Abstract
The prominence of cardiovascular diseases has prompted investigations into alternative treatment options, including tissue engineering. Currently, the biggest limitation in cardiac tissue engineering lies in delivering oxygen to all cells within the construct. Synthetic oxygen carriers hold much promise in that they have high affinity for oxygen and can be supplemented to culture medium without adverse effect on the cells. This review highlights two complementary studies by our group that utilized oxygen carriers in cardiac tissue engineering. Experimental and modeling studies were performed to evaluate the effect of a perfluorocarbon (PFC)-based synthetic oxygen carrier, OxygentTM, on oxygen supply within tissue engineered cardiac constructs. Porous biorubber scaffolds with an array of parallel channels mimicking the capillary network were seeded with cardiomyocytes and fibroblasts, and cultivated in medium supplemented with PFC. The presence of PFC enhanced the transport of oxygen, increased oxygen concentrations, and yielded constructs that displayed stronger cardiac-like phenotype.
INTRODUCTION
Cardiovascular disease is one of the leading causes of mortality in Canada and the United States. Current treatments for end-stage cardiovascular disease have not been effective at replacing diseased or damaged tissues and are limited by availability of donor implants and tissue degradation [Citation[1], Citation[2]]. Tissue engineering of cardiac tissues represents a promising alternative source for repair and regeneration of functional cardiac tissue. However, a major problem with cardiac tissue engineering arises from diffusion limitations associated with the poor solubility of oxygen in culture medium, leading to incomplete delivery of oxygen to cells within the three-dimensional tissue construct and thereby causing cell death [Citation[2-5]].
In the cardiac milieu, hemoglobin acts as a high affinity carrier of oxygen, maintaining a large oxygen reservoir to quickly replenish oxygen depleted from blood by the surrounding tissues. The use of artificial blood substitutes, such as culture medium supplemented with perfluorocarbons (PFCs), is now actively being explored for use in vitro to mimic the role of hemoglobin in vivo. PFCs have high affinity for oxygen and allow for high solubility of oxygen when emulsified in culture medium. The diffusion of oxygen from the PFC droplets into the aqueous phase constantly replenishes any oxygen depleted by the cells.
This review highlights two studies by our group that utilized artificial blood substitutes for cardiac tissue engineering. In the first study, a biomimetic tissue engineering strategy was employed using medium perfusion through porous channeled scaffolds, with or without PFCs. We tested the hypothesis that an in-vivo-like regime of oxygen supply will enable the in vitro cultivation of millimeters thick constructs with physiologic cell density [Citation[6]]. In the second study, mathematical modeling was used to rationalize the measured effects of PFC on engineered cardiac tissue. A steady state model of oxygen distribution was developed and solved using finite element analysis to investigate the effects of PFC supplementation on oxygen delivery to the cells [Citation[7]]. This model was used to define channel diameters and spacings and medium flow conditions for various scaffold thicknesses.
Both studies showed that oxygen availability and delivery to the tissue space was significantly improved in the presence of PFCs. Measured data document improved construct cellularity, contractile function and cell phenotype in the presence of PFCs. Modeling results showed that transport of oxygen was improved in the presence of PFC, due to an increase in both convective transport and effective diffusivity of oxygen. Thus, the use of artificial blood substitutes for cultivation of engineered tissue constructs represents an effective strategy in situations where a high oxygen carrying capacity is required, such as cardiac tissue engineering.
EXPERIMENTAL STUDY
The objective of these experiments was to establish convective-diffusive oxygen transport in engineered cardiac constructs in a configuration that resembled a capillary network. The strategy used to provide adequate oxygen to all parts of the construct involved perfusion of culture medium supplemented with an artificial oxygen carrier through channels. Cardiomyocytes and fibroblasts were isolated from neonatal rat hearts [Citation[5]]. Poly(glycerol-sebacate) (PGS) scaffolds were fabricated by a salt-leaching technique resulting in highly elastic, porous scaffolds with up to 91% porosity (C). Cylindrical channels mimicking the in vivo capillary network were engraved into the scaffolds using a computerized CO2 laser. The scaffolds were cut into 5–6 mm diameter by 2 mm thick discs and repeatedly sterilized with ethanol.
Figure 1 Schematics of the model system. (A) Perfusion loop, consisting of channeled biorubber scaffolds (7), perfusion cartridges (4), two debubbling syringes (5, 6), a multi-channel peristaltic pump (1), a gas exchanger (2), and the reservoir bag (3). (B) Modes of oxygen transport in the channeled construct perfused with culture medium include convection through the channel lumen and diffusion into the tissue space surrounding each channel. In unsupplemented culture medium (left), oxygen dissolved in the aqueous phase during gas exchange in the external loop is transported into the tissue phase and consumed by the cells. In culture medium supplemented with perfluorocarbon (PFC) emulsion (right), oxygen is replenished within the tissue construct by the release from PFC particles, a process governed by Henry's law. (C) SEM showing parallel channels and porous structure of scaffold (100X, scale bar is 500 µm). (D) Cross section of a single channel showing key modeling parameters (Rc = channel radius, Rt = tissue radius) and cylindrical geometry of channel (z-axis = axial coordinate, r-axis = radial coordinate).
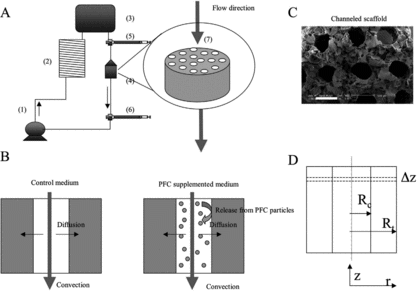
Since the majority of the cells in the native hearts are non-myocytes, a mixed cell population of fibroblasts and myocytes was used for tissue engineering. Scaffolds were first preconditioned by fibroblasts that were cultured for four days. Subsequently, cardiomyocytes were seeded into the same constructs and cultivated for 3 more days with unidirectional flow of culture medium. The constructs were fitted within polycarbonate perfusion cartridges surrounded on either side by stainless steel screens and silicone rings to provide mechanical support and ensure perfusion of medium only through the construct, and not around the construct. These cartridges were incorporated into perfusion loops consisting of a reservoir bag, a silicone tube gas exchanger, and a multi-channel peristaltic pump [Citation[5]] (A). The total volume of culture medium in the perfusion loop was 30 mL, and in the case of PFC supplemented medium, 3 mL of OxygentTM (Alliance Pharmaceuticals Corp, San Diego, CA) were added to 27 mL of culture medium. Constructs cultivated with unsupplemented culture medium served as controls.
The PFC droplets continually and quickly replenish any oxygen depleted in the aqueous phase due to the surrounding tissue layer (B). The concentration of circulating PFC was determined at the end of the construct cultivation by spectrophotometric analysis at 970 nm. Seven independent experiments were conducted, each producing 4–6 constructs.
Spectrophotometry revealed that the PFC volume fraction was ∼ 5.4%, slightly higher than the nominal fraction of 3.2%, due to some settling of the PFC emulsion in the loop. It was shown that pO2 was slightly lower in the PFC supplemented constructs, but that the pCO2 and pH were comparable to the control constructs. Lactate dehydrogenase levels (LDH), however, were significantly lower in the PFC constructs, indicating less cell damage. Total oxygen consumption in the control constructs between the inlet and outlet was 62 µM whereas in the PFC constructs it was 82 µM, indicating greater usage of oxygen by the cells. Excitation threshold was significantly lower in the PFC constructs, while maximum capture rates were comparable () suggesting better contractile function from improved oxygen delivery.
Table 1. Metabolic, contractile and biochemical properties of constructs cultivated with PFC emulsion and pure culture medium. * indicates significantly different than control medium by t-test, p < 0.05 considered significant. The values shown are Average±SE
DNA content was greater by nearly 60% in the PFC constructs (). Protein content was lower in the PFC-cultivated constructs, but the protein to DNA ratio was slightly lower than the control constructs. Scanning electron microscopy revealed a highly porous scaffold with average channel diameter and wall-to-wall spacing of around 377 µm and 222 µm, respectively (C). Both scaffolds were densely populated by cells, but the cell density was higher in the PFC-supplemented constructs. Staining for connexin-43 revealed gap junctions in all constructs. Staining for cardiac troponin I and vimentin revealed a mixed population of fibroblasts and cardiomyocytes, with cardiomyocytes being the dominant cell type. Image analysis of bands revealed a greater overall presence of cardiac tropinin I and connexin-43 in PFC-supplemented constructs compared to controls.
MODELING STUDY
Model Parameters
A steady state mathematical model was developed to describe the oxygen concentration profiles within the channels of the tissue construct [Citation[7]], assuming local equilibrium between the PFC and aqueous phase at every point along the channel. It was demonstrated that the internal diffusion as well as the oxygen diffusion from the PFC droplet into the culture medium was not rate-limiting. The main modes of oxygen transport in the channel lumen include convection in the axial direction and diffusion in the radial direction, governed by:where K is the partition coefficient, Vz (r) is velocity profile, ϕ is the volume fraction of PFC, Ca is oxygen concentration in the aqueous phase, and Deff is effective diffusivity defined as:
where Da is the diffusivity of O2 in the aqueous phase, Dp is the diffusivity of O2 in the PFC phase, γ = KDp/Da. Combining these equations yields the following conservation equation for the oxygen in the channel lumen:
The simplified governing equation for oxygen distribution in the tissue space, where oxygen consumption is assumed to follow Michaelis-Menten kinetics, is:where Ct is the oxygen concentration in the tissue space, Dt is diffusion coefficient in the tissue space, Vmax and Km are Michaelis-Mentent parameters. For the measured inlet and outlet oxygen concentration, the following boundary conditions were used:
For predictions of oxygen concentration profiles at conditions that were not obtained experimentally, the inlet and outlet oxygen concentration were not measured. Therefore, an alternative set of boundary conditions was used. It was assumed that the culture medium entering the perfusion cartridge was fully saturated with oxygen and Ca(r,0) = Ct (r,0) = 222.5 µM. The axial variations in the oxygen concentration cease to exist at the very short distance from the outlet of the channel array. Therefore,in the culture medium at the channel outlet. It was also assumed that the culture medium at the outlet was well mixed, with no variations in the radial direction. Therefore, the mixing cup concentration of the culture medium at the channel outlet was set to be the boundary condition for the concentration at the tissue space outlet.
The validity of the boundary conditions used for predictions was confirmed by comparing the oxygen concentration measured at the outlet of the experimentally obtained channeled tissue to the outlet values obtained using the described boundary conditions in the channel array of identical geometry, cell density and flow conditions.
A symmetry boundary condition was applied at the centerline of the channel lumen:
The region supplied by each channel was approximated by a cylinder. Since these cylindrical regions are equally spaced, a no flux boundary condition is applied at the half distance between channel centers (Rt), which is a common assumption in the well-known Krogh cylinder model.
Finally, the fluxes of oxygen and the concentrations have to match at the interface between channel lumen and tissue space, yielding the remaining two boundary conditions:
The model was solved for various channel geometries, flow rates and PFC emulsion volume fractions using the finite element method and a commercial software, Femlab. The results were expressed in terms of oxygen concentration in the aqueous phase [µM].
Experimentally obtained parameters were initially investigated and oxygen concentration profiles were generated for this system. The parameters included a channel diameter of 330 µm and a wall-to-wall spacing of 370 µm, perfused at an average linear velocity of 0.049 cm/s (0.1 mL/min bulk flow) and at 5.4 vol% of PFC emulsion. The Vmax was set to 10.5 µM/s (for PFC supplemented) and 8.8 µM/s (for pure culture medium) according to the measured protein content and reported maximum oxygen consumption per cell 27.6 nmol/mg protein/min [Citation[8]]. Aqueous phase oxygen concentrations at the inlet were comparable in the PFC-supplemented and control constructs in the channel lumen, but were lower in the tissue space in the PFC constructs, consistent with the higher cell densities in these constructs (). However, moving along the length of the construct, the oxygen concentration in the lumen and tissue space of the PFC-supplemented construct was observed to be higher in comparison to the control construct, demonstrating the benefit of PFC supplementation. The differences in oxygen concentration in the tissue space were only evident at small depths, however, and became negligible at larger depths ().
Figure 2 Oxygen concentration profiles generated by FEMLAB for experimentally derived parameters. Oxygen profile is shown for half of a channel width for (A) unsupplemented culture medium and (B) PFC-supplemented culture medium (5.4% v/v).
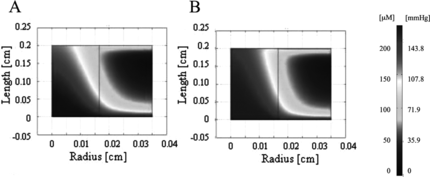
When the PFC concentrations were varied (0, 3.2% and 6.4% v/v PFC) while maintaining the geometry and flow rate (0.049 cm/s) from the experimental study, an overall increase in the oxygen concentration was observed with increasing PFC concentration in both the lumen and tissue space for both cell densities. However, even at the highest PFC concentration, at 100 µm away from the lumen, the oxygen concentration dropped several orders of magnitude for a physiological cell concentration of 108cells/cm3 in the tissue space ().
Figure 3 Log plot showing the oxygen concentration as a function of distance from the inlet at a position 100 µm away from the lumen. For a 330 µm channel diameter and 370 µm wall-to-wall spacing, at the highest PFC concentration of 6.4% v/v, and at a physiological cell density of 1 × 108 cells/cm3, the oxygen concentration drops several orders of magnitude along the length of the construct leading to sub-physiological oxygen levels throughout most of the tissue space.
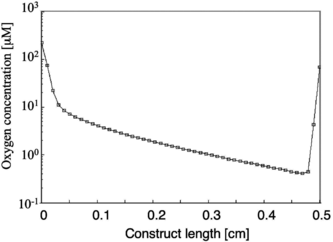
To address this issue, the geometry was optimized to a more closely packed one with a 100 µm channel diameter and 100 µm wall-to-wall spacing. As shown in , the oxygen concentration profile improved significantly when the fraction of PFC was increased from 0% to 6.4% v/v, but it was also necessary to increase the flow velocity from 0.049 cm/s to 0.135 cm/s to ensure all parts of the tissue space were being supplied adequately with oxygen. These flow rates had correspondingly normal wall shear stress values (∼ 1 dyne/cm2) that did not exceed values known to lead to decreased cell viability [Citation[9], Citation[10]]. Both the “coarse” and the “fine” geometry were fully compatible with the transport properties of blood [Citation[11]].
Figure 4 Oxygen concentration profile in the channel array. A 100 µm channel diameter and 100 µm wall-to-wall spacing was investigated in the model as an optimization to the in vitro parameters. The flow velocity was also increased in the range of 0.049 cm/s to 0.135 cm/s. A remarkable improvement in oxygen concentration is seen between (A) 0% PFC and (B) 6.4% PFC conditions.
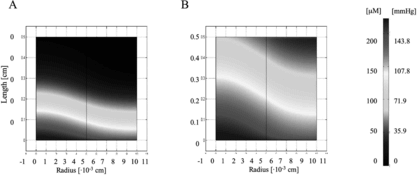
Analysis of the modeling parameters revealed that effective diffusivity of the medium was improved in the presence of PFC by up to 18% at the highest PFC fraction. Also, convective transport (apparent average velocity) was increased up to 123%. This was validated by looking at the contributions of the diffusive and convective contributions of PFC supplementation, alone and in combination, on volume averaged tissue oxygen concentration, minimum tissue oxygen concentration, and mixing cup culture medium oxygen concentration at the outlet for zero order oxygen consumption kinetics (18 µM/s). As shown in , a significant increase is seen in all three of these quantities with increasing PFC concentration. More strikingly, the majority of the effect is due to the convective contribution of the PFC supplementation, with only a slight contribution from diffusion.
Figure 5 Overall effect of PFC emulsion on oxygen concentration in the cardiac constructs. (A) Volume average oxygen concentration, (B) minimum oxygen concentration, and (C) mixing cup outlet concentration of oxygen in medium are shown as functions of PFC fraction (0, 3.2%, 6.4%). The contribution of PFC to the effective diffusivity (white bars) is compared to the contribution of PFC to the convective transport term (black bars), and the combination of both (grey bars). As shown, the effect of the PFC is dominated by its contribution to convective transport of oxygen.
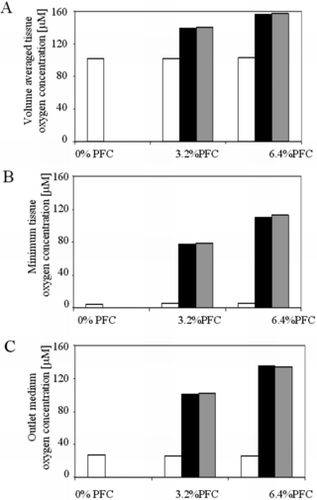
DISCUSSION
In the cardiac milieu, oxygen supply is of utmost importance in ensuring that tissues remain viable. This is facilitated by the dense capillary network feeding cardiac tissues and the high oxygen carrying capacity of blood due to the presence of hemoglobin. Here we have highlighted two important studies, which employ a biomimetic strategy to improve oxygen delivery to cultivated tissue engineered cardiac constructs. Cells grown on porous scaffolds with a capillary-like network of parallel channels and perfused with culture medium supplemented with a PFC-based synthetic oxygen carrier, OxygentTM, were metabolically, phenotypically and functionally healthier than those in control constructs. Constructs cultured in the presence of PFC also exhibited better contractile properties. The proposed mathematical model of the perfusion system used to grow these constructs showed that the presence of the PFC did, indeed, improved oxygen delivery within the tissue constructs. Such mathematical models may be useful tools in determining optimal conditions and geometries for further experimental studies.
The total oxygen content of PFC-supplemented culture medium, 5.4% v/v, was approximately two times higher than that of unsupplemented culture medium. Culture medium was perfused through the constructs at a velocity comparable to that found in the capillary network (560 µm/s). Oxygen depleted from the aqueous phase by respiring cardiomyocytes within the tissue space was replenished with oxygen dissolved in PFC droplets (B). Passive diffusion of oxygen from PFC droplets into the aqueous phase was very fast and was not a rate-limiting step.
The change in PO2 between the inlet and outlet was almost twice as high as that of the unsupplemented constructs, suggesting that the cells were availing of the large reservoir of oxygen. This is also consistent with the estimate of total oxygen content in PFC-supplemented medium being twice as high as control constructs. The higher availability of oxygen also markedly increased DNA content of the tissues. The lower levels of lactate dehydrogenase (LDH) release, lower L/G ratio, and higher ratio of oxygen/glucose consumed suggested PFC-supplemented cells were undergoing more aerobic respiration compared to unsupplemented controls. Protein content was also higher in the PFC supplemented constructs, whereas the oxygen consumption per unit protein was comparable in both groups. Lower excitation thresholds suggested better electrical coupling of the cells, which was also consistent with the higher cellularity and DNA content, and better maintenance of cardiac phenotype (as evidenced by higher cardiac troponin I and connexin-43 levels). SEM images revealed dense patches of cells within the channel, which may in part have contributed to the reduction in channel diameter at the end of the culture period.
A steady-state model was developed to produce oxygen concentration profiles in the lumen and tissue space of a single channel within the perfusion construct for various geometries, flow conditions and PFC concentrations. This model aided in visualizing the oxygen demands in the system and validating experimental findings. It also provided information on geometries that could not be experimentally tested due to limitations in experimental method. Thus, flow rates, channel diameter and spacing, and PFC concentration could be fine-tuned in silico to ensure adequate oxygen supply to all parts of the construct, providing the basis for further experimental studies in vitro.
The flow conditions used in the experiments and the mathematical model were chosen to mimic the low Re number flow seen in the microvasculature (Re ∼ 1). These flow conditions were selected to ensure that shear stresses did not exceed physiological thresholds reported in the literature (> 1.6 dynes/cm2) [Citation[9], Citation[10], Citation[12]] and to prevent dedifferentiation of cardiomyocytes at high shear stresses. Provided that these shear stress thresholds are not exceeded, then, in principle, the model can be used to predict oxygen profiles for a variety of geometries, flow rates, PFC concentrations, and cell densities to arrive at optimal parameters for experimental study.
Just as the shear stresses place an upper bound on the flow rates that can be used in an experimental study, a lower bound is placed on the dimension and spacing of the channels in the construct by considering the geometry of natural capillary beds, which have a 10 µm diameter and 20 µm wall-to-wall spacing. While it may be difficult to fabricate a scaffold with these micro-scale features and test it experimentally, such boundary conditions are of theoretical value and place a reality check on modeling parameters. Also, such geometries can be tested inexpensively in silico, avoiding costly or time-consuming fabrication processes unless they are absolutely necessary.
In the model study, the geometry closely matching the experimentally tested dimensions of 330 µm channel diameter and 370 µm wall-to-wall spacing provided a good visual picture of the oxygen profile in the channel. This profile showed that, in fact, the oxygen concentration profile within the tissue space was sub-optimal, with areas that were still largely sub-physiological in terms of oxygen concentration. A variety of PFC concentrations were investigated using this same geometry and it was observed that there was an improvement in the oxygen profiles with increasing PFC concentration, but this improvement was not sufficient to provide an adequate oxygen supply, largely because many of the original experimental parameters were still being retained. By varying the geometry of the scaffold and flow rate through the construct while still observing physiological upper and lower bounds placed on the system, it was possible to arrive at an optimal set of parameters that can be tested in the future. This illustrates the immense benefit of mathematical modeling in providing answers to questions that may not immediately be verifiable experimentally but would be of immense practical and theoretical interest in the future.
CONCLUSIONS AND FURTHER WORK
The establishment of convective-diffusive oxygen transport in channeled cardiac constructs is a viable option in engineering thick and contractile cardiac patches in vitro. An important area of future research includes overcoming the limitation associated with the laser cutting engraving system in order to produce a finer channel array (e.g. 100 µm diameter and 100 µm spacing). Adhesion peptides could be immobilized to the biorubber in order to improve the cell attachment properties. This would be advantageous to using collagen sponges, which have poor mechanical properties, are prone to swelling, and are not amenable to the creation of a channel array. While dense patches of cells were found within the channels, the absence of a continuous tissue layer between the channels might be addressed by seeding higher numbers of cells within the constructs, giving rise to further practical studies aimed at improving construct cellularity and viability.
REFERENCES
- Association, A.H. (2004). Heart Disease and Stroke Statistics-2004 Update.
- Bursac, N., , et al. (1999). Cardiac muscle tissue engineering: Toward an in vitro model for electrophysiological studies. American Journal of Physiology: Heart and Circulatory Physiology 277(46): H433–H444.
- Carrier, R.L., , et al. (1999). Cardiac tissue engineering: Cell seeding, cultivation parameters and tissue construct characterization. Biotechnology and Bioengineering 64: 580–589.
- Papadaki, M., , et al. (2001). Tissue engineering of functional cardiac muscle: molecular, structural and electrophysiological studies. American Journal of Physiology: Heart and Circulatory Physiology 280(Heart Circ. Physiol. 44): H168–H178.
- Radisic, M., , et al. (2003). High density seeding of myocyte cells for tissue engineering. Biotechnology and Bioengineering 82(4): 403–414.
- Radisic, M., , et al. (2003). Biomimetic Approach to Cardiac Tissue Engineering: Oxygen Carriers and Channeled Scaffolds. In review.
- Radisic, M., , et al. (2005). Mathematical model of oxygen distribution in engineered cardiac tissue with parallel channel array perfused with culture medium containing oxygen carriers: American Journal of Physiology: Heart and Circulatory Physiology 288(3): H1278–H1289.
- Yamada, T., , et al. (1985). Oxygen Consumption of mammalian myocardial—cells in culture—measurements in beating cells attached to the substrate of the culture dish. Analytical Biochemistry 145(2): 302–307.
- Stathopoulos, N.A., Hellums, J.D. (1985). Shear stress effects on human embryonic kidney cells in vitro. Biotechnology and Bioengineering 27: 1021–1026.
- Kretzmer, G., Schugerl, K. (1991). Response of mammalian cells to shear stress. Applied Microbiology and Biotechnology 34(5): 613–616.
- Radisic, M., Vunjak-Novakovic, G. (2005). Cardiac tissue engineering. Journal of the Serbian Chemical Society 70(3): 541–556.
- Fukuda, S., , et al. (2000). Mechanisms for regulation of fluid shear stress response in circulating leukocytes. Circulation Research 86(1): E13–E18.