Abstract
Polyhydroxyalkanoates (PHA) are a family of polyesters synthesized by microorganisms under unbalanced growth conditions. PHA including poly-3-hydroxybutyrate (PHB), copolymers of 3-hydroxybutyrate and 3-hydroxyvalerate (PHBV), poly-4-hydroxybutyrate (P4HB), copolymers of 3-hydroxybutyrate and 3-hydroxyhexanoate (PHBHHx), and poly-3-hydroxyoctanoate (PHO) are now available in sufficient quantity for tissue engineering medical application studies due to their reasonable biocompatibility, adjustable mechanical properties, and controllable biodegradability. This paper reviews many achievements based on PHA for medical devices development, tissue repair, artificial organ construction, drug delivery, and nutritional/therapeutic uses. Combined with the recent FDA approval for P4HB clinical application, one can expect a good prospect for PHA application in the medical fields.
INTRODUCTION
Polyhydroxyalkanoates (PHA) are a family of polyesters synthesized by microorganisms under unbalanced growth conditions. Once extracted from the cells, PHA exhibit thermoplastic and elastomeric properties Citation[1]. PHA can be divided into three subgroups based on the number of carbon atoms in the monomer units ( Citation[2]) incorporated into the polymers. PHA containing up to five carbon atom (C5) monomers are classified as short-chain-length PHA (scl-PHA). PHA with C6–C14 and >C14 monomers are classified as medium-chain-length (mcl-PHA) and long-chain-length (lcl-PHA) PHA, respectively Citation[3]. The changing compositions provide PHA with various physical properties () Citation[4]. The scl-PHA have properties close to conventional plastics polyethylene or polypropylene while the mcl-PHA are similar to elastomers and rubbers Citation[2], Citation[4], Citation[5].
Figure 1. General molecular structure of polyhydroxyalkanoates (Chen & Wu 2005). x=1, 2, 3, yet x = 1 is most common, n can range from 100 to several thousands. R is variable. When x = 1, R = CH3, the monomer structure is 3-hydroxybutyrate, while x = 1 and R = C3H7, it is a 3-hydroxyhexanoate monomer.
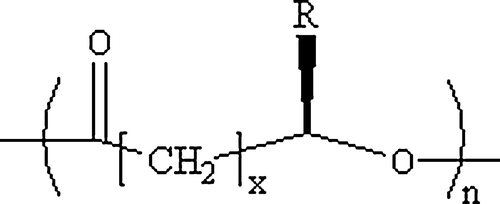
Table 1. Comparison of polymer properties (Khanna & Srivastava, 2005)
At present poly-3-hydroxybutyrate (PHB) and copolymers of 3-hydroxybutyrate and 3-hydroxyvalerate (PHBV) have been produced on a commercial scale Citation[4]. The relatively higher cost of PHA compared with polyethylene has limited their use as supplement to conventional plastics. Alternatively, they have been explored for tissue engineering and medical application due to their good biocompatibility, variable mechanical properties and controllable biodegradable ability under physiological conditions Citation[6]. So far, only several PHA including PHB, PHBV, poly-4-hydroxybutyrate (P4HB), copolymers of 3-hydroxybutyrate and 3-hydroxyhexanoate (PHBHHx), copolymers of 3-hydroxybutyrate and 4-hydroxybutyrate (P3HB4HB), and poly-3-hydroxyoctanoate (PHO) are available in sufficient quantity. Some of these PHA have undergone animal testing and in vivo tests for tissue responses Citation[7].
PHA can be blended, surface modified and/or composite with other polymers, inorganic materials and growth factors to adjust their biocompatibility, mechanical strength and degradation rates, depending on various requirements for different applications Citation[6], Citation[7]. Over the past years, PHA and its derivatives have been used to develop medical devices, fabricate matrix for tissue repair and artificial organ construction, carry drugs into the body and serve as nutritional and therapeutic composites Citation[6–8](). This review summarizes the many achievements of PHA application in medical fields.
MEDICAL DEVICES
Over the past many years, efforts have been made to develop PHA applications in medical devices, and many patents have been filed regarding this field (). PHA have been used to develop the following medical implant devices, including sutures, suture fasteners, meniscus repair devices, rivets, tacks, staples, screws (including interference screws), bone plates and bone plating systems, surgical mesh, repair patches, slings, cardiovascular patches, orthopedic pins (including bone filling augmentation material), adhesion barriers, stents, guided tissue repair/regeneration devices, articular cartilage repair devices, nerve guides, tendon repair devices, atrial septal defect repair devices, pericardial patches, bulking and filling agents, vein valves, bone marrow scaffolds, meniscus regeneration devices, ligament and tendon grafts, ocular cell implants, spinal fusion cages, skin substitutes, dural substitutes, bone graft substitutes, bone dowels, wound dressings, and hemostats. In order to obtain the United States Food and Drug Administration (FDA) approval, the endotoxin content in a particular PHA medical device should not exceed 20 US Pharmacopeia endotoxin units (EU) Citation[9].
Table 2. Patents for medical application of polyhydroxyalkanoates since 2000
One of the contributions of PHA to medicine has been in the cardiovascular area. Tepha Inc., based in Cambridge, MA, has been devoted to manufacturing pericardial patches, artery augments, cardiological stents, vascular grafts, heart valves, implants and tablets, sutures, dressings, dusting powders, prodrugs and microparticulate carriers using PHA Citation[10]. The first PHA-based product approved by the FDA for clinical application is the TephaFLEX® absorbable suture prepared from P4HB (http://www.tepha.com/). The most remarkable property of P4HB is its very high elasticity that benchmarks closely to ultrahigh molecular weight polyethylene; it can be stretched 10 times its original length before breaking Citation[11]. In 2007, the FDA had cleared its marketing in the US, indicating a bright future of PHA practical application in biomedical areas.
CARDIOVASCULAR TISSUE ENGINEERING
Cardiovascular diseases are a dominant cause of morbidity and mortality worldwide Citation[12]. Typically, surgical treatment is required, including patch closure, reconstruction of the defect or revascularization Citation[13]. The ideal cardiovascular patch material should have long durability, resistance to degradation and infection, non-toxicity, lack immunogenicity, have availability in a variety of sizes to suit cardiac and peripheral vascular reconstructions, with flexibility that allows easy suture and avoids interstitial bleeding following implantation Citation[14], Citation[15].
Heart Valves
Clinically applied heart valve replacements, including mechanical valves as well as xeno- or homo-grafts, have been used as implants, yet all are unable to grow or to repair the damaged tissues, thus child patients outgrow replacement valves and need repeated surgeries Citation[16]. The field of tissue engineering focusing on the regeneration of functional, living heart valve replacements uses materials configured in the shape of the heart valve with subsequent cell seeding Citation[17]. Several materials have been applied, including decellularized extracellular matrix Citation[18], Citation[19], polyglycolic acid (PGA) Citation[20], Citation[22], polylactic acid (PLA) Citation[20], polycaprolactone Citation[21] and PHA, to provide appropriate environments for cell growth and even differentiation.
Compared with PGA and PLA, mcl-PHA are more flexible materials, which are suitable to function as leaflets inside a tri-leaflet valve. Implantation of a whole trileaflet tissue-engineered heart valve using copolyester of 3-hydroxyhexanoate and 3-hydroxyoctanoate [P(3HHx-co-3HO)] and autologous cells in the pulmonary position in a lamb model was found to be efficient up to 120 days, with no thrombus formation and mild stenosis Citation[23]. Blending PGA with P(3HHx-co-3HO) showed even better results; there is no thrombus formation but mild, non-progressive, valvular regurgitation up to 6 months after implantation Citation[24]. In another study, by impregnating with biodegradable PHB, decellularized porcine aortic matrix/polymer hybrid tissue valves performed better biological properties including lower in vitro plasmatic coagulation cascades and less calcification Citation[25]. Most interestingly, some tissue engineered heart valves exhibited in vivo volume expansion with time. Twenty weeks after implantation of tri-leaflet heart valve produced from a PGA non-woven mesh solvent coated with P(4HB), the size of the valve increased from 19 to 23 mm with the growth of the lamb Citation[26], indicating the possibility to develop a valve that can grow with the growth of children.
Vascular Grafts
Vascular grafting is the alternative of cardiovascular pathologies in many situations. Compared with large diameter blood vessels, which are usually replaced with synthetic materials such as DarcronTM or expanded PTFE, small diameter grafts (<6 mm) have to confront the problem of incomplete endothelialization and rapid closure owing to low flow conditions Citation[27]. Many efforts have been made to solve this problem, such as UV activation treatment and coating Citation[28]. Advanced polyurethane graft material poly(carbonate-urea) (CPU) was demonstrated to have better compliance with natural vascular; it has passed the FDA clinical trial Citation[29].
The adjustable PHA monomer compositions allow the selection of favorable mechanical properties, biocompatibility, and degradation times; this expands the PHA application in cardiovascular tissue engineering Citation[30]. One of the most exciting events is the study of a P(4HB) patch with autologous cell seeding by Stock et al. Citation[31]. In their experiments, vascular cells isolated from ovine peripheral veins were seeded on porous P(4HB) patches and the patches were implanted into the proximal pulmonary artery in a sheep model. At 169 days, a near-complete resorption of the polymer with formation of organized and functional tissues was demonstrated without thrombus, stenosis or dilation, indicating avoidable re-operation and reduced damage to heart and blood vessels Citation[31]. Opitz et al. seeded vascular smooth muscle cells (vSMCs) onto P(4HB) scaffolds and incubated them in a pulsatile flow bioreactor. They observed confluent layered tissue formation and mechanical properties that approached those of native aorta Citation[32]. Moreover, P(3HHx-co-3HO) was evaluated as a component of an autologous cells seeding TE vascular graft in the systematic circulation in lambs. After 5 months, all of the PHA-PGA TE grafts remained unblocked without any aneurysms developed. The mechanical strain-stress curve of the TE aorta approached that of the native vessel Citation[33]. In the rat model, PHO showed good biocompatibility in terms of enzyme activity and tissue reaction accompanied by a slow in vivo degradation, suggesting its potential application in tissue engineering Citation[34].
PHBHHx is a newly developed member of the PHA family, with improved physical and chemical properties compared with PHB and PHBV Citation[35]. It was demonstrated that PHBHHx had improved hemocompatibility and cytocompatibility; it holds promise as a blood-contact material with less platelet adhesion, reduced erythrocyte contact and hemolysis reactivity compared with PHB and PHBV films Citation[36]. When smooth muscle cells from rabbit aorta (RaSMCs) were grown on PHBHHx containing 0–20% HHx (mol%), it was demonstrated that 20%-HHx containing PHBHHx supported not only RaSMCs proliferation, but also induced its change to contractile phenotype Citation[37]. Coincidentally, surface modification of PHBHHx using ammonia plasma treatment and/or fibronectin coating improved these results, demonstrated by better growth of human umbilical vein endothelial cells (HUVECs) and RaSMCs () Citation[38]. On fibronectin coating and plasma treated PHBHHx film (PFn-PHBHHx) film, HUVECs formed a confluent monolayer after 3 days of incubation, while SMCs were unable to form a sub-confluent layer, indicating that PFn-PHBHHx benefits endotheliazation rather than SMCs proliferation, and thus holds promise as a material for luminal surface and vascular grafting Citation[38]. However, further investigation including vascular replacement in animal models should be carried out to evaluate the possible clinical application of PHBHHx in vascular tissue engineering.
Figure 3. Fibronectin (green) distribution and actin filament formation (red) in SMCs cultured on test films(Qu et al. 2005). (A) SMCs cultured on PHBHHx stained with anti-fibronectin revealed an intense, granular and an oriented delicate fibrillar extracellular labeling pattern. Fibronectin was co-localized with actin filaments clearly (arrow). (B) SMCs on P-PHBHHx stained with anti-fibronectin revealed an intense, perinuclear, granular labeling pattern. Fibronectin was co-localized with actin filaments obviously (arrow). (C) SMCs on Fn-PHBHHx stained with anti-fibronectin revealed a random granular labeling pattern. Fibronectin revealed almost no co-localization with actin filaments (arrow). (D) SMCs cultured on PFn-PHBHHx stained with anti-fibronectin revealed a tense, granular, perinuclear labeling pattern. The co-localization between fibronectin and actin was impaired in some cells (blue arrow) while normal in others (white arrow). (A) PHBHHx; (B) P-PHBHHx; (C) Fn-PHBHHx; (D) PFn-PHBHHx (confocal microscopy, LSM510 meta, Zeiss).
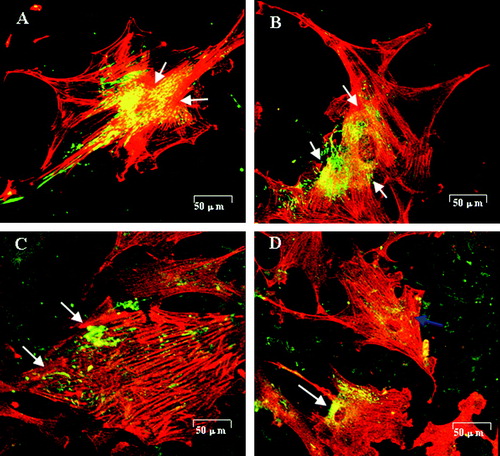
So far, the primary challenge for the application of many materials in vascular tissue engineering remains the compliance mismatch Citation[28]. Several approaches have been developed including electrospining Citation[39] and 3D weaving technique Citation[40] for manufacturing of scaffolds with appropriate mechanical properties. It is believed that the application of these new PHA processing and surface modification methods will significantly improve their biomechanical properties, and extend their application in cardiovascular tissue engineering.
NERVE CONDUITS TISSUE ENGINEERING
The clinical technique for the reconstruction in a peripheral nerve injury is still the use of autologous nerve grafts in recent years, which has inevitable disadvantages such as extended surgery, loss of donor nerve function, limited supply of donor nerves, fascicular organization between lesioned nerve and graft Citation[41]. Recently, efforts have been made to develop the synthetic nerve conduits for the repair of peripheral nerve defects, and different types of biomaterials have been investigated, such as PGA Citation[41], PLGA Citation[42], Citation[43], collagen Citation[44–48], chitosan Citation[41], Citation[44], Citation[49–51] and silk Citation[52], Citation[53]. It is believed that an ideal biodegradable conduit should have the following properties including maintaining tissue structure integrity, permitting cell communication and subsequent tissue ingrowth during the regenerative processes Citation[43]. The mechanical property and biocompatibility of the material are vital factors in the nerve tissue engineering.
PHB conduits have been successfully used to repair a 10-mm gap in the rat sciatic nerves; good regeneration and biocompatibility were observed Citation[54]. To improve applicability of PHB nerve conduits, extracellular matrix (ECM), Schwann cells and nerve growth factors were used to modify the grafts Citation[55–58]. Schwann cells grown in the inner surface of PHB conduits could retain their ability to enhance nerve regeneration. These bioengineered nerve conduits provided an ideal environment for optimal regeneration following peripheral nerve injury Citation[57]. Modified PHB nerve conduits were successfully used to repair the long defects in rabbit common-peroneal-nerve injury model Citation[59–61]. PHB filled with glial growth factor (GGF) suspended in alginate hydrogel was used to bridge the gaps of 2–4 cm in rabbit common peroneal nerve; the results proved that PHB conduits supported peripheral nerve regeneration up to 63 days, suggesting PHB nerve conduits are suitable for long-gap nerve injury repair Citation[61]. PHBHHx had also been demonstrated to be biocompatible to nerve cells Citation[62].
Biodegradability is also an indispensable factor for the nerve conduit. The degradation rates should match that of new nerve regeneration. Although degradation rates of PHB and PHBHHx scaffolds can be controlled by modulating ratios of materials of different molecular weights, the degradation of PHB and PHBHHx were shown to be always slower compared with the speed of new tissue formation Citation[59–61]. Thus, long-term research should be conducted to develop PHA materials that promote tissue regeneration accompanied by an appropriate degradation rate based on tissue regeneration speed.
BONE TISSUE ENGINEERING
The major function of bone tissue is to support the body weight. Adult bones consist of 80% cortical (or compact) bones and 20% cancellous (or trabecular) bones. The proportions of two forms differ at various locations in the skeleton. Cortical bone has only 10% porosity, with spaces only for osteocytes, canaliculi and blood vessels. Cancellous bone presents a higher porosity of 50–90%, making it lighter and less dense Citation[63], Citation[64].
When a high mechanical strength is required for a hard tissue like bone tissues, researchers mainly focus on scl-PHA like PHB and PHBV. Meanwhile, hydroxyl apatite (HA), which contributes 65–70% to the bone matrix, is commonly used as an additional blending material. Some in-vitro studies Citation[65–69] showed that mechanical properties of the composite could be changed by varying the amount of HA. Galego et al. Citation[70] reported that P(HB-co-8%HV)/HA (30% w/w) had a mechanical compression strength of 62 MPa, which is about the same order of magnitude of several human bones. The best parameters of growth and differentiation of murine marrow osteoblasts were found on PHB/HA blends containing 10% and 20% HA Citation[71]. Nano-sized HA reinforcing PHBV had even lower inflammatory response and higher levels of mineralization compared with other materials used Citation[72]. PHBHHx had better performances on attachment, proliferation, and differentiation of osteoblasts Citation[73], Citation[74] compared with PHB. However, PHBHHx scaffolds containing of HA particles did not lead to an improvement in mechanical properties or osteoblast responses Citation[75].
For in vivo study, PHB and PHB reinforced with particulate HA were demonstrated to produce consistent favorable bone tissue adaptation response with no evidence of an undesirable chronic inflammatory response after an implantation period up to 12 months Citation[76]. Highly organized new bone was rapidly formed close to the implant material Citation[77]. Osteoblasts and osteocytes were identified throughout the interface region in a rabbit tibias implantation study using PHBV/HA Citation[78]. Lamellar bone formed at the interface accompanied by polymer matrix degradation. The thickness of the newly formed bone significantly increased over the period of the experiment from 130 µm at 1 month to 770 µm after 6 months Citation[78]. Bone marrow stromal cells (BMSC) exhibited osteoblastic phenotype in vitro together with increasing ALP and osteocalcin secretion on PHBV foams Citation[79]. When preseeded on the PHBV scaffolds, the BMSC containing scaffolds were found to help in vivo tissue repair Citation[79]. The repair effects of PHBV were compared to calcium phosphate-loaded collagen (CaP-Gelfix). Better healing, less fibrous tissue formation with PHBV matrices than with CaP-Gelfix in 3 weeks was demonstrated macroscopically and radiologically Citation[79].
Other bioactive materials such as tricalcium phosphate (TCP) Citation[80], Citation[81], sol-gel-bioactive glass (SGBG) Citation[81], Citation[82], natural coral (NC) Citation[83], Citation[84], wollastonite (W) Citation[85], Citation[86], were used to blend with PHB or PHBV. Similar biomineralized deposit layers were formed on the surface of these hybrid scaffolds; they were determined to be the bone-like crystalline hydroxyl-carbonate-apatite. Functional evaluation for bone replacement needs to be applied to provide further improvement information for tissue engineered PHA bones.
CARTILAGE TISSUE ENGINEERING
Articular cartilage tissue with chondrocytes as the exclusive cell type covers the ends of bones in diarthrodial joints for distribution of applied loads. It has the lowest volumetric cellular density of any tissue in the human body. Once damaged, cartilage tissue has a very limited capacity to heal itself, which leads to osteoarthritis and functional loss of the joints. Traditional surgical treatments, such as subchondral drilling, arthroscopic abrasion, and microfracture technique, can never thoroughly revert the damage Citation[87], Citation[88]. To regenerate long-lasting hyaline cartilage in the defects, porous three-dimensional scaffolds made from PGA Citation[89], PLA Citation[90], PLGA Citation[91], Citation[92], chitosan Citation[93], Citation[94], silk fibroin Citation[95], collagen Citation[96] and PHA were used to provide appropriate environments biochemically and biomechanically for cell proliferation and tissue infusion.
Biochemical environments for cell growth can be evaluated by cell proliferation and extracellular matrix (ECM) production. In vitro chondrocytes proliferated and preserved their phenotype on PHB, PHBHHx, PHBHHx/PHB blends Citation[97] and PHBV Citation[98]. They proliferated better on the PHBHHx/PHB scaffolds than on PHB ones Citation[97]. Scaffolds made of PHBHHx/PHB consisting of 60 wt% PHBHHx showed strong growth and proliferation of chondrocytes on the blending materials Citation[99]. PHBHHx had a positive effect on ECM production of chondrocytes in the composite PHB/PHBHHx scaffolds. Second-harmonic generation (SHG) imaging technique combined with confocal fluorescence microscopy (CFM) revealed that PHBHHx in PHB scaffold provided better surface properties for anchoring type II collagen filaments and their penetration into internal layers of the scaffolds Citation[100]. Besides this, crystallinity of the blended polyesters remarkably influenced phenotype maintenance of chondrocytes. The amount of extracellular collagen X, which is the marker of endochondral ossification, decreased with increasing polarity contributed by increasing PHBHHx content in the blend Citation[101].
In vivo tissue repair was investigated using PHBV matrices implanted into full thickness cartilage defects (4.5 mm in diameter and 4 mm in depth) compared with collagen containing calcium phosphate (CaP-Gelfix). PHBV matrices presented early cartilage formation resembling normal articular cartilage with minimal foreign body reaction. PHBV had better healing response than CaP-Gelfix Citation[102]. Very recently, three-dimensional PHBHHx scaffolds with or without chondrocytes seeded were evaluated in rabbit articular cartilage defect model. During 16 weeks implant in vivo, the defects were found to be filled with white cartilaginous tissue. Owing to pre-seeded chondrocytes in the PHBHHx scaffolds, better surface integrality and more accumulation of ECM including type II collagen and sulfated glycosaminoglycan (sGAG) were achieved in the engineered cartilage constructs. The repaired tissues possessed an average compressive modulus of 1.58 MPa. No side effect of scaffold remained in the defects after 16 weeks Citation[103]. However, a longer time implantation study needs to be performed to examine carefully the degradation behavior of the scaffolds and their effects to regenerated tissues.
So far, challenges of engineered cartilaginous tissues are still the bio- and mechanical properties of the implant constructs. PGA-based 3D weaving technique for making scaffolds with mechanical properties of the same order of magnitude as that of the native articular cartilage have been developed Citation[89]. It is expected that the new material processing methods combined with other material modification approaches will be applied to PHA for the enhancement of elascity and biocompatibility.
DRUG DELIVERY SYSTEMS
Polymers play a central role in control of drug release and fabrication of drug delivery devices. The need for polymers with specific physical and biological properties has generated great interest in novel polymer synthesis and its application in drug delivery. PHA have various chemical compositions and functionalized groups in the side chain that allows further chemical modification; they can also be applied for various drug delivery devices Citation[104].
Fine control of degradation rate of a polymer is necessary for control drug release. A variety of strategies can be used. Block copolymers with a biodegradable element as additives are typically used. Such block copolymerization offers the opportunity to change the surface properties of solid polymers, and hence to modify the drug delivery properties Citation[105]. PEGylation of PHA is one example, which provides techniques for control of PHA composition, molecular mass, and their mechanical properties. It has been demonstrated that such techniques include forming natural-synthetic hybrids through the addition of PEG to microbial cultivation systems and chemical conjugation with PEG Citation[106]. Mcl-PHA copolymers are particularly interesting candidates for drug delivery in view of their lower melting point and lower degree of crystallinity Citation[104]. Martin et al. have demonstrated extremely favorable mechanical properties, biocompatibility, and proper degradation rates of P(4HB) Citation[107]. In addition, methods for manufacturing the devices may alter the degradability of PHA. There are many techniques to increase porosity, such as emulsion technique, spray drying technique, or incorporation of leachable or lyophilizable particles into the polymer matrices Citation[30].
Development of drug delivery systems (DDSs) must consider the drug encapsulation, DDS uptake via endocytosis, its retaining, drug release and drug activation in vivo. A high affinity to drugs, a relatively long half-life in the blood, and high specificity to target tissues and cells are main factors to be taken into consideration when developing a DDS. Langer et al. succeeded in increasing blood circulation times of PHA polymeric nanospheres by conjugating PHA with PEG Citation[108]. PHA are biocompatible and hydrophobic; they can also be turned into films, porous matrices, microcapsules, microspheres and nanoparticles. Drugs can be entrapped or microencapsulated in a PHA homopolymer or copolymer Citation[109]. Microcapsule structure for drug delivery could be designed to contain external and internal PHA layers where at least one layer was added with magnetic substance Citation[110]. Furthermore, a new method for bionanofabrication of PHA micro-/nano-structures on solid surfaces was developed Citation[111]. In this method, PHB polymer synthesized on gold surfaces formed very distinct and uniform granular structures with microscale size. In addition to PHB biocompatible surface coatings, the novel PHB polymeric micro-/nano-structures were built in spaces that cannot be accessed by convention lithographic tools or other fabrication process Citation[111]. On the other hand, PHA may be a potential candidate in treating highly resistant infections, as demonstrated, PHA drug delivery systems showed the ability for provision and maintenance of adequate concentrations of antibiotics at infection sites Citation[112]. PHB, PHBV, and P(3HB-4HB) were shown to be useful in the construction of biodegradable, implantable rods for the local delivery of antibiotics in chronic osteomyelitis therapy Citation[113–115].
In addition to DDS, studies on the application of PHA, especially mcl-PHA to transdermal drug delivery systems (TDDS), were also been reported. Drugs currently present in clinical TDDS are limited to hydrophobic ones with low molecular weights that are effective at low doses; this is because the hydrophobic stratum corneum represents a major barrier against hydrophilic ionizable drugs. Mcl-PHA with various monomer compositions and versatile properties, such as hydrophobicity, biocompatibility and low glass transition temperature (Tg), have attracted many interests in this area. Drugs encapsulated in mcl-PHA showed good adhesiveness to the skin model used, and dispersed well in all model drugs tested; the drugs also permeated well through snake skin from the PHA matrix Citation[116], Citation[117].
NUTRITIONAL AND THERAPEUTIC USES
Since PHA are generally degradable under physiological conditions, the cytotoxicity of PHA main degradation products was evaluated in vitro. Oligo(3-hydroxybutyrate) (OHB), oligo(3-hydroxybutyrate-co-4-hydroxybutyrate) (O3HB4HB), oligo (3-hydroxybutyrate-co-3-hydroxyhexanoate) (OHBHHx), and medium-chain-length oligo(3-hydroxyalkanoates) (OmclHAs) as insoluble particles were encapsulated in liposomes and transferred into cytosol of murine fibroblast L929 Citation[118]. The results showed that OHAs in concentration lower than 20 mg/l did not significantly affect cell viability, while OHAs over 40 mg/l reduced cell viability with more cell apoptosis, more cell death, delayed cell cycle, and reduced cell proliferation. The cytotoxicity of OHAs decreased with increasing OHAs side chain length Citation[118]. 3-hydroxybutyrate (3HB) monomer promoted proliferation of L929 cells in high-density cultures by preventing apoptotic and necrotic cell death Citation[119]. It also promoted cell proliferation of human umbilical vein endothelial cells and rabbit articular cartilage chondrocytes through a stimulatory effect on cell cycle progression that was mediated by a signaling pathway dependent upon increases in [Ca2 +] Citation[120].
As one of the ketone bodies in the blood, 3HB has been demonstrated as an alternate source of energy in brains when glucose supply is depleted Citation[121], Citation[122]. The dimer and trimer of 3HB in solution were converted completely to monomers when incubated with rat serum or liver homogenate for 10 minutes [123[. The mixture was also hydrolyzed by human liver homogenate but not by serum. 3-Hydroxybutyrate oligomers could be an energy substrate Citation[123]. 3HB had been shown to have a protective effect against 1-Methyl-4-Phenylpyridine (MPP) and beta-amyloid1-42 toxicity in cultured neurons Citation[124–126]. Sodium gamma-hydroxybutyrate (GHB-Na) administration suppressed the increasing expression of N-methyl-D-aspartate subunit (NR2B) mRNA in the rat hippocampus and protected rat neonatal brain against hypoxia-ischemia (HI) Citation[127]. The neuroprotective mechanism was demonstrated that 3HB supported the mitochondrial respiration system by reversing the inhibition of complex I or II Citation[128]. A recent study showed that 3HB and its derivatives prevented glial cells from apoptosis () Citation[129].
Figure 4. Glial cell apoptosis study by Annexin V-FITC staining assay (Xiao, Zhao, & Chen 2007). Glial cells at an initial concentration of 105 cells/well were grown in DMEM medium supplemented with 20% FBS for 24 h. After the cells attached on the bottom of the wells, the culture medium was replaced by DMEM medium. D-3-HB, DL-3-HB and M-3-HB were all added in 10 mM to the culture medium, respectively. After 48 h, the cells were harvested and stained by Annexin V-FITC and PI. The dead cells were stained by PI and the cells in apotosis were stained by Annexin V-FITC and allocated in the D4 region. (A)—(a) control: cells cultured with DMEM medium; (b) cells cultured with DMEM supplemented with 10mM D-3-HB; (c) cells cultured with DMEM supplemented with 10mM DL-3-HB; (d) cells cultured with DMEM supplemented with 10mM M-3-HB. (B) The percentage of cell apoptosis: statistic result obtained by averaging 6 flow cytometry results of each group in (A).
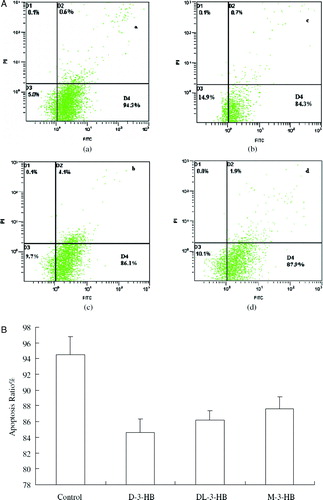
In another therapeutic study, 3HB had been proved to support in vitro growth and differentiation of murine osteoblast MC3T3-E1 cells. Oral administration of 3HB increased serum alkaline phosphatase (ALP) activity and calcium deposition; it decreased serum osteocalcin (OCN), and prevented reduction on bone mineral density (BMD) resulting from ovariectomy in female Wistar rats Citation[130]. 3-hydroxyacids could be administered orally or intravenously, as a nutritional or dietary supplement Citation[122]. An enhanced blood ketone level is beneficial for seizure control, metabolic disease control, reduction of protein catabolism, appetite suppression, parenteral nutrition, promotion on cardiac efficiency, treatment of diabetes and insulin resistant states, as well as treatment on neurodegenerative disorders and epilepsy Citation[122].
CONCLUSIONS
PHA and their derivatives were demonstrated by many studies to be useful materials for medical application. Their biocompatibility, mechanical strength, degradation rates, and other properties can be adjusted by monomer structures and ratios, by blending, surface modification and/or composition with other polymers, inorganic materials and growth factors to meet various requirements for different tissue engineering fields. Along with novel PHA production and material processing advancement, it is believed that more functions of PHA in medical fields will be developed in the very near future.
Acknowledgements
The research was supported by National High Tech 863 Grant (Project No. 2006AA02Z242 and 2006AA020104), as well as the State Basic Science Foundation 973 (2007CB707804). The Guangdong Provincial Grant for collaboration among industry, university, and research organizations awarded to GQC has also contributed to this study. The Multidisciplinary Research Center at Shantou University where some of the studies were conducted has been supported by Li Ka-Shing Foundation.
References
- Steinbüchel A., Hustede E., Liebergesell M., Pieper U., Timm A., Valentin H. FEMS Microbiol Rev 1992; 9: 217–230
- Chen G. Q., Wu Q., Xi J. Z., Yu H. P. Prog Nat Sci 2000; 10: 843–850
- Suriyamongkol P., Weselake R., Narine S., Moloney M., Shah S. Biotechnol Adv 2007; 25: 148–175
- Khanna S., Srivastava A. K. Process Biochem 2005; 40: 607–619
- Li R., Zhang H. X., Qi Q. S. Bioresource Technol 2007; 98: 2313–2320
- Chen G. Q., Wu Q. Biomaterials 2005; 26: 6565–6578
- Valappil S. P., Misra S. K., Boccaccini A. R., Roy I. Expert Rev Med Devic 2006; 3: 853–868
- Williams, S. F., Martin, D. P., and Skraly, F. A. 2005. US Patent No. 6838493.
- Williams S. F., Martin D. P., Horowitz D. M., Peoples O. P. Int J Biol Macromol 1999; 25: 111–121
- Philip S., Keshavarz T., Roy I. J Chem Technol Biot 2007; 82: 233–247
- Martin D. P., Williams S. F. Biochem Eng J 2003; 16: 97–105
- Morosco, Gregory J. Prev Cardiol 2002; 5: 31–36
- Kofidis T., et al. Eur J Cardio-Thoracic 2002; 22: 238–243
- Valappil S. P., Misra S. K., Boccaccini A. R., Roy I. Expert Rev Med Devic 2006; 3: 853–868
- Vara D. S., Salacinski H. J., Kannan R. Y., Bordenave L., Hamilton G., Seifalian A. M. Pathol Biol 2005; 53: 599–612
- Schmidt D., Hoerstrup S. P. Swiss Med Wkly 2006; 136: 618–623
- Schmidt D., Stock U. A., Hoerstrup S. P. Philos T R S B 2007; 362: 1505–1512
- Rieder E., Kasimir M. T., Silberhumer G., Seebacher G., Wolner E., Simon P., Weigel G. J Thorac Cardiov Sur 2004; 127: 399–405
- Schenke-Layland K., et al. Cardiovasc Res 2003; 60: 497–509
- Engelmayr G. C., Hildebrand D. K., Sutherland F. W. H., Mayer J. E., Sacks M. S. Biomaterials 2003; 24: 2523–2532
- Hutmacher D. W., Schantz T., Zein I., Ng K. W., Teoh S. H., Tan K. C. J Biomed Mater Res 2001; 55: 203–216
- Sodian R., Hoerstrup S. P., Sperling J. S., Martin D. P., Daebritz S., Mayer J. E., Vacanti J. P. Asaio J 2000; 46: 107–110
- Sodian R., et al. Circulation 2000; 102(19)22–29
- Stock U. A., et al. J Thorac Cardiov Sur 2000; 119: 732–740
- Stamm C., et al. Ann Thorac Sur 2004; 78: 2084–2092
- Hoerstrup S. P., et al. Circulation 2000; 102: 44–49
- Cassell O. C. S., Hofer S. O. P., Morrison W. A., Knight K. R. British J Plast Sur 2002; 55: 603–610
- Salacinski H., Tiwari A., Hamilton G., Seifalian A. M. J Biomed Mater Res 2002; 61: 337–338
- Tiwari A., Salacinski H., Seifalian A. M., Hamilton G. Cardiovasc Res 2002; 10(3)191–197
- Chen G. Q., Wu Q. Biomaterials 2005; 26: 6565–6578
- Stock U. A., et al. J Thorac Cardiov Sur 2000; 120: 1158–1167
- Opitz F., Schenke-Layland K., Richter W., Martin D. P., Degenkolbe I., Wahlers T., Stock U. A. Ann Biomed Eng 2004; 32: 212–222
- Shum-Tim D., et al. Ann Thorac Sur 1999; 68: 2298–2304
- Marois Y., Zhang Z., Vert M., Beaulieu L., Lenz R. W., Guidoin R. Tissue Eng 1999; 5: 369–386
- Yoshie N., Fujiwara M., Kasuya K., Abe H., Doi Y., Inoue Y. Macromol Chem Phys 1999; 200: 977–982
- Qu X. H., Wu Q., Chen G. Q. J Biomat Sci-Polym E 2006; 17: 1107–1121
- Qu X. H., Wu Q., Liang J., Zou B., Chen G. Q. Biomaterials 2006; 27: 2944–2950
- Qu X. H., Wu Q., Liang J., Qu X., Wang S. G., Chen G. Q. Biomaterials 2005; 26: 6991–7001
- Ashammakhi N., et al. J Nanosci Nanotechno 2006; 6: 2693–2711
- Lee J., Cuddihy M. J., Kotov N. A. Tissue Eng Rev 2008; 14: 61–86
- Wang X. D., Hu W., Cao Y., Yao J., Wu J., Gu X. S. Brain 2005; 128: 1897–1910
- Liu B., et al. J Mater Sci-Mater M 2008; 19: 1127–1132
- Oh S. H., et al. Biomaterials 2008; 29: 1601–1609
- Kim S. W., Bae H. K., Nam H. S., Chung D. J., Choung P. H. Macromol Res 2006; 14: 94–100
- Kose G. T., Korkusuz F., Korkusuz P., Hasirci V. Tissue Eng 2004; 10: 1234–1250
- Li W. S., et al. J Mater Sci-Mater M 2008; 19: 847–854
- Liu B., et al. J Mater Sci-Mater M 2008; 19: 1127–1132
- Schnell E., Klinkhammer K., Balzer S., Brook G., Klee D., Dalton P., Mey J. Biomaterials 2007; 28: 3012–3025
- Gang, X. , et al 2007. 2007 International Forum on Biomedical Textile Materials, Proceedings 275–282.
- Wang A. J., et al. Biotechnol Lett 2007; 29: 1697–1702
- Zhang P., Xu H., Zhang D., Fu Z., Zhang H., Jiang B. Artif Cell Blood Sub 2006; 34: 89–97
- Chen X. M., Yang Y. M., Wu J., Zhao Y. H., Ding F., Gu X. S. Prog Nat Sci 2007; 17: 1029–1034
- Yang Y., Ding F., Wu H., Hu W., Liu W., Liu H., Gu X. Biomaterials 2007; 28: 5526–5535
- Hazari A., Wiberg M., Johansson-Ruden G., Green C., Terenghi G. Brit J Plast Surg 1999; 52: 653–657
- Mosahebi A., Wiberg M., Terenghi G. Tissue Eng 2003; 9(2)209–218
- Armstrong S. J., Wiberg M., Terenghi G., Kingham P. J. Tissue Eng 2007; 13: 2863–2870
- Terenghi G., Mosahebi A. Glial Interfaces in the Nervous System 2002; 47: 13–20
- Goldspink, G. and Terenghi, G. 2002. US Patent No. 2002083477.
- Mohanna P. N., Terenghi G., Wiberg M. Scand J Plast Recons 2005; 39: 129–137
- Young R. C., Wiberg M., Terenghi G. Brit J Plast Surg 2002; 55: 235–240
- Mohanna P. N., Young R. C., Wiberg M., Terenghi G. J Anat 2003; 203: 553–565
- Yang Y., Li X., Li G., Zhao N., Zhang X. Journal of Modern Clinical Medical Bioengineering 2002; 19: 25–29
- Salgado A. J., Coutinho O. P., Reis R. L. Macromol Biosci 2004; 4: 743–765
- Sikavitsas V. I., Temenoff J. S., Mikos A. G. Biomaterials 2001; 22: 2581–2593
- Ni J., Wang M. Mat Sci Eng C-Bio Sup 2002; 20: 101–109
- Chen L. J., Wang M. Biomaterials 2002; 23: 2631–2639
- Boeree N. R., Dove J., Cooper J. J., Knowles J., Hastings G. W. Biomaterials 1993; 14: 793–796
- Jiang T., Hu P., Liu L., Li Y. Tsinghua Sci Technol 2002; 7: 463–465
- Coskun S., Korkusuz F., Hasirci V. J Biomat Sci-Polym E 2005; 16: 1485–1502
- Galego N., Rozsa C., Sanchez R., Fung J., Vazquez A., Tomas J. S. Polym Test 2000; 19: 485–492
- Shishatskaya E. I., Khlusov I. A., Volova T. G. J Biomat Sci-Polym E 2006; 17: 481–498
- Cool S. M., Kenny B., Wu A., Nurcombe V., Trau M., Cassady A. I., Grondahl L. J Biomed Mater Res A 2007; 82A: 599–610
- Li J., Yun H., Gong Y. D., Zhao N. M., Zhang X. F. J Biomed Mater Res A 2005; 75A: 985–998
- Wang Y. W., Wu Q., Chen G. Q. Biomaterials 2004; 25: 669–675
- Wang Y. W., Wu Q., Chen J. C., Chen G. Q. Biomaterials 2005; 26: 899–904
- Doyle C., Tanner E. T., Bonfield W. Biomaterials 1991; 12: 841–847
- Luklinska Z. B., Bonfield W. J Mater Sci-Mater M 1997; 8: 379–383
- Luklinska Z. B., Schluckwerder H. J Microsco-Oxford 2003; 211: 121–129
- Kose G. T., Korkusuz F., Korkusuz P., Purali N., Ozkul A., Hasirci V. Biomaterials 2003; 24: 4999–5007
- Liu Y., Wang M. Key Eng. Mater. 2007; 334–335: 1217–1220
- Zheng Y. D., Wang Y. J., Yang H. A., Chen X. F., Chen Z. H. J Biomed Mater Res B 2007; 80B: 236–243
- Zheng Y. D., Wang Y. J., Chen X. F., Ren Y. B., Wu G. Chem J Chinese U 2003; 24: 1325–1328
- Al-Salihi, K. A. and Samsudin, A. R. 2004. Med J Malaysia 59(Suppl B): 202–203.
- Shamsuria, O., Fadilah, A. S., Asiah, A. B., Rodiah, M. R., Suzina, A. H. and Samsudin, A. R. 2004. Med J Malaysia 59(Suppl B): 174–175.
- Li H. Y., Chang J. Biomaterials 2004; 25: 5473–5480
- Li H. Y., Zhai W. Y., Chang J. J Mater Sci-Mater M 2008; 19: 67–73
- LeBaron R. G., Athanasiou K. A. Biomaterials 2000; 21(24)2575–2587
- Schulz R. M., Bader A. Eur Biophys J Biophy 2007; 36: 539–568
- Moutos F. T., Freed L. E., Guilak F. Nat Mater 2007; 6: 162–167
- Gong Y. H., He L. J., Li J., Zhou Q. L., Ma Z. W., Gao C. Y., Shen J. C. J Biomed Mater Res B 2007; 82B: 192–204
- Fan H. B., Hu Y. Y., Zhang C. L., Li X. S., Lv R., Qin L., Zhu R. Biomaterials 2006; 27: 4573–4580
- Xin X. J., Hussain M., Mao J. J. Biomaterials 2007; 28: 316–325
- Xia W. Y., et al. J Biomed Mater Res B 2004; 71B: 373–380
- Yamane S., et al. J Biomed Mater Res A 2007; 81A: 586–593, (2007)
- Wang Y. Z., Blasioli D. J., Kim H. J., Kim H. S., Kaplan D. L. Biomaterials 2006; 27: 4434–4442
- Galois L., et al. Biomaterials 2006; 27: 79–90
- Deng Y., Zhao K., Zhang X. F., Hu P., Chen G. Q. Biomaterials 2002; 23: 4049–4056
- Sun J. Y., Wu J., Li H. Y., Chang J. Eur Polym J 2005; 41: 2443–2449
- Zhao K., Deng Y., Chen J. C., Chen G. Q. Biomaterials 2003; 24: 1041–1045
- Deng Y., Lin X. S., Zheng Z., Deng J. G., Chen J. C., Ma H., Chen G. Q. Biomaterials 2003; 24: 4273–4281
- Zheng Z., Bei F. F., Tian H. L., Chen G. Q. Biomaterials 2005; 26: 3537–3548
- Kose G. T., Korkusuz F., Ozkul A., Soysal Y., Ozdemir T., Yildiz C., Hasirci V. Biomaterials 2005; 26: 5187–5197
- Wang Y., Bian Y. Z., Wu Q., Chen G. Q. Biomaterials 2008; 29: 2858–2868
- Pouton C. W. Adv Drug Deliver Rev 2001; 53: 1–3
- Bures P., Huang Y. B., Oral E., Peppas N. A. J Control Release 2001; 72: 25–33
- Foster L. J. R. Appl Microbiol Biot 2007; 75: 1241–1247
- Martin, David P., Skraly, Frank, and Williams, Simon F. 2004. US Patent No. 6828357.
- Ueda H., Tabata Y. Adv Drug Deliver Rev 2003; 55: 501–518
- Marchessault, R. H., Nobes, G. A. R., and Maysinger, D. 2000. US Patent No. 6146665.
- Yano, T., Nomoto, T., Kozaki, S., Imamura, T., Honma, T., and Canon, K. K. 2006. US Patent No. 2006263432.
- Niamsiri, N. , et al 2006. 2006 Bio Micro and Nanosystems Conference IEEE Cat No.06EX1265C: 1-ROM.
- Gursel I., Yagmurlu F., Korkusuz F., Hasirci V. J Microencapsul 2002; 19: 153–164
- Yagmurlu M. F., Korkusuz F., Gursel I., Korkusuz P., Ors U., Hasirci V. J Biomed Mater Res 1999; 46: 494–503
- Turesin F., Gursel I., Hasirci V. J Biomat Sci-Polym E 2001; 12: 195–207
- Gursel I., Korkusuz F., Turesin F., Alaeddinoglu N. G., Hasirci V. Biomaterials 2001; 22: 73–80
- Wang Z. X., et al. J Biosci Bioeng 2003; 96: 537–540
- Wang Z. X., et al. J Biosci Bioeng 2003; 95: 541–543
- Sun J., Dai Z. W., Zhao Y., Chen G. Q. Biomaterials 2007; 28: 3896–3903
- Cheng S., Guo-Qiang C., Leski M., Zou B., Wang Y., Wu Q. Biomaterials 2006; 27: 3758–3765
- Cheng S., Wu Q., Yang F., Xu M., Leski M., Chen G. Q. Biomacromolecules 2005; 6: 593–597
- Gasior M., Rogawski M. A., Hartman A. L. Behav Pharmacol 2006; 17: 431–439
- Martin, D. P., Peoples, O. P., Williams, S. F., and Zhong, L. H. 2002. US Patent No. 6380244.
- Tasaki O., Hiraide A., Shiozaki T., Yamamura H., Ninomiya N., Sugimoto H. Journal of Parenteral and Enteral Nutrition 1999; 23: 321–325
- Tieu, K., Caspersen, C., Jackson-Lewis, V., Neystat, M., Ramasamy, R., and Przedborski, S. 2002. Society for Neuroscience Abstract Viewer and Itinerary Planner 2002: Abstract.
- Kashiwaya, Y., Takeshima, T., Mori, N., Nakashima, K., Clarke, K., and Veech, R. L. 2000. PNAS 97: 5440–5444.
- Reger, M. , et al 2002. Society for Neuroscience Abstract Viewer and Itinerary Planner 2002: Abstract.
- Ma Z. L., Gu X. P., Zeng Y. M., Zhang Y. Ann Clin Lab Sci 2006; 36: 307–311
- Imamura K., Takeshima T., Kashiwaya Y., Nakaso K., Nakashima K. J Neurosci Res 2006; 84(6)1376–1384
- Xiao X. Q., Zhao Y., Chen G. Q. Biomaterials 2007; 28: 3608–3616
- Zhao Y., Zou B., Shi Z. Y., Wu Q., Chen G. Q. Biomaterials 2007; 28: 3063–3073
- Nascimento, J. F. and Pachekoski, W. M., Arnaud, Anotonio M. P. 2008. WO Patent No. 2007090255.
- Schmitz, K., Behrend, D., Sternberg, K., Grabow, N., Martin, D. P., and Williams, S. F. 2007. US Patent No. 2007185561.
- Huja, A., Martin, D., Mccarthy, S. J., Martin, D. P., and Mccarthy, S. 2007. WO Patent No. 2007009090.
- Rizk, S., Martin, D. P., Ho, K., Ganatra, A., Williams, S. F., Schmitz, K., Behrend, D., Sternberg, K., Grabow, N., Coleman, S., and Huja, A. 2007. US Patent No. 2007182041.
- Bae, H., Kang, I., Mun, J., Kim, Y., Bae, H. I Kim, Y. J., Mun, J. Y., and Kang, I. G. 2006. WO Patent No. 2006068421.
- Terenghi, G., Mohanna, P., and Martin, D. P. 2005. WO Patent No. 2005020825.
- Veech, R. L. 2005. US Patent No. 2005058724.
- Terenghi, G., Mohanna, P., and Martin, D. P. 2005. WO Patent No. 2005020825.
- Yano, T., Nomoto, T., Kozaki, S., Imamura, T., Honma, T., Canon, K. K. 2004. WO Patent No. 2004096188.
- Imamura, T., Yano, T., Honma, T., Kozaki, S., Nomoto, T., Tsuchitani, A., Canon, K. K. 2004. WO Patent No. 2004097417.
- Imamura, T., Yano, T., Nomoto, T., Kozaki, S., Honma, T., Tsuchitani, A., Tominaga, A., Homma, T., Kosaki, S. Canon, K. K. 2004. WO Patent No. 2004097416.
- Veech, R. L., King, M. T., Veech, R., and King, M. 2004. WO Patent No. 2004108740.
- Martin, D. P., Rizk, S., Huja, A., Williams, S. F., Martin, D., and Williams, S. 2004. WO Patent No. 2004101002.
- Nomoto, T., Yano, T., Kozaki, S., Honma, T., Homma, T., Kosaki, S., Canon, K. K., Nomoto, T., Yano, T., Kozaki, S., and Honma, T. 2003. EP Patent No. 1277464.
- Yano, T., Nomoto, T., Kozaki, S., Honma, T., Nomoto, K., Canon, K. K., Nomoto, T., Yano, T., Kozaki, S., and Honma, T. 2003. EP Patent No. 1275378.
- Noda, I. 2002. EP Patent No. 1332173.
- Noda, I. 2002. EP Patent No. 1132446.
- Williams, S. F., Martin, D. P., Williams, S., Martin, D., Williams, F., and Martin, P. 2001. WO Patent No. 200119422.
- Williams, S. F. 2001. WO Patent No. 200115671.
- Williams, S. F., Martin, D. P., Williams, F., and Martin, P. 2001. WO Patent No. 200119361.
- Williams, S. F., Martin, D. P., and Skraly, F. 2000. WO Patent No. 200056376.
- Williams, S. F. 2000. WO Patent No. 200051662.
- Marchessault, R. H., Nobes, G. A. R., and Maysinger, D. 2000. CA Patent No. 2247013.
- Veech, R. L. 2000. WO Patent No. 200015216.
- Martin, D. P., Peoples O. P., Williams, S. F., and Zhong, L. 2000. WO Patent No. 200004895.