conducted according to the established principles of animal experiments in the UK Directive of 1986; 86/609/EEC. The experimental protocols were approved by the Alexandria University Ethics Committee (IRBNO:00010556-IORG0008839).
Hamsters at 5 weeks of age (80–120 g) were obtained from VACSERA, Cairo, Egypt. They were housed in show box cages (Technoplast, Italy), one per cage, at the Animal House Unit in the Medical Research Institute, Alexandria University. They were allowed to acclimatize for 2 weeks before starting the experiment. The hamsters were housed at a room temperature of 23 ± 1 °C, a relative humidity of 50% ± 5, and a 12-h light/dark cycle. Hamsters received standard diet and water throughout the experimental period, with close monitoring of their normal behavior throughout the duration of the experiment. During therapeutic application, animals were lightly sedated by IP injection of a low-dosed combination of 0.1 mL/100 g ketamine hydrochloride and 0.05 mL/100 g xylazine hydrochloride. At the end of experimental duration, hamsters were euthanized by an overdose of ketamine hydrochloride (>30 mg/kg), given IP. Cautious assessment of animal was then performed to confirm death and the remains of animals’ bodies were handled by special authorities. All animal experiments were performed following the ARRIVE guidelines.
Authors’ contributions
All authors contributed to the design of the article. The initial idea of the article was developed by Ossama Y. Abdallah who conceived and designed the analysis, contributed to study design, interpretation of data, supervision, and manuscript review. Shimaa A. Elbanna performed the literature search, data collection/analysis, wrote the original draft and contributed to publication process. Hebatallah S. Barakat contributed to study design, interpretation of data, supervision, and manuscript review. Heba MK. Ebada contributed to study design, interpretation of data, supervision, and manuscript review. Marwa M. Essawy and Hend M. Abdelhamid carried out the in vivo experiment involving study design, clinical assessment, histopathological analysis, immunohistochemical/morphometric analysis, and manuscript review. All authors read and approved the final version of the manuscript.
Supplemental Material
Download MS Word (567.2 KB)Acknowledgements
The authors express deep gratitude to the Center of Excellence for Research in Regenerative Medicine and Applications (CERRMA), Faculty of Medicine, Alexandria University (STDF-Funded) for providing technical assistance and facilitation of the microscopic imaging unit.
Disclosure statement
No potential conflict of interest was reported by the author(s).
Data availability statement
The datasets used and/or analyzed during the current study are available from the corresponding author on reasonable request.
Additional information
Funding
References
- Abdel Hamid HM, Darwish ZE, Elsheikh SM, et al. (2021). Following cytotoxic nanoconjugates from injection to halting the cell cycle machinery and its therapeutic implications in oral cancer. BMC Cancer 21:1. doi: 10.1186/s12885-021-07849-x.
- Ahmed IS, Elnahas OS, Assar NH, et al. (2020). Nanocrystals of fusidic acid for dual enhancement of dermal delivery and antibacterial activity: in vitro, ex vivo and in vivo evaluation. Pharmaceutics 12:199. doi: 10.3390/pharmaceutics12030199.
- Ahmed MA, Abdelgawad WY, Gad MK, Mohamed MI. (2021). A novel approach for the treatment of oral ulcerative lesion using mucoadhesive proniosome gel. J Drug Delivery Sci Technol 63:102460. doi: 10.1016/j.jddst.2021.102460.
- Alhallak M, Karpukhina N, Patel M. (2023). Triamcinolone acetonide release modelling from novel bilayer mucoadhesive films: an in vitro study. Dent Mater 39:595–19. doi: 10.1016/j.dental.2023.04.005.
- Allam AN, Hamdallah SI, Abdallah OY. (2017). Chitosan-coated diacerein nanosuspensions as a platform for enhancing bioavailability and lowering side effects: preparation, characterization, and ex vivo/in vivo evaluation. Int J Nanomedicine 12:4733–45. doi: 10.2147/IJN.S139706.
- Alqahtani WS, Almufareh NA, Al-Johani HA, et al. (2020). Oral and oropharyngeal cancers and possible risk factors across gulf cooperation council countries: a systematic review. World J Oncol 11:173–81. doi: 10.14740/wjon1283.
- Alqalshy EM, Ibrahim AM, Abdel-Hafiz AA-S, et al. (2022). Effect of docosahexaenoic acid as a chemopreventive agent on experimentally induced hamster buccal pouch carcinogenesis. Cancer Treat Res Commun 31:100558. doi: 10.1016/j.ctarc.2022.100558.
- Alshweiat A. (2020). Development and characterization of loratadine nanosystems for intranasal delivery using quality by design approach. PhD thesis, Institute of Pharmaceutical Technology and Regulatory Affairs, Faculty of pharmacy, University of Szeged. doi:10.14232/phd.10508.
- Alshweiat A, Katona G, Csoka I, Ambrus R. (2018). Design and characterization of loratadine nanosuspension prepared by ultrasonic-assisted precipitation. Eur J Pharm Sci 122:94–104. doi: 10.1016/j.ejps.2018.06.010.
- Andrade AO, Parente ME, Ares G. (2014). Screening of mucoadhesive vaginal gel formulations. Braz J Pharm Sci 50:931–41. doi: 10.1590/S1984-82502014000400029.
- Atia NM, Hazzah HA, Gaafar PME, Abdallah OY. (2019). Diosmin nanocrystal-loaded wafers for treatment of diabetic ulcer: in vitro and in vivo evaluation. J Pharm Sci 108:1857–71. doi: 10.1016/j.xphs.2018.12.019.
- Balasubramanian R, Kim SS, Lee J. (2018). Novel synergistic transparent k-carrageenan/xanthan gum/gellan gum hydrogel film: mechanical, thermal and water barrier properties. Int J Biol Macromol 118:561–8. doi: 10.1016/j.ijbiomac.2018.06.110.
- Ball R, Bajaj P, Whitehead K. (2017). Achieving long-term stability of lipid nanoparticles: examining the effect of pH, temperature, and lyophilization. Int J Nanomedicine 12:305–15. Volume doi: 10.2147/ijn.s123062.
- Bertram U, Bodmeier R. (2006). In situ gelling, bioadhesive nasal inserts for extended drug delivery: In vitro characterization of a new nasal dosage form. Eur J Pharm Sci 27:62–71. doi: 10.1016/j.ejps.2005.08.005.
- Cazorla-Luna R, Martín-Illana A, Notario-Pérez F, et al. (2021). Naturally occurring polyelectrolytes and their use for the development of complex-based mucoadhesive drug delivery systems: an overview. Polymers 13:2241. doi: 10.3390/polym13142241.
- Chavan DU, Marques SM, Bhide PJ, et al. (2020). Rapidly dissolving felodipine nanoparticle strips-formulation using design of experiment and characterisation. J Drug Delivery Sci Technol 60:102053. doi: 10.1016/j.jddst.2020.102053.
- Chen Q, Dan H, Pan W, et al. (2021). Management of oral leukoplakia: a position paper of the Society of Oral Medicine, Chinese Stomatological Association. Oral Surg Oral Med Oral Pathol Oral Radiol 132:32–43. doi: 10.1016/j.oooo.2021.03.009.
- Chhaparwal Y, M Pai K, Kamath M. s, et al. (2018). Efficacy and safety of tetrahydrocurcuminoid in the treatment of oral leukoplakia: a pilot study. Asian J Pharm Clin Res 11:194. doi: 10.22159/ajpcr.2018.v11i12.28107.
- Council NR. (2010). Guide for the care and use of laboratory animals. https://grants.nih.gov/grants/olaw/guide-for-the-care-and-use-of-laboratory-animals.pdf
- Elnaggar YS, Shehata EM, Galal S, Abdallah OY. (2017). Self-emulsifying preconcentrates of daidzein-phospholipid complex: design, in vitro and in vivo appraisal. Nanomedicine 12:893–910. doi: 10.2217/nnm-2016-0387.
- Essawy MM, El-Sheikh SM, Raslan HS, et al. (2021). Function of gold nanoparticles in oral cancer beyond drug delivery: implications in cell apoptosis. Oral Dis 27:251–65. doi: 10.1111/odi.13551.
- Farias S, Boateng JS. (2020). In vitro, ex vivo and in vivo evaluation of taste masked low dose acetylsalicylic acid loaded composite wafers as platforms for buccal administration in geriatric patients with dysphagia. Int J Pharm 589:119807. doi: 10.1016/j.ijpharm.2020.119807.
- Farooq MA, Xu L, Aquib M, et al. (2020). Denatured food protein-coated nanosuspension: a promising approach for anticancer delivery of hydrophobic drug. J Mol Liq 303:112690. doi: 10.1016/j.molliq.2020.112690.
- Freag MS, Saleh WM, Abdallah OY. (2018). Laminated chitosan-based composite sponges for transmucosal delivery of novel protamine-decorated tripterine phytosomes: ex-vivo mucopenetration and in-vivo pharmacokinetic assessments. Carbohydr Polym 188:108–20. doi: 10.1016/j.carbpol.2018.01.095.
- Gangwar SK, Kumar A, Jose S, et al. (2022). Nuclear receptors in oral cancer-emerging players in tumorigenesis. Cancer Lett 536:215666. doi: 10.1016/j.canlet.2022.215666.
- Gao F, Chen M, Yu J, et al. (2022). Tetrahydrocurcumin protects against nonalcoholic fatty liver disease by improving lipid metabolism and redox homeostasis. J Funct Foods 89:104957. doi: 10.1016/j.jff.2022.104957.
- Ha J-M, Kang S-Y, Park C-W, et al. (2012). Effect of poloxamer on physicochemical properties of tacrolimus solid dispersion improving water solubility and dissolution rate. Journal of Pharmaceutical Investigation 42:171–6. doi: 10.1007/s40005-012-0025-4.
- Habibi N, Bissonnette C, Pei P, et al. (2023). Mucopenetrating janus nanoparticles for field-coverage oral cancer chemoprevention. Pharm Res 40:749–64. doi: 10.1007/s11095-022-03465-x.
- Hazzah HA, Farid RM, Nasra MMA, et al. (2015). Lyophilized sponges loaded with curcumin solid lipid nanoparticles for buccal delivery: development and characterization. Int J Pharm 492:248–57. doi: 10.1016/j.ijpharm.2015.06.022.
- Jacob S, Nair AB, Shah J. (2020). Emerging role of nanosuspensions in drug delivery systems. Biomater Res 24:3. doi: 10.1186/s40824-020-0184-8.
- Jain D, Athawale R, Bajaj A, et al. (2013). Studies on stabilization mechanism and stealth effect of poloxamer 188 onto PLGA nanoparticles. Colloids Surf B Biointerfaces 109:59–67. doi: 10.1016/j.colsurfb.2013.03.027.
- Jongjitphisut N, Thitikornpong W, Wichitnithad W, et al. (2023). A stability-indicating assay for tetrahydrocurcumin-diglutaric acid and its applications to evaluate bioaccessibility in an in vitro digestive model. Molecules 28:1678. doi: 10.3390/molecules28041678.
- Kalam MA, Iqbal M, Alshememry A, et al. (2022). Fabrication and characterization of tedizolid phosphate nanocrystals for topical ocular application: improved solubilization and in vitro drug release. Pharmaceutics 14:1328. doi: 10.3390/pharmaceutics14071328.
- Kassem MAA, ElMeshad AN, Fares AR. (2015). Lyophilized sustained release mucoadhesive chitosan sponges for buccal buspirone hydrochloride delivery: formulation and in vitro evaluation. AAPS PharmSciTech 16:537–47. doi: 10.1208/s12249-014-0243-3.
- Kéri O, Kocsis E, Nagy ZK, et al. (2018). Preparation of AL2O3 coated PVA and PVP nanofibers and AL2O3 nanotubes by electrospinning and atomic layer deposition. Rev Roum Chim 63:401–6.
- Keshk AA, Elsayed NH, Zareh MM, et al. (2023). Kappa-carrageenan for benign preparation of CdSeNPs enhancing the electrochemical measurement of AC symmetric supercapacitor device based on neutral aqueous electrolyte. Int J Biol Macromol 234:123620. doi: 10.1016/j.ijbiomac.2023.123620.
- Kianfar F, Ayensu I, Boateng JS. (2014). Development and physico-mechanical characterization of carrageenan and poloxamer-based lyophilized matrix as a potential buccal drug delivery system. Drug Dev Ind Pharm 40:361–9. doi: 10.3109/03639045.2012.762655.
- Kumar R, Siril PF. (2016). Preparation and characterization of polyvinyl alcohol stabilized griseofulvin nanoparticles. Mater Today: Proc 3:2261–7. doi: 10.1016/j.matpr.2016.04.135.
- Lai C-S, Ho C-T, Pan M-H. (2020). The cancer chemopreventive and therapeutic potential of tetrahydrocurcumin. Biomolecules 10:831. doi: 10.3390/biom10060831.
- Lai C-S, Wu J-C, Yu S-F, et al. (2011). Tetrahydrocurcumin is more effective than curcumin in preventing azoxymethane-induced colon carcinogenesis. Mol Nutr Food Res 55:1819–28. doi: 10.1002/mnfr.201100290.
- Li Q, Chen F, Liu Y, et al. (2018). A novel albumin wrapped nanosuspension of meloxicam to improve inflammation-targeting effects. Int J Nanomedicine 13:4711–25. doi: 10.2147/ijn.s160714.
- Lin H-W, Chen T-C, Yeh J-H, et al. (2022). Suppressive effect of tetrahydrocurcumin on pseudomonas aeruginosa lipopolysaccharide-induced inflammation by suppressing JAK/STAT and Nrf2/HO-1 pathways in microglial cells. Oxid Med Cell Longev 2022:4978556. doi: 10.1155/2022/4978556.
- Liu M, Wen C, Pan S. (2021). Modulator effect of mangiferin on biochemical characterization in 7,12-dimethylbenz[a]anthracene induced oral cancer in experimental hamsters. Vet Med Sci 7:2015–25. doi: 10.1002/vms3.500.
- Liu W, Zhang Z, Lin G, et al. (2017). Tetrahydrocurcumin is more effective than curcumin in inducing the apoptosis of H22 cells via regulation of a mitochondrial apoptosis pathway in ascites tumor-bearing mice. Food Funct 8:3120–9. doi: 10.1039/c7fo00484b.
- Lopez-Vidal L, Real JP, Real DA, et al. (2022). Nanocrystal-based 3D-printed tablets: semi-solid extrusion using melting solidification printing process (MESO-PP) for oral administration of poorly soluble drugs. Int J Pharm 611:121311. doi: 10.1016/j.ijpharm.2021.121311.
- Loron A, Gardrat C, Tabary N, et al. (2021). Tetrahydrocurcumin encapsulation in cyclodextrins for water solubility improvement: synthesis, characterization and antifungal activity as a new biofungicide. Carbohydrate Polymer Technologies and Applications 2:100113. doi: 10.1016/j.carpta.2021.100113.
- Mahajan HD, Desmukh SB, Sisode NR, et al. (2023). Development and evaluation of oral nanosuspension of rosuvastatin. J Biomed Eng 40:24–34.
- Mehanna M, Elgindy N, Mohyeldin S. (2016). The relevancy of controlled nanocrystallization on rifampicin characteristics and cytotoxicity. Int J Nanomedicine 11:2209–22. doi: 10.2147/ijn.s94089.
- Meng X, Lu Y, Gao Y, et al. (2021). Chitosan/alginate/hyaluronic acid polyelectrolyte composite sponges crosslinked with genipin for wound dressing application. Int J Biol Macromol 182:512–23. doi: 10.1016/j.ijbiomac.2021.04.044.
- Miranda CA, Beretta EM, Ferreira LA, et al. (2023). Role of biotransformation in the diazinon-induced toxicity in HepG2 cells and antioxidant protection by tetrahydrocurcumin. Toxicol Rep 10:32–9. doi: 10.1016/j.toxrep.2022.12.005.
- Mohamed MS, Abdelhafez WA, Zayed G, Samy AM. (2019). Optimization, in-vitro release and in-vivo evaluation of gliquidone nanoparticles. AAPS PharmSciTech 21:35. doi: 10.1208/s12249-019-1577-7.
- Mohammed MF, Sadeq ZA, Salih OS. (2022). Formulation and evaluation of mucoadhesive buccal tablet of Anastrozole. J Adv Pharm Educ Res 12:38–44. doi: 10.51847/lEmpSyVsbx.
- Momin M, Kurhade S, Khanekar P, Mhatre S. (2016). Novel biodegradable hydrogel sponge containing curcumin and honey for wound healing. J Wound Care 25:364–72. doi: 10.12968/jowc.2016.25.6.364.
- Moustafa MA, El-Refaie WM, Elnaggar YSR, et al. (2023). Fucoidan/hyaluronic acid cross-linked zein nanoparticles loaded with fisetin as a novel targeted nanotherapy for oral cancer. Int J Biol Macromol 241:124528. doi: 10.1016/j.ijbiomac.2023.124528.
- Nandini DB, Rao RS, Hosmani J, et al. (2020). Novel therapies in the management of oral cancer: an update. Dis Mon 66:101036. doi: 10.1016/j.disamonth.2020.101036.
- Ossama M, Lamie C, Tarek M, et al. (2021). Management of recurrent aphthous ulcers exploiting polymer-based muco-adhesive sponges: in-vitro and in-vivo evaluation. Drug Deliv 28:87–99. doi: 10.1080/10717544.2020.1858999.
- Ou J, Gao Y, Li H, et al. (2022). Application of 5-aminolevulinic acid-mediated waterlase-assisted photodynamic therapy in the treatment of oral leukoplakia. Sci Rep 12:9391. doi: 10.1038/s41598-022-13497-3.
- Oyapero A, Oyapero O, Akinleye A. (2020). Burden of tobacco, kola nut and alcohol consumption and its association with periodontal disease, potentially malignant lesions and quality of life among bus drivers, Lagos State, Nigeria. Popul Med 2:1–9. doi: 10.18332/popmed/118726.
- Panatarani C, Praseptiangga D, Widjanarko PI, et al. (2023). Synthesis, characterization, and performance of semi-refined kappa carrageenan-based film incorporating cassava starch. Membranes 13:100. doi: 10.3390/membranes13010100.
- Pandey A, Chaturvedi M, Mishra S, et al. (2020). Reductive metabolites of curcumin and their therapeutic effects. Heliyon 6:e05469. doi: 10.1016/j.heliyon.2020.e05469.
- Panich AM, Salti M, Prager O, et al. (2021). PVP-coated Gd-grafted nanodiamonds as a novel and potentially safer contrast agent for in vivo MRI. Magn Reson Med 86:935–42. doi: 10.1002/mrm.28762.
- Paredes da Rocha N, de Souza A, Nishitani Yukuyama M, et al. (2023). Highly water-soluble dapsone nanocrystals: Towards innovative preparations for an undermined drug. Int J Pharm 630:122428. doi: 10.1016/j.ijpharm.2022.122428.
- Patel J, Maji B, Moorthy NSHN, Maiti S. (2020). Xanthan gum derivatives: review of synthesis, properties and diverse applications. RSC Adv 10:27103–36. doi: 10.1039/d0ra04366d.
- Peleg-Evron O, Davidovich-Pinhas M, Bianco-Peled H. (2023). Crosslinking konjac-glucomannan with kappa-carrageenan nanogels: a step toward the design of sacrificial materials. Int J Biol Macromol 227:654–63. doi: 10.1016/j.ijbiomac.2022.12.092.
- Pérez Zamora CM, Michaluk AG, Chiappetta DA, Nuñez MB. (2022). Herbal buccal films with in vitro antibacterial and anti-inflammatory effects. Journal of Herbal Medicine 31:100527. doi: 10.1016/j.hermed.2021.100527.
- Pınar SG, Oktay AN, Karaküçük AE, Çelebi N. (2023). Formulation strategies of nanosuspensions for various administration routes. Pharmaceutics 15:1520. doi: 10.3390/pharmaceutics15051520.
- Pourfarzad A, Ahmadian Z, Habibi-Najafi MB. (2018). Interactions between polyols and wheat biopolymers in a bread model system fortified with inulin: a Fourier transform infrared study. Heliyon 4:e01017. doi: 10.1016/j.heliyon.2018.e01017.
- Prasad R, Dalvi SV. (2020). Sonocrystallization: monitoring and controlling crystallization using ultrasound. Chem Eng Sci 226:115911. doi: 10.1016/j.ces.2020.115911.
- Pukale SS, Mittal A, Chitkara D. (2021). Topical application of vitamin D3-loaded hybrid nanosystem to offset imiquimod-induced psoriasis. AAPS PharmSciTech 22:238. doi: 10.1208/s12249-021-02116-5.
- Ravikumar R, Ganesh M, Senthil V, et al. (2018). Tetrahydro curcumin loaded PCL-PEG electrospun transdermal nanofiber patch: preparation, characterization, and in vitro diffusion evaluations. J Drug Delivery Sci Technol 44:342–8. doi: 10.1016/j.jddst.2018.01.016.
- Roi A, Roi CI, Negruțiu ML, et al. (2020). The challenges of OSCC diagnosis: salivary cytokines as potential biomarkers. J Clin Med 9:2866. doi: 10.3390/jcm9092866.
- Rosso A, Andretto V, Chevalier Y, et al. (2021). Nanocomposite sponges for enhancing intestinal residence time following oral administration. J Control Release 333:579–92. doi: 10.1016/j.jconrel.2021.04.004.
- Rudhziah S, Apandi NAA, Subban RHY, Mohamed NS. (2019). Ionic conductivity of biopolymer electrolytes based on seaweed kappa-carrageenan. Sci Lett 12(2):45–52. doi: 10.18332/popmed/118726.
- Said M, Haq B, Al Shehri D, et al. (2021). Modification of xanthan gum for a high-temperature and high-salinity reservoir. Polymers 13:4212. doi: 10.3390/polym13234212.
- Saini K, Modgill N, Singh K, Kakkar V. (2022). Tetrahydrocurcumin lipid nanoparticle based gel promotes penetration into deeper skin layers and alleviates atopic dermatitis in 2,4-dinitrochlorobenzene (DNCB) Mouse Model. Nanomaterials (Basel) 12:636. doi: 10.3390/nano12040636.
- Santos A M d, Meneguin AB, Fonseca-Santos B, et al. (2020). The role of stabilizers and mechanical processes on physico-chemical and anti-inflammatory properties of methotrexate nanosuspensions. J Drug Delivery Sci Technol 57:101638. doi: 10.1016/j.jddst.2020.101638.
- Shahine Y, El-Aal SAA, Reda AM, et al. (2023). Diosmin nanocrystal gel alleviates imiquimod-induced psoriasis in rats via modulating TLR7,8/NF-κB/micro RNA-31, AKT/mTOR/P70S6K milieu, and Tregs/Th17 balance. Inflammopharmacology 31:1341–59. doi: 10.1007/s10787-023-01198-w.
- Shaji J, Balakrishnan G, Halim N, et al. (2022). Oral potentially malignant disorders: mini review. JOENTR 14:44–7. doi: 10.15406/joentr.2022.14.00504.
- Shehata EMM, Gowayed MA, El-Ganainy SO, et al. (2022). Pectin coated nanostructured lipid carriers for targeted piperine delivery to hepatocellular carcinoma. Int J Pharm 619:121712. doi: 10.1016/j.ijpharm.2022.121712.
- Shiledar RR, Tagalpallewar AA, Kokare CR. (2014). Formulation and in vitro evaluation of xanthan gum-based bilayered mucoadhesive buccal patches of zolmitriptan. Carbohydr Polym 101:1234–42. doi: 10.1016/j.carbpol.2013.10.072.
- Shin M, Izumi S, Nakane PK. (1995). Multilayer peroxidase-labeled antibody method: comparison with labeled streptavidin-biotin method, avidin-biotin-peroxidase complex method, and peroxidase-antiperoxidase method. J Clin Lab Anal 9:424–30. doi: 10.1002/jcla.1860090615.
- Sokolova EV, Chusovitin EA, Barabanova AO, et al. (2013). Atomic force microscopy imaging of carrageenans from red algae of Gigartinaceae and Tichocarpaceae families. Carbohydr Polym 93:458–65. doi: 10.1016/j.carbpol.2012.12.026.
- Song G, Lu H, Chen F, et al. (2018). Tetrahydrocurcumin‑induced autophagy via suppression of PI3K/Akt/mTOR in non‑small cell lung carcinoma cells. Mol Med Rep 17:5964–9. doi: 10.3892/mmr.2018.8600.
- Soroushnia A, Ganji F, Vasheghani-Farahani E, Mobedi H. (2022). Effect of combined stabilizers on midazolam nanosuspension properties. Iran Polym J 31:215–22. doi: 10.1007/s13726-021-00981-2.
- Tang X, Dong Q, Li J, et al. (2021). Anti-melanogenic mechanism of tetrahydrocurcumin and enhancing its topical delivery efficacy using a lecithin-based nanoemulsion. Pharmaceutics 13:1185. doi: 10.3390/pharmaceutics13081185.
- Tang X, Zhang M, Zhang H, et al. (2021). Evaluation of the bioaccessibility of tetrahydrocurcumin-hyaluronic acid conjugate using in vitro and ex vivo models. Int J Biol Macromol 182:1322–30. doi: 10.1016/j.ijbiomac.2021.05.086.
- Trivedi MK, Panda P, Sethi KK, et al. (2020). Solid and liquid state characterization of tetrahydrocurcumin using XRPD, FT-IR, DSC, TGA, LC-MS, GC-MS, and NMR and its biological activities. J Pharm Anal 10:334–45. doi: 10.1016/j.jpha.2020.02.005.
- Truong TH, Alcantara KP, Bulatao BPI, et al. (2022). Chitosan-coated nanostructured lipid carriers for transdermal delivery of tetrahydrocurcumin for breast cancer therapy. Carbohydr Polym 288:119401. doi: 10.1016/j.carbpol.2022.119401.
- Tsai Y-Z, Tsai M-L, Hsu L-Y, et al. (2021). Tetrahydrocurcumin upregulates the adiponectin-adipor pathway and improves insulin signaling and pancreatic β-cell function in high-fat diet/streptozotocin-induced diabetic obese mice. Nutrients 13(12):4552. doi: 10.3390/nu13124552.
- Vairaktaris E, Spyridonidou S, Papakosta V, et al. (2008). The hamster model of sequential oral oncogenesis. Oral Oncol 44:315–24. doi: 10.1016/j.oraloncology.2007.08.015.
- Wang C, Wang M, Chen P, et al. (2022). Dasatinib nanoemulsion and nanocrystal for enhanced oral drug delivery. Pharmaceutics 14:197. doi: 10.3390/pharmaceutics14010197.
- Wang H, Khor TO, Shu L, et al. (2012). Plants vs. cancer: a review on natural phytochemicals in preventing and treating cancers and their druggability. Anticancer Agents Med Chem 12:1281–305. doi: 10.2174/187152012803833026.
- Wang Q, Yang X, Gu X, et al. (2022). Celecoxib nanocrystal-loaded dissolving microneedles with highly efficient for osteoarthritis treatment. Int J Pharm 625:122108. doi: 10.1016/j.ijpharm.2022.122108.
- Wang Y, Wang C, Zhao J, et al. (2017). A cost-effective method to prepare curcumin nanosuspensions with enhanced oral bioavailability. J Colloid Interface Sci 485:91–8. doi: 10.1016/j.jcis.2016.09.003.
- Wu X, Liu Y, Li X, et al. (2010). Preparation of aligned porous gelatin scaffolds by unidirectional freeze-drying method. Acta Biomater 6:1167–77. doi: 10.1016/j.actbio.2009.08.041.
- Xu C, Xiong Q-W, Li Y, et al. (2022). Explore the multitarget mechanism of tetrahydrocurcumin preventing on UV-induced photoaging mouse skin. Heliyon 8:e09888. doi: 10.1016/j.heliyon.2022.e09888.
- Yahoum MM, Toumi S, Tahraoui H, et al. (2023). Formulation and evaluation of xanthan gum microspheres for the sustained release of metformin hydrochloride. Micromachines 14:609. doi: 10.3390/mi14030609.
- Yapijakis C, Kalogera S, Papakosta V, Vassiliou S. (2019). The hamster model of sequential oral carcinogenesis: an update. In Vivo 33:1751–5. doi: 10.21873/invivo.11665.
- Yermak IM, Davydova VN, Kravchenko AO, et al. (2020). Mucoadhesive properties of sulphated polysaccharides carrageenans from red seaweed families Gigartinaceae and Tichocarpaceae. Int J Biol Macromol 142:634–42. doi: 10.1016/j.ijbiomac.2019.10.005.
- Youssef JR, Boraie NA, Ibrahim HF, et al. (2021). Glibenclamide nanocrystal-loaded bioactive polymeric scaffolds for skin regeneration: in vitro characterization and preclinical evaluation. Pharmaceutics 13:1469. doi: 10.3390/pharmaceutics13091469.
- Yoysungnoen B, Bhattarakosol P, Patumraj S, Changtam C. (2015). Effects of tetrahydrocurcumin on hypoxia-inducible factor-1α and vascular endothelial growth factor expression in cervical cancer cell-induced angiogenesis in nude mice. Biomed Res Int 2015:391748. doi: 10.1155/2015/391748.
- Yu W, Liu X, Cai D, et al. (2023). Regional distributions of curcumin and tetrahydrocurcumin in the liver and small intestine of rats when orally co-administered with quercetin and paeoniflorin. Biopharm Drug Dispos 44:183–91. doi: 10.1002/bdd.2346.
- Zhang X, Li Z, Gao J, et al. (2020). Preparation of nanocrystals for insoluble drugs by top-down nanotechnology with improved solubility and bioavailability. Molecules 25:1080. doi: 10.3390/molecules25051080.
- Zhang Y, Liu Y, Zou J, et al. (2017). Tetrahydrocurcumin induces mesenchymal-epithelial transition and suppresses angiogenesis by targeting HIF-1α and autophagy in human osteosarcoma. Oncotarget 8:91134–49. doi: 10.18632/oncotarget.19845.
- Zhang Z, Zhang R, Chen L, McClements DJ. (2016). Encapsulation of lactase (β-galactosidase) into κ-carrageenan-based hydrogel beads: Impact of environmental conditions on enzyme activity. Food Chem 200:69–75. doi: 10.1016/j.foodchem.2016.01.014.
- Zhao Y, Baeza JA, Koteswara Rao N, et al. (2014). Unsupported PVA- and PVP-stabilized Pd nanoparticles as catalyst for nitrite hydrogenation in aqueous phase. J Catal 318:162–9. doi: 10.1016/j.jcat.2014.07.011.
- Zhao Y, Li Y, Du Q, et al. (2019). Shape memory histocompatible and biodegradable sponges for subcutaneous defect filling and repair: greatly reducing surgical incision. J Mater Chem B 7:5848–60. doi: 10.1039/c9tb00902g.
- Zheng M, Lian F, Xiong Y, et al. (2019). The synthesis and characterization of a xanthan gum-acrylamide-trimethylolpropane triglycidyl ether hydrogel. Food Chem 272:574–9. doi: 10.1016/j.foodchem.2018.08.083.
- Zhou Y, Fang Q, Niu B, et al. (2018). Comparative studies on amphotericin B nanosuspensions prepared by a high pressure homogenization method and an antisolvent precipitation method. Colloids Surf B Biointerfaces 172:372–9. doi: 10.1016/j.colsurfb.2018.08.016.
- Zhu L, Xue Y, Feng J, et al. (2023). Tetrahydrocurcumin as a stable and highly active curcumin derivative: A review of synthesis, bioconversion, detection and application. Food Biosci 53:102591. doi: 10.1016/j.fbio.2023.102591.