ABSTRACT
Several studies have been conducted to address the potential adverse health risks attributed to exposure to nanoscale materials. While in vivo studies are fundamental for identifying the relationship between dose and occurrence of adverse effects, in vitro model systems provide important information regarding the mechanism(s) of action at the molecular level. With a special focus on exposure to inhaled (nano)particulate material toxicity assessment, this review provides an overview of the available human respiratory models and exposure systems for in vitro testing, advantages, limitations, and existing investigations using models of different complexity. A brief overview of the human respiratory system, pathway and fate of inhaled (nano)particles is also presented.
Introduction
Nanotechnology is an emerging field that offers insightful and innovative approaches and applications in many areas (De Jong and Borm Citation2008; Kermanizadeh et al. Citation2016; Thiruvengadam, Rajakumar, and Chung Citation2018; Zhao and Castranova Citation2011). According to the recently reviewed European Commission recommended definition, the term “nanomaterial” refers to a “natural, incidental or manufactured material consisting of solid particles that are present, either on their own or as identifiable constituent particles in aggregates or agglomerates, and where 50% or more of these particles in the number-based size distribution fulfill at least one of the following conditions: (a) one or more external dimensions of the particle are in the size range 1 nm to 100 nm; (b) the particle has an elongated shape, such as a rod, fiber or tube, where two external dimensions are smaller than 1 nm and the other dimension is larger than 100 nm; (c) the particle has a plate-like shape, where one external dimension is smaller than 1 nm and the other dimensions are larger than 100 nm.” (European Commission Citation2022). Nanoparticles (NP) production and use are increasing exponentially, making environmental and human exposure to these particles inevitable (Malakar et al. Citation2021). Multiple efforts have been made to understand the health implications from manipulation of and exposure to these nanoscale materials. Inhalation is the main route of entrance for airborne (nano)particles (Oberdorster et al. Citation2015). Based upon clearance mechanisms, it is estimated that solid (nano)particles half-life in the human alveolar region is approximately 700 days, which might constitute a threat to human health (Hagens et al. Citation2007; Oberdörster, Oberdörster, and Oberdörster Citation2005). Growing evidence demonstrated that due to their small size and increased surface area relative to an equal mass of larger diameter particles, inhalation of nano-sized particles might lead to systemic oxidative stress and inflammatory responses that might affect cardiovascular (Alsaleh Citation2021; Kan, Pan, and Castranova Citation2018) and hepatic functions (Kermanizadeh, Powell, and Stone Citation2020; Sun et al. Citation2021). Previously, several investigators demonstrated an association between adverse human health effects following exposure to airborne particulate matter (PM), where ultrafine particles (UFP) seem to play an important role in some responses (Delfino, Sioutas, and Malik Citation2005; Johnson et al. Citation2021; Li et al. Citation2016). For example, it has been widely reported the presence of endotoxins in PM, particularly in coarse and fine particles, that can potentially trigger inflammatory responses, thus exacerbating several respiratory diseases such as asthma (Boraschi et al. Citation2017; Degobbi, Saldiva, and Rogers Citation2011). In addition, despite their distinct origin, UFP and NP share many similarities in terms of their physicochemical properties and modes of action (Stone et al. Citation2017).
Over the past few years, many in vitro and in vivo studies have explored the effects of micro- and nano-sized materials in different lung-related models [reviewed in (Fytianos et al. Citation2016; Kumar, Sharma, and Maitra Citation2017; Nahar et al. Citation2013; Wick et al. Citation2015; Wiemann et al. Citation2016)]. Data generated from these studies characterized multiple hallmarks of (nano)particle-induced pulmonary toxicity, including particle internalization pathways, oxidative stress, genotoxicity, cell cycle alterations, and dysregulation of signaling cascades (Bakand, Hayes, and Dechsakulthorn Citation2012; Li et al. Citation2010; Paur et al. Citation2011; Pietroiusti et al. Citation2018). Several of these mechanisms are associated with the occurrence of negative health outcomes such as impaired lung function and inflammation, vascular dysfunction, and severe acute respiratory and cardiovascular complications (Kobos et al. Citation2020; Sreedharan et al. Citation2022; Stone et al. Citation2017). These adverse effects are strongly linked with different disease states including lung cancer, bronchitis, and acute asthma, cardiac infection and arrhythmia, hypertension, atherosclerosis, and ischemia (Brook et al. Citation2004; Kelly and Fussell Citation2015; Knaapen et al. Citation2004; Kreyling, Semmler-Behnke, and Möller Citation2006; Schulz et al. Citation2005; Shannahan, Kodavanti, and Brown Citation2012).
In vivo studies have been extremely useful for identifying important toxic properties of nanomaterials, screening of nano-delivered drugs or agents with great diversity of physicochemical parameters, as well as for estimation of health-related risks to humans and the environment (Carneiro and Barbosa Citation2016; He Citation2016; Jain and Thareja Citation2019; Sreedharan et al. Citation2022). In vivo investigations are, of course, indispensable to validate and assess the predictivity of the in vitro models (Miller and Poland Citation2020).
Given the increasing urge to find reliable alternatives to in vivo testing, several efforts have been made toward development and/or improvement of more complex and physiologically relevant in vitro systems for inhalation toxicology testing of (nano)particles (Fröhlich Citation2018). Therefore, the main purpose of this review was to provide an overview of the existing pulmonary in vitro models and exposure conditions for identifying and assessing (nano)particle-initiated hazards with determination of associated underlying molecular mechanisms. This review addresses the advantages and limitations of these in vitro models, existing gaps and future directions. As far as we known, this is the first review providing an overview of existing in vitro studies on airborne (nano)particle-induced toxicity in human respiratory system in vitro models of different complexity.
Brief overview of the human respiratory system
The respiratory system is primarily composed of the nose, airways, and parenchyma. The upper respiratory tract allows the passage of air and protects the lower respiratory regions from external injuries (Thomas Citation2013), while gas exchange occurs in the lower respiratory tract such as the alveoli (Weibel, Sapoval, and Filoche Citation2005). Along the respiratory tract, cell types and morphology vary (), which also may be affected in pulmonary diseases (Whitsett and Alenghat Citation2015). As illustrated in , the upper airways are lined with a pseudostratified epithelium that is composed of ciliated, secretory (goblet and club cells), neuro-endocrine, and basal cells, these latter acting as progenitor cells for various cell types of the airway epithelium (Crapo et al. Citation1982; Hiemstra, McCray, and Bals Citation2015). The bronchioles are lined by ciliated cuboidal epithelium, with a small number of non-ciliated club cells that are more dominant in the distal portion (Khan and Lynch Citation2018). In addition, the alveolar epithelium is important for maintaining lung homeostasis and is constituted by cuboidal alveolar epithelial type 1 cells (AEC1) and type 2 cells (AEC2).
Figure 1. Respiratory tract: cell types and morphology. This illustration was created including images obtained from Smart Servier Medical Art (www.smart.servier.com) CC by 3.0.
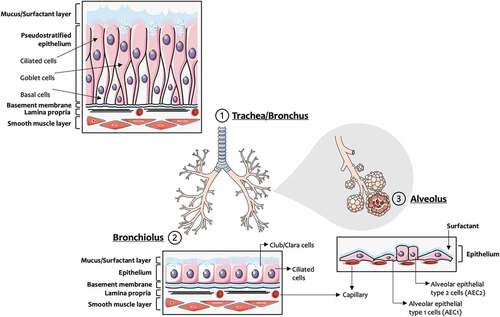
The defense mechanisms of the airways and lung comprise the cough reflex, the epithelial barrier and lining fluid, the mucociliary escalator, metabolic signaling cascades (e.g., activation of cytochrome P450 family and and/or activation of nuclear factor erythroid 2–related factor 2 (Nrf2)-mediated transcription factors), humoral factors including antimicrobial and surfactant peptides or complement proteins and cells that elicit immune responses, namely epithelial cells, macrophages, monocytes, dendritic cells, neutrophils, natural killer cells, and mast cells (Hastedt et al. Citation2016; Rothen-Rutishauser et al. Citation2008). In the large airways, the epithelial lining fluid is composed of a superficial mucus layer overlying a periciliary liquid layer that is responsible for mucociliary clearance through physical unidirectional cilia movement and removal of deposited particles and gases dissolved in the mucus from the respiratory tract (Schuster et al. Citation2013). Further, the alveolar surface is covered by pulmonary surfactant that also plays a critical role in the clearance of inhaled toxicants including aerosolized (nano)particles (Wohlleben et al. Citation2016).
Rodents are the most frequently used animal model in inhalation toxicity studies (Movia, Bruni-Favier, and Prina-Mello Citation2020). However, as presented in , considerable differences in the anatomy and physiology of the respiratory system between rodents and humans exist, that need to be considered when designing and interpreting inhalation (nano)toxicity studies. Indeed, the distinct respiratory tract architecture between rodents and humans affects the airflow pattern and ventilation rates, which in turn influence deposition, clearance, and retention of inhaled particles (Bakand, Hayes, and Dechsakulthorn Citation2012; Clippinger et al. Citation2018). Differences in the type and number of cells lining the airways, as well as in mucociliary clearance also exist. Taken together, these aspects markedly influence (nano)particle biological fate and hazard potential.
Table 1. Main anatomical and physiological differences between humans’ and rodents’ respiratory system. [Adapted from Harkema et al. (Citation2012); Meyerholz et al. (Citation2018); Rackley and Stripp (Citation2012)].
Pathways and fate of inhaled particles
As depicted in , once a particle is inhaled, it enters the oropharyngeal region where the particle meets the trachea, being conducted to the bronchi and alveolar region. Aerosol particles are often described as spherical and monodisperse; however, particle collisions often originate non-spherical aggregates and/or agglomerates (Kleinstreuer and Zhang Citation2010). Deposition of inhaled particles is dependent upon (1) aerosol physicochemical properties (Morawska and Buonanno Citation2021) (2) anatomical (diameter, length, and branching angle of airway segments) and (3) physiological (airflow and breathing pattern) factors (Andujar et al. Citation2011; Clippinger et al. Citation2018). Physicochemical parameters governing particle deposition include particle size/and or size distribution, surface area, surface reactivity, density, shape, hygroscopicity/hydrophobicity, and chemical reactions of the particle (Morawska and Buonanno Citation2021). In addition, the composition, viscosity and surface tension of lung surfactant influence markedly particle trajectory, deposition, and biological fate. Anatomical features, such as surface roughness, airway morphology, cross-sectional profiles, and branching angles exert a significant influence on the deposition of (nano)particles in humans. This needs to be considered for the lung burden estimation of airborne particles, particularly when comparing healthy vs vulnerable individuals such as with lung disease (Jakobsson et al. Citation2018).
Figure 2. Inhaled particles: deposition mechanisms along the respiratory tract. This illustration was created including images obtained from SlidesCarnival (https://www.slidescarnival.com) CC by 4.0.
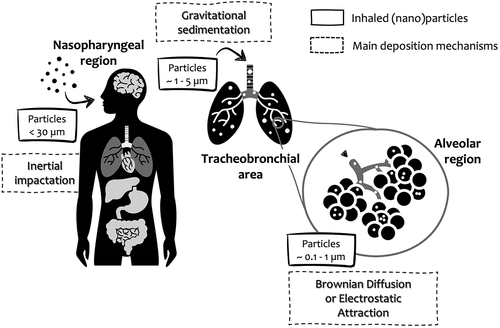
Overall, the main mechanisms for particle deposition include: interception (particle-surface contact), inertial impaction (particle sudden change in the direction of the flow), gravitational sedimentation (settling of particles under the action of gravity), diffusion (random motions of the particles, e.g. Brownian motion, where randomized particle motion is initiated by their collision with gas molecules), and electrostatic precipitation (particle charge may potentially affect their deposition in the airways) (Bui et al. Citation2020; Darquenne Citation2020; Tsuda, Henry, and Butler Citation2013). As illustrated in , larger particles (5–30 μm) are deposited in the nasopharyngeal region by inertial impaction, while smaller particles (1–5 μm) are deposited in the tracheobronchial area by gravitational sedimentation where they may or may not be removed by mucociliary clearance. On the other hand, nano-sized particles (0.1–1 μm) penetrate deeper into the alveolar region, such as the alveoli where airflow is low, deposited by Brownian diffusion or electrostatic attraction (Bakand, Hayes, and Dechsakulthorn Citation2012; Hagens et al. Citation2007).
So far, it is not clearly understood how (nano)particles interact with complex biological environments to influence phagocytic recognition and clearance (indirectly via necrosis or apoptosis), cellular processing and, consequently, toxicological outcomes (Gustafson et al. Citation2015). Physicochemical properties of aerosol particles significantly influence their deposition but also their clearance from human airways (Bierkandt et al. Citation2018; Braakhuis et al. Citation2014). Individual (nano)particles might be too small to be efficiently recognized and phagocytosed by alveolar macrophages. By avoiding the normal phagocytic defenses of the respiratory system, (nano)particles may gain access to the systemic circulation and reach different extrapulmonary sites (Nemmar et al. Citation2013). Soluble particles are more readily eliminated compared to insoluble particle components (Braakhuis, Oomen, and Cassee Citation2016). In contrast, charged (nano)particles seem to be retained to a greater extent in the human respiratory airways due to their ability to attract different proteins (Docter et al. Citation2015). Gustafson et al. (Citation2015) postulated that when a (nano)particle incomplete/arrested phagocytosis occurs as during long-term residence of inhaled (nano)particle within phagocytic cells, the human body, rather than eliminating these particles, developed a mechanism that induces a local response to isolate these foreign (nano)materials from the host biological environments, which might potentially trigger adverse chronic inflammatory or immunological responses.
Another important aspect to take into consideration is how physiological fluids might change the physicochemical properties and behavior of (nano)particles (Urban et al. Citation2016). Upon contact with the biological pulmonary milieu, (nano)particles may become surrounded by biomolecules such as albumin and proteins in the surfactant, which significantly contribute to the formation of a corona around them and change particle size and kinetics in the airways (Monopoli et al. Citation2012). In the alveolar region, (nano)particles interact with the lipids present in the surfactant film located at the air-liquid interface (ALI) in the epithelial lining fluid covering the internal surface of the lung (Raesch et al. Citation2015). The surfactant helps to stabilize the alveoli and promotes clearance of inhaled particles to maintain alveoli in a sterile- and inflammation-free environment (Kendall and Holgate Citation2012). Accordingly, characterization of nano-sized materials in relevant pulmonary biological fluids is of utmost importance (Wohlleben et al. Citation2016).
There are several in vitro and in vivo studies that already identified the major biological mechanisms involved in pulmonary toxicity induced by (nano)particles, including: ineffective particle clearance, intracellular uptake/internalization, impairment of lung macrophage-mediated phagocytosis, loss of plasma membrane integrity, and mitochondrial dysfunction, oxidative stress (ROS generation, glutathione depletion, and lipid peroxidation), cytokine production and activation of inflammatory signaling cascades, DNA and chromosomal damage, altered DNA methylation and repair, and altered cell cycle regulation (Bakand and Hayes Citation2016; Ianni et al. Citation2022; Paur et al. Citation2011; Pietroiusti et al. Citation2018; Stone et al. Citation2017).
In vitro models for (nano)particle human respiratory toxicity assessment
For many years, in vitro culture systems failed to represent the complexity of multicellular organisms. Recently, more complex and physiologically relevant human in vitro models emerged and gained some acceptance (Bassi et al. Citation2021; Sakolish et al. Citation2016). The study of the biological effects of inhaled (nano)particles is particularly challenging and in vitro respiratory models have been used as an important tool for assessing underlying molecular mechanisms and cellular responses. At the same time, cell culture and exposure conditions need to be as close as possible to the ones found in vivo. Several human in vitro models exist to represent specific areas of the respiratory tract such as nasal, tracheal, bronchial, and alveolar regions (Fröhlich and Salar-Behzadi Citation2014; Steimer, Haltner, and Lehr Citation2005). In order to accurately predict the effects of inhaled (nano)particles, an in vitro system needs to include the following features: (1) a suitable model mimicking the morphology and metabolic function of a particular healthy or vulnerable tissue/organ, and able to reproduce in vivo particle deposition patterns and clearance; (2) an exposure chamber for culture exposure to aerosolized (nano)particles; (3) a set of relevant biomarkers that enable high-throughput evaluation of key toxicity events. While concerns regarding the resemblance of cells cultured in vitro to those found in the human lung may be raised, information available from molecular cell atlas, a computer-assisted qualitative data analysis software, may be performed, either by single-cell RNA sequencing (Travaglini et al. Citation2020) or multi-omics atlas analysis (Madissoon et al. Citation2021) to compare freshly isolated human lung cells with in vitro cell cultures to display a reliable level of resemblance. An overview of the key features of the available pulmonary in vitro models, namely pulmonary cell lines, primary cultures, organoids/spheroids, three dimensional (3D) cultures and lung-on-chip devices () is provided below.
Figure 3. Schematic representation of the in vitro models addressed in the present review: (a) Mono- and co-cultures of respiratory cells under submerged and (b) air-liquid interface culture conditions; (c) Spheroid and organoids; (d) 3D cultures and (e) Microfabricated human breathing-inspired lung-on-a-chip microfluidic device [Adapted from Huh et al. (Citation2010)]. The device simulates physiological breathing movements by applying a vacuum into the side chambers causing a stretch of the membrane. This illustration was created including images obtained from Smart Servier Medical Art (www.smart.servier.com) CC by 3.0. The relative costs of each cell system are indicated using a 1 to 4 level ($-).
![Figure 3. Schematic representation of the in vitro models addressed in the present review: (a) Mono- and co-cultures of respiratory cells under submerged and (b) air-liquid interface culture conditions; (c) Spheroid and organoids; (d) 3D cultures and (e) Microfabricated human breathing-inspired lung-on-a-chip microfluidic device [Adapted from Huh et al. (Citation2010)]. The device simulates physiological breathing movements by applying a vacuum into the side chambers causing a stretch of the membrane. This illustration was created including images obtained from Smart Servier Medical Art (www.smart.servier.com) CC by 3.0. The relative costs of each cell system are indicated using a 1 to 4 level ($-).](/cms/asset/67b6646e-e049-4479-9da4-7a2ac8bd8c03/uteb_a_2166638_f0003_oc.jpg)
Pulmonary cell lines: mono- and cocultures
Primary human lung epithelial cells are, in theory, the ideal choice for (nano)particles' testing, due to their resemblance in morphology, organization, stratification, and physiological function to the human airway epithelium (Dvorak et al. Citation2011; Pezzulo et al. Citation2011; Rosario et al. Citation2018). In addition, primary cultures may be established from tissues of healthy or lung disease donors offering the possibility of evaluating in vitro the susceptibilities in health and disease (Faber and McCullough Citation2018). However, primary cultures have some drawbacks as follows: 1) these are difficult to establish and maintain in culture, (2) require complex and expensive cell culture media, (3) are poorly reproducible, and (4) have a limited life span. This is why continuous cell lines have been widely used for in vitro nanotoxicology studies (Katt et al. Citation2016). There are numerous in vitro lung cell lines that can be used to study the cellular interplay and cellular responses following (nano)particle exposure. Notwithstanding, since epithelial cells play a central role in the respiratory tract, providing a physical barrier to inhaled (nano)particles, these are commonly used in vitro models for assessing (nano)toxicity. In accordance, presents the most popular epithelial cell lines for in vitro toxicological assessment of inhaled particles (Bierkandt et al. Citation2018; Fröhlich Citation2018; Hiemstra et al. Citation2018; Jia, Wang, and Liu Citation2017; Rothen-Rutishauser et al. Citation2008; Schlinkert et al. Citation2015). Despite all advantages, some tumoral or immortalized epithelial cell lines including bronchial 16HBE14o, BEAS-2B, and Calu-3 cells, lack human in vivo characteristics such as (1) mucociliary differentiation, (2) formation of an effective barrier, and (3) reduced metabolic capacity (Faber and McCullough Citation2018). However, under appropriate growth conditions, some of these cell lines may exhibit tight junctions, cilia and mucus production providing an effective barrier between the external and internal environments. Previously Gonzalez-Mariscal et al. (Citation1990) reported the importance of calcium for the development of tight junctions between epithelial cells.
Table 2. Human epithelial cell lines commonly used in in vitro (nano)particle pulmonary toxicity studies.
George et al. (Citation2015) comparatively assessed the translocation of fluorescent 50 nm silica (SiO2) NP in three in vitro human lung models, in bronchial epithelial NCI-H292 and Calu-3 cells, and in alveolar epithelial A549 cells grown onto Transwell® inserts. George et al. (Citation2015) found that bronchial epithelial Calu-3 cells would be the most relevant model for assessing NP uptake since, amongst all the studied cells, these were able to form tight junctions. Inflammatory or immune responses are also difficult to mimic in such simplistic models since these processes involve a highly coordinated network of many cell types (Joris et al. Citation2013; Savolainen et al. Citation2010). Some of these limitations might be overcome by using coculture models constituted by two or more cell types with some degree of contact between them, which may potentially improve culture success, by improving intracellular interactions and communication between different cell types as well as cellular morphology and integrity by often displaying tight and adherent junctions (Goers, Freemont, and Polizzi Citation2014; Kasper et al. Citation2011; Snyder-Talkington et al. Citation2012; Vis, Ito, and Hofmann Citation2020). Therefore, cocultures are versatile models that more closely mimic human in vivo environments (Edmondson et al. Citation2014), and are preferred models to understand (nano)particle mechanistic behavior in more complex biological systems (Costa et al. Citation2013). Notwithstanding, cocultures present many challenges. The selection of the optimal culture conditions for cocultures may be demanding, for instance if each cell type requires a specific culture medium composition. Coculture medium optimization might be a laborious and time-consuming task due to the numerous possible combinations and cellular implications (Goers, Freemont, and Polizzi Citation2014; Vis, Ito, and Hofmann Citation2020). Another important feature is the cell population ratio that needs to be optimized to achieve a stable culture such that one cell type does not negatively affect the others, and to more closely resemble the in vivo situation (Vis, Ito, and Hofmann Citation2020).
Coculture models have been developed to resemble the complexity of the human airways by combining airway epithelial cells together with fibroblasts, endothelial cells, airway smooth muscle cells, as well as immune cells including macrophages, dendritic, and mast cells. These models contribute to a better understanding of the toxicology and translocation mechanisms of (nano)particles (Bierkandt et al. Citation2018; Braakhuis et al. Citation2015). summarizes some studies that assessed the effects of (nano)particle exposure in human cocultures of different regions of the lung barrier. One of the most studied regions is the bronchial epithelium since it plays a critical role in biological stress responses to inhaled (nano)particles (Jia, Wang, and Liu Citation2017). It is noteworthy that nano-sized particle deposition occurs in the respiratory tract primarily in the alveolar region (Londahl et al. Citation2014). Therefore, alveolar epithelial cells are relevant models for the toxicity of inhalable (nano)particles, particularly in the assessment of particle retention and translocation through these cells (Leibrock et al. Citation2019). For this purpose, in vitro models representative of the alveolar-capillary and/or air-blood barrier are also explored in (nano)toxicity assessments. The air-blood barrier is predominantly composed of alveolar epithelial cells and macrophages, that function as a structural and immunological barrier to environmental aggressors, such as fine and nano-sized particles (Kletting et al. Citation2018). For the alveolar region, A549 is the most frequently used cell line for assessment of (nano)particle toxicity. These cells are often used either as monocultures or in cocultures with other cell lines such as immune cells or macrophages (Fröhlich Citation2018). Alveolar macrophages, in turn, are important regulators of the inflammatory processes in the lung and ingest nano-sized particles as a clearance defense mechanism (Geiser Citation2010). THP-1 cells are a commonly used cell line for investigating in vitro function and regulation of monocytes and macrophages.
Table 3. Pulmonary (nano)particle toxicity studies using coculture models mimicking relevant human lung barriers.
Increasing evidence supports the higher relevance of cocultures compared to monocultures (). Kasper et al. (Citation2011) investigated the cytotoxic and inflammatory responses of monodisperse amorphous 30 nm SiO2 NP in conventional monocultures vs coculture models representative of the alveolar-capillary barrier. Although lower cytotoxic effects in terms of cell viability, membrane integrity, and Trans-Epithelial Electrical Resistance (TEER) were observed in cocultures compared to monocultures, significantly increased levels of inflammatory mediators IL-6 and IL-8 and apoptotic markers including phosphorylation of p53-protein at Ser15, Ser46 and Ser392 were found. Kasper et al. (Citation2011) postulated that these cocultures were more representative of the alveolar regions of the human lung compared to the conventional monocultures since these exhibited the usual early inflammatory responses that occur in pulmonary alveoli. In addition, Wang et al. (Citation2020) assessed the toxicity of aerosolized citrate-capped gold (Au), 15% silver on silica (Ag-SiO2) and copper oxide (CuO) NP on triple-cocultures of human THP-1 differentiated macrophages, alveolar epithelial A549 and endothelial EA.hy 926 cells, cultured under ALI conditions (4 hr; 3.5 mg/m3). Wang et al. (Citation2020) compared NP effects in the triple cocultures vs monocultures of A549, EA.hy 926, and THP-1 cells. Data demonstrated that NP induced greater oxidative stress (15% Ag-SiO2) and higher IL-8 levels (15% Ag-SiO2, CuO) in coculture than in A549 monocultures, whereas a similar response in terms of reactive oxygen species (ROS) and IL-8 responses were noted following CuO NP exposure between triple cocultures and EA.hy 926 endothelial cells (Wang et al. Citation2020). Further, Loret et al. (Citation2016) also compared the effect of poorly soluble cerium oxide (CeO2) and titanium oxide (TiO2) NP in monocultures vs cocultures of A549 and THP-1 cells, and found that cocultures were not only more sensitive than monocultures but also more predictive of in vivo pulmonary toxicity attributed to CeO2 and TiO2 NP in the rat. For each dose metric used (mass/alveolar surface or mass/macrophage), A549 and THP-1 cocultures under ALI conditions exhibited similar levels of pro-inflammatory responses, cytotoxicity and oxidative stress to those observed in rat bronchoalveolar lavage fluid (BALF) supernatants and cells (Loret et al. Citation2018). Similarly, Camassa et al. (Citation2022) compared nanomaterial300K-induced cyto- and genotoxicity in monocultures of human epithelial A549 cells to co-cultures of human endothelial EA.hy926 and differentiated dTHP-1 cells under ALI conditions. Nanomaterial-300K induced cytotoxicity in the A549 monocultures and DNA strand breaks and oxidized base lesions in the coculture with EA.hy926. By further adding the immune cells , an increase in the complexity of the system was accompanied by changes in the sensitivity of the triculture to NM-300K. Camassa et al. (Citation2022) concluded that utilization of more complex 3D models altered not only the sensitivity but the outcome of the toxicity testing, an aspect that needs to be considered when elucidating human hazard assessment.
As the number of cell types in the coculture increases, more predictive the model seems to be. In this regard, Wang et al. (Citation2019) assessed the toxicity mechanisms of urban ambient PM <2.5 µm (PM2.5) in three in vitro model approaches of increasing complexity: (1) monocultures of A549 alveolar epithelial cells, THP-1 differentiated macrophages, and EA.hy926 endothelial cells; (2) bicultures of A549 cells and EA.hy926 endothelial cells seeded on the apical side of Transwell® inserts and on the basolateral chamber, respectively; (3) triple cocultures of A549 and THP-1 cells seeded on the apical side of Transwell® inserts, and EA.hy926 endothelial cells seeded on the basolateral chamber. These investigators showed that apical exposure for 24 hr of the cocultures induced a greater inflammatory response compared to A549 monocultures. Intercellular Adhesion Molecule 1 (ICAM-1) and caveolin-1 mRNA expression levels in EA.hy926 cells, an endothelial function marker, were significantly higher in the tricultures than in monocultured cells, while upregulation of caveolin-1 was detected in bicultures but only at high concentrations. In addition, PM2.5 crossed the epithelial barrier and deposited in the endothelium. Taken together these data suggest that triple cocultures constitute a reliable model to assess the impact of these ambient particles on cytotoxicity and adverse effects on cardiopulmonary system (Ferraz et al. Citation2016; Wang et al. Citation2019).
Fizesan et al. (Citation2019) used a human tetraculture alveolar barrier model consisting of alveolar epithelial A549, THP-1 macrophages and HMC-1 mast (in the apical compartment), and EA.hy926 endothelial (in the basolateral compartment) cells cultured at ALI to evaluate the influence of exposure to aerosolized Ag NM (two spherical shape particles and one PVP-coated Ag nanowire) utilizing a VitroCell® Cloud exposure system. Fizesan et al. (Citation2019) noted the capacity of this tetraculture model to secrete both anti- and pro-inflammatory cytokines (IL-12p70, IL-13, IL-1β, IL-2, IL-4, and IL-8) following exposure to Ag NM, reflecting complex biological responses that naturally occur in native respiratory epithelia. It is worthwhile noting that human alveolar epithelial lentivirus immortalized (hAELVi) cells combined with THP-1 alveolar macrophages (Kletting et al. Citation2018) or human airway epithelial cells (huAEC) (Leibrock et al. Citation2019) were found to form an effective barrier under ALI conditions, constituting promising models to study the effect of inhalable NP ().
Advanced respiratory tissue cultures
For the assessment of (nano)particle toxicity in the respiratory tract, advanced 3D in vitro tissue models have been emerging as promising systems over traditional two-dimensional (2D) cultures. These models contain different cell types in varied orientation and number that need to be organized in a structure that reflects the tissue of interest. These cultures are often obtained from donor-derived primary cells or from stem cells such as Induced Pluripotent Stem Cell (iPSC)), and are commonly grown in tissue-specific scaffolds (Carvalho et al. Citation2020; Kastlmeier et al. Citation2022; Langhans Citation2018). These models either mimic normal or diseased tissues (Jackson and Lu Citation2016; Sotty et al. Citation2019). Advanced multicellular 3D lung tissue models better reflect cellular interactions observed in vivo and, therefore, enable investigation of the cellular interplay between different cell types following (nano)particle inhalation exposure. Some of these respiratory models display active ciliary beating and mucus production that mimic mucociliary clearance defense systems (George et al. Citation2019; Kooter et al. Citation2017).
Despite the major advantages of 3D models, these fail to reproduce the mechanical stretch and deformation that occurs in lung tissues during breathing (Nossa et al. Citation2021). This mechanical stimulus strongly influences biological functions and signaling of lung cells, which consequently impacts biological responses elicited by (nano)particles. Therefore, future studies need to address these major gaps in development of advanced respiratory tissue cultures, such that this crucial aspect needs to be taken into consideration in airborne (nano)particle toxicity assessment.
In are depicted currently available human respiratory tissue 3D models that include MucilAir™ and SmallAir™ from Epithelix Sàrl, EpiAirway™ and EpiAlveolar™ from MatTek Corporation, and Micro-Lung™ and Metabo-Lung™ from Cardiff University. Commercially available 3D models provided by Epithelix Sàrl and MatTek Corporation are established from nasal, tracheal, bronchial or small airways biopsies of individuals, either healthy or with respiratory pathologies such as COPD, asthma and cystic fibrosis. These models are highly differentiated mucus-producing and ciliated cultures, with well-developed tight junctions, effective epithelial resistance and metabolic activity, that are able to maintain their characteristics over long periods of time (up to several months), which is a major advantage over other cellular models. Recently, human-derived tracheal/bronchial epithelial cultures are also available as a co-culture system with normal human fibroblasts.
Table 4. Advanced 3D in vitro models of human respiratory tissues.
In contrast, Micro-Lung™ and Metabo-Lung™ from Cardiff University are not commercially available. The Micro-LungTM model was established from normal human bronchial epithelial (NHBE) cells isolated from surgical patients and postmortem donors (Prytherch and Bérubé Citation2015), while the Metabo-Lung™ consists of NHBE cells cocultured with human primary hepatocytes, offering the possibility of assessing the role of metabolism (Prytherch et al. Citation2011). To the best of our knowledge, there are no published studies available using these models to evaluate (nano)particle-mediated toxicity. Recently, OncoCilAir™ has appeared, a 3D lung cancer model that combines a functional reconstituted human airway epithelium, human lung fibroblasts, and lung adenocarcinoma cells that may be kept in culture for several months (Benainous et al. Citation2018), enabling concurrent testing of drug efficacy, side-toxicity and tumor recurrence. Nevertheless, despite all the efforts in development of advanced and robust 3D in vitro cell cultures, as reliable alternatives tools to animal testing, thus far none had been approved or validated for pulmonary toxicity testing of airborne chemical, (nano)particle or NM exposure (Pfuhler et al. Citation2020).
presents an overview of existing in vitro studies on the pulmonary toxicity of (nano)particles using advanced 3D lung models. The majority of investigations performed so far were conducted utilizing MucilAir™ human bronchial epithelial cultures. In this regard, Frieke Kuper et al. (Citation2015) comparatively investigated the toxicity of liquid suspensions of aggregated CeO2 NP in MucilAir™ bronchial cultures, bronchial epithelial BEAS-2B and alveolar epithelial A549 cells. While no signs of marked toxicity were observed in the MucilAir™ cultures, most likely due to the presence of the mucociliary apparatus that prevented NP from reaching the cultures, significant concentration-dependent genotoxicity on BEAS-2B and A549 cells after 24 hr exposure to CeO2 NP (33–333 µg/cm2) was detected. Di Cristo et al. (20200 also reported no apparent signs of morphological alterations or cytotoxic effects following repeated exposure of MucilAir™ cultures for 12 weeks, 5 days per week to aerosolized SiO2 NP (0.90 to 55 µg/cm2), which was also attributed to the mucociliary clearance.
Table 5. Overview of the existing pulmonary (nano)particle toxicity studies using advanced 3D in vitro models.
As indicated previously, MucilAir™ cultures might also be established from diseased tissues, which offers the possibility to investigate the effects of (nano)particles in primary cultures from individuals with respiratory diseases. In this context, Chortarea et al. (Citation2017) evaluated the pulmonary toxicity of occupationally relevant doses (10 μg/cm2 for 5 weeks/5 days per week) aerosolized multiwalled carbon nanotubes (MWCNT) in MucilAir™ bronchial cultures from healthy and asthmatic donors. Although no cytotoxicity or morphological changes were observed, chronic MWCNT exposure induced a pro-inflammatory and oxidative stress response in both types of cultures, accompanied by elevated cilia beating frequency and alterations in mucociliary clearance. However, the magnitude and duration of the observed effects were higher in the asthmatic compared to healthy cells, suggesting that individuals with asthma may be more susceptible to adverse effects from chronic MWCNT exposure (Chortarea et al. Citation2017). Donor variability is an important aspect that needs to be considered when designing and interpreting data of primary culture-based studies. In this regard, Kooter et al. (Citation2017) examined the toxicity of aerosolized CuO NP bronchial airway MucilAir™ cultures obtained from 4 donors. Despite no major cytotoxicity occurrence, an increase in levels of IL-6 and Monocyte Chemoattractant Protein (MCP)-1 release after 24 hr exposure was found. Dankers et al. (Citation2018) also investigated the pro-inflammatory potential of 6 metal oxide NPs (CeO2, Mn2O3, CuO, ZnO, Co3O4, and WO3; 27–108 μg/mL) in MucilAir™ cultures and dendritic cells (DC). In MucilAir™ cultures, higher secretion of IL‐6, IL‐8, and MCP-1 pro-inflammatory cytokines was found after 24 hr incubation with CuO NP droplets, while only exposure for 48 hr to Mn2O3 NP upregulated all examined DC maturation biomarkers (HLA‐DR, CD80, CD83, and CD86). Interestingly, Dankers et al. (Citation2018) addressed the potential interaction between epithelial cells and DC by exposing the latter to MucilAir™-exposed cultures media, and found that only Mn2O3 NP triggered DC maturation, suggesting the process was not dependent upon epithelial cells stimulation (Dankers et al. Citation2018).
These models were employed to assess the toxicity of occupationally relevant NP. In this regard, George et al. (Citation2019) evaluated the toxicity of thermonuclear fusion released-like milled tungsten (W) NP, tungstate (WO42-) and tungsten carbide cobalt particles alloy (WC-Co), in MucilAir™ cultures treated for 24 hr with particle liquid suspensions, whose effects were monitored up to 28 days after exposure. These NP exerted a minor impact in MucilAir™ bronchial cultures since no induce significant alterations were noted in metabolic activity and viability; however, a decrease in barrier integrity and a transient rise of IL-8 levels at 24 and 96 hr was detected after initial exposure (George et al. Citation2019). Recently, Bessa et al. (Citation2021) also assessed the toxicity of two engineered NP [ENP; antimony-tin oxide (ATO) and zirconium oxide (ZrO2) NP] used as input materials in advanced ceramics, and two fractions [fine <2.5 µm (PGFP) and nano-sized <0.2 µm (PGNP)] of airborne particles released during thermal spraying of ceramic coatings onto metal surfaces, in MucilAir™ cells under ALI conditions. These cultures were exposed for three consecutive days to aerosolized particles, and cyto-, genotoxicity, and pro-inflammatory responses were assessed. The obtained results showed that PGFP and PGNP exhibited greater toxicity than ENP in mass per area unit, although the presence of mucociliary apparatus in the advanced 3D in vitro bronchial cultures seemed to markedly attenuate the adverse effects (Bessa et al. Citation2021). Pavlovska et al. (Citation2021) determined the biological effects of airborne particulates released from woodworking and metalworking industrial processes in 3D EpiAirwayTM cultures and A549 lung epithelial cells. Data demonstrated that exposure to these ambient pollutant particles exerted minor acute effects on the morphology and viability of A549 cells and EpiAirwayTM tissues. However, a marked reduction in tissue viability after 8 hr exposure to woodworking particles, as well as a non-significant reduction after 72 hr incubation with metalworking particles were reported. In addition, suppressed expression of gelsolin (more pronounced for metalworking particles), caspase-3 and IL-6 (metalworking particles) was found (Pavlovska et al. Citation2021).
Few studies exist on the effects of (nano)particle exposure in human small airway models. Based upon previous in vivo findings that showed Ag NP deposition in the small airway epithelium following inhalation (Seiffert et al. Citation2016), Guo et al. (Citation2018) investigated the effects of the same Ag NP in organotypic reconstituted 3D primary human small airway epithelial cell cultures (SmallAir™) under ALI conditions using the same in vivo doses. Data obtained showed DNA damage, cell cycle changes, and oxidative stress in response to exposure to the aerosolized Ag NP (up to 13.2 ng/cm2). A significant correlation between in vivo–in vitro transcriptional changes in immune-related genes, that were of similar magnitude in response to Ag in the ionic (Ag+) or in the nanoform was detected (Guo et al. Citation2018). More recently, Barosova et al. (Citation2020) evaluated the effects of repeated exposure over 3 weeks to occupational doses (1–30 μg/cm2) of two MWCNT- Mitsui-7 and Nanocyl- aerosols, in EpiAlveolar™ cultures constituted by human alveolar epithelial cells, pulmonary endothelial cells and fibroblasts. In parallel, EpiAlveolar™ cultures were cocultured with human monocyte-derived macrophages (MDM) to determine their potential role in the pro-inflammatory and profibrotic responses. Barosova et al. (Citation2020) found that Nanocyl induced less pronounced toxicity than Mitsui-7 in EpiAlveolar™ cultures, whereas EpiAlveolar™ + MDM cocultures exhibited pro-inflammatory responses at later time points compared to EpiAlveolar™ cultures.
Lung spheroids/organoids
Spheroids and organoids are 3D structures composed of multiple cells that cluster together into self-organized aggregates. Both terms are often used interchangeably in the literature, though some differences exist between the two. Spheroids are simple spherical and scaffold-free cellular models that are typically obtained from mature single-cell suspensions (Zanoni et al. Citation2020). On the other hand, organoids are complex clusters derived from organ-specific cells that self-assemble within a scaffold such as gels made of a complex mixture of different extracellular matrix (ECM) proteins, including laminin, fibronectin, collagen, and heparin sulfate (e.g., Matrigel), though not all organoids are formed within an ECM (Gkatzis et al. Citation2018). Organoids may be generated from adult or embryonic stem cells (Hofer and Lutolf Citation2021). One curious aspect of these particular 3D structures is that they can be maintained for prolonged periods of time without karyotype changes (Kar et al. Citation2021). In the respiratory field, organoids are valuable models to mimic the complex environment of the respiratory mucosa and the relationship between different cell types. Indeed, human lung organoids have been successfully established from cells of different origins such as epithelial progenitor cells derived from embryonic (Miller et al. Citation2018) or adult lung (Tan et al. Citation2017; Zacharias et al. Citation2018), and from human pluripotent stem cells (hPSC) (Chen et al. Citation2017; Gotoh et al. Citation2014; Yamamoto et al. Citation2017). Previously, establishment of alveolar organoids from cocultures of epithelial progenitor cells with mesenchymal or endothelial cells or from cocultures of mesenchymal cells with fetal lung tissue or hPSC were reported (Wilkinson et al. Citation2016).
While lung spheroids/organoids may be reliable models to investigate pulmonary injury and repair mechanisms, their 3D spherical nature and matrix components pose technical challenges for application under ALI conditions (Hiemstra et al. Citation2018). Another limitation is that lung movements during gas exchange are difficult to simulate in lung spheroid/organoid models (Li et al. Citation2020). However, human lung spheroids or organoids have still been used in the fields of regenerative medicine, lung disease modeling, and drug efficacy testing (Archer, Bobet-Erny, and Gomes Citation2021; Cores et al. Citation2020; Tan et al. Citation2017).
Organotypic lung cultures are also valuable models for toxicological studies, namely for nanotoxicity assessment (Prasad et al. Citation2021). However, few studies have been conducted for NP testing in human-derived lung spheroids/organoids. In this regard, Sambale et al. (Citation2015) comparatively investigated the effects of ZnO and TiO2 NP using human alveolar epithelial A549 cells grown in traditional 2D monolayer cultures or in 3D spheroids. Overall, A549 cells displayed lower resistance to ZnO NP-induced toxicity (up to 100 µg/ml) in the form of spheroids compared to 2D monolayers, while exposure to TiO2 NP (up to 150 µg/ml) was nontoxic to 2D cultures but produced a decrease in A549 spheroids viability and affected spheroid formation with several smaller spheroids being formed instead of a single larger spheroid (Sambale et al. Citation2015). Kabadi et al. (Citation2019) investigated the influence of different types of carbon-based materials (MWCNT, M120 carbon black NP, or crocidolite asbestos fibers; 0.5–10 μg/ml) in scaffold-free 3D spheroids established from human lung IMR-90 fibroblasts, bronchial epithelial BEAS-2B and monocytic THP-1 cells. MWCNT were the most cytotoxic to the spheroid-like triple cocultures by day 7, producing more than 40% decrease in cell viability at the highest tested concentration. Further, gene expression analysis carried out following 4 or 7 day exposure to tested materials revealed significant upregulation of ECM components (collagens and decorin), cytokines IL-1β and IL-6, growth factors, and matrix metalloproteases (MMP) related genes (Kabadi et al. Citation2019). Recently, Liu et al. (Citation2021) assessed the toxicity of nano-carbon black and nano-SiO2 utilizing alveolar type 2 epithelial cell-like cells (ATL), differentiated from hPSC cells, grown in 2D monolayers or in 3D organoids assembled in Matrigel scaffolds. The toxicity of the nano-carbon black was determined in ATL 2D monolayers, while nano-SiO2 effects were assessed in ATL 3D organoids. Nano-carbon black (1–100 ng/ml) did not induce oxidative stress in ATL 2D cultures or alter cell viability after 6 hr or 7 day treatment, respectively. As for nano-SiO2 (1–100 ng/ml), a significant elevation in ROS generation was observed in ATL 3D organoids at 6 hr after exposure, although organoid viability and gene expression of the investigated markers at 14 days after exposure were not markedly affected (Liu et al. Citation2021).
Lung-on-a-chip
Mechanically active microdevices, named organ-on-a-chip, combining the capability of cell culture models with microfluidics were developed to (1) reconstitute tissue-tissue interfaces crucial to resemble organ functions, and (2) are gaining relevance for drug screening and toxicology applications. The ideal lung-on-a-chip needs to encompass a micro engineered cell culture device capable of replicating the human lung 3D architecture, environment, breathing movement, and physiology. The first lung-on-a-chip was reported by Huh et al. (Citation2010), who developed a biomimetic microsystem replicating key structural, functional, and mechanical properties of the human alveolar-capillary interface. This model consisted of a microfluidic system with two microchannels separated by a thin, flexible and porous membrane coated with fibronectin or collagen to resemble the ECM, and human alveolar epithelial and pulmonary microvascular endothelial cells cultured on opposite sides of the membrane (). This biologically inspired human breathing lung-on-a-chip microdevice has been successfully used to evaluate SiO2 NP transport across this in vitro alveolar-capillary barrier, intracellular ROS production, and inflammatory responses (Huh et al. Citation2010). To simulate in vivo environment of the alveolar space and gas exchange conditions, epithelial cells were exposed at the ALI. After exposure to SiO2 NP aerosols it was observed that : (1) high levels of intercellular adhesion molecule (ICAM)-1 expression from the underlying endothelium cells; (2) increased endothelial capture of circulating neutrophils, promotion of their migration across the permeable membrane (tissue-tissue interface), and accumulation onto epithelial surface; (3) simulated physiological “breathing” by mechanical forces; (4) enhanced absorption of SiO2 NP from the airspace to the microvascular channel; (5) accentuated pro-inflammatory activities and development of acute lung inflammation; and (6) a steady rise in ROS production (Huh Citation2015; Huh et al. Citation2010).
Many other lung-on-a-chip devices emerged, as reported by Punde et al. (Citation2015), Stucki et al. (Citation2015), Blume et al. (Citation2015), Rahimi et al. (Citation2016), Yang et al. (Citation2018), among other research groups. However, nanotoxicity studies using lung-on-a-chip models are still scarce. Zhang et al. (Citation2018) assembled a lung-on-a-chip model, reproducing the alveolar-capillary barrier, using three parallel channels: (1) an alveolar channel with human pulmonary alveolar epithelial cells (HPAEpiC) to embody the alveolar side of the alveolar-capillary barrier; (2) a vessel channel with human endothelial cells (HUVEC) to represent the capillaries, and (3) an ECM channel with Matrigel membrane sandwiching between the alveolar and vessel channels. In this study, Zhang et al. (Citation2018) exposed epithelial cells for 24 h to TiO2 NP and zinc oxide (ZnO) NP that were injected into the alveolar channel. Both NP induced concentration-dependent toxicity on the epithelial and endothelial cells, including elevated ROS generation and apoptosis; however, ZnO NP were more toxic than TiO2 NP. Meghani et al. (Citation2020) designed an alveolus-epithelium-on-a-chip device with in-built sensors for enabling monitorization of pH-responsive ZnO quantum dots (QD)-loaded human serum albumin (HSA) NP. This model comprised lung cancer cells and stromal cells such as fibroblasts along with a collagen ECM. Results showed a significant internalization of the NP under the coculture conditions (Meghani et al. Citation2020).
Exposure conditions: submerged vs air-liquid interface
Most in vitro studies for assessing pulmonary toxicity attributed to (nano)particles were performed using thin (mono)layers of lung cells cultured under submerged conditions, where (nano)particles to be tested were added directly into the cell culture media (Lacroix et al. Citation2018). NP dispersion in complex media such as cell culture medium is likely to change their original physicochemical properties (Kendall et al. Citation2015; Moore et al. Citation2015, Citation2019), which may influence particle-particle and particle-cell interactions, thus markedly affecting lung cell responses. Indeed, the interaction of particles with cell culture media components often leads to the formation of a protein corona (Monopoli et al. Citation2011), which together with media salts and osmolarity may affect particle stability and make particles more prone to aggregation and/or agglomeration (Falahati et al. Citation2019; Teeguarden et al. Citation2007). Another major limitation of submerged cultures is that molecular and cellular features such as expression of key transporters and proteins, cilia formation, mucus, and surfactant secretion may be affected (Acosta et al. Citation2016). At the same time, dose deposition is hard to control as, depending upon their density, particles might remain in suspension and/or interact with material such as plastics where cells were seeded, which may exert an impact on the actual dose that cells are exposed to (Lenz et al. Citation2013). For all the aforementioned, it is widely accepted that in vitro cell exposure to (nano)particles under the classic submerged conditions does not accurately and effectively mimic cellular and physiological features observed in inhalation exposures in vivo. Consequently, the responses detected might also differ from the ones occurring in in vivo situation (Blank, Gehr, and Rothen-Rutishauser Citation2009; Lacroix et al. Citation2018). To overcome the major drawbacks associated with submerged cultures, innovative approaches have emerged over the past years to more accurately control dosimetry and deposition (Polk et al. Citation2016; Secondo, Liu, and Lewinski Citation2017).
Another important issue is the choice of dose metrics. While for non-nano substances dose is normally expressed on the basis of mass, for nanoscale materials the most suitable metrics may be mass, number of particles or surface area, which is yet to be defined since many physicochemical factors including NP surface, shape and size may contribute to their toxicity (Schmid and Cassee Citation2017). In addition, selection of the proper dose range to test is not an easy task. In vitro experiments frequently use significantly higher doses compared to in vivo inhalation studies, making difficult to compare in vitro vs. in vivo responses. Particokinetic models, such as the In Vitro Sedimentation, Diffusion and Dosimetry (ISDD) (Hinderliter et al. Citation2010) or In Vitro Sedimentation, Diffusion, Dissolution, and Dosimetry (ISD3) (Thomas et al. Citation2018) models, describing the delivery of (nano)particles to cells in liquid cell culture systems were useful for dose-response analysis and translation of exposures across in vivo and in vitro systems. At the same time, computational tools including the Multiple Path Particle Dosimetry (MPPD) (Anjilvel and Asgharian Citation1995) and the human respiratory tract model developed by the International Commission on Radiological Protection (ICRP) (Smith et al. Citation2014) were employed to calculate deposition and clearance of particles along the respiratory tract. Not only is the dose important, but also duration and frequency of exposure, with most of the available investigations focusing on the effects of acute exposure, while little is known regarding the long-term implications of chronic exposure to (nano)particles (Bui et al. Citation2020; Kolanjivil and Kleinstreuer Citation2013).
The human airways are not fully immersed in pulmonary fluid, but instead, are covered by surfactant layer and air that creates the ALI, which enables an efficient gas exchange between cells and the environment. Human respiratory system cells such as nasal or bronchial epithelial cells cultured under ALI conditions become effectively polarized and might secrete surfactant, improving the resemblance to the in vivo situation, which is not possible to achieve in fully immersed environments (Barosova et al. Citation2020). In vitro exposure systems are capable of delivering aerosolized (nano)particles directly to the surface of cells cultured under ALI conditions which constitutes a more reliable alternative for conducting pulmonary nanotoxicity studies. depicts the existing aerosol exposure systems available for (nano)particle aerosol generation and cell exposure at ALI. The normal setup generally involves an aerosol generator from powders/dusts or liquid droplets, connections, and peripherals to an exposure chamber with controlled temperature and humidity conditions. Compared to submerged exposures, particle aerosolization and exposure at ALI, where the apical liquid in cultured cells is negligible, seem to have a diminished impact on the particle original properties (Fujitani et al. Citation2015; Polk et al. Citation2016). Some of the available systems are equipped with a quartz crystal microbalance (QCM) for monitoring dose deposition in the ng range, which is a great advantage for controlling deposition over time (Ding et al. Citation2020). Despite their numerous advantages, one needs to take into account existing technical limitations and constraints of the ALI exposure systems. However, only a few commercially available exposure systems allow exposure of cultured cells to aerosolized particles with precise dosimetry (Upadhyay and Palmberg Citation2018). In systems where particle deposition is based upon sedimentation and gravitation, the maximum achieved deposited particle mass is generally low (Bierkandt et al. Citation2018). Particle-specific efficacy of deposition and deposition rate of these aerosol generator devices display considerable variations (e.g., XposeALI®). In addition, a large loading of particles and/or long-term exposure may also be problematic, as particle physicochemical characteristics (e.g., ALICE system) might be affected and particle agglomeration and/or aggregation may occur (Upadhyay and Palmberg Citation2018). Most of the available systems only allow short, single-exposure experiments through a nano aerosol deposition chamber for efficient and quantitative deposition of NP, whereas the nano aerosol chamber in vitro toxicity (NACIVT) system allows for a continuous air-stream (Clippinger et al. Citation2016). Further, these exposure systems require specialized personnel with qualified expertise to set up and operate, being considerably more expensive and time-consuming than the traditional submerged studies (Braakhuis et al. Citation2015)..
Table 6. Aerosol generation and exposure systems for cells cultured under air-liquid interface (ALI) conditions.
Several studies have previously addressed (nano)particles toxicity in human respiratory cells under submerged vs ALI conditions. The vast majority of investigations support the view that ALI cultures seem to be more resistant than submerged cultures to the effects induced by (nano)particles in vitro exposure. In this regard, Lenz et al. (Citation2013) compared cellular responses to ZnO NP (0.7 and 2.5 µg/cm2; immediately and 2 hr after exposure) in terms of oxidative stress [(heme oxygenase 1 (HMOX1), SOD-2 and glutamate-cysteine synthetase, catalytic subunit (GCS) transcription markers expression] and pro-inflammatory (IL-8, IL-6, and GM-CSF levels) responses in human alveolar epithelial A549 cells cultured under ALI or submerged conditions. Overall, a similar response to ZnO NP was observed in A549 cells exposed under both conditions. However, while no significant changes in oxidative stress were found for most markers in both cultures, lower levels of pro-inflammatory responses were detected in A549 cells exposed at ALI than submerged (Lenz et al. Citation2013). Ghio et al. (Citation2013) also compared the influence of ambient air pollution particles, collected in North Carolina outside the United States Environmental Protection Agency (EPA) Human Studies Facility, under submerged and ALI conditions on normal human bronchial epithelial (NHBE) cells by assessing pro-inflammatory (IL-8 and IL-6 levels) and oxidative stress (HOX1 and COX2 expression) markers up to 21 days. Ghio et al. (Citation2013) demonstrated that in NHBE cells exposed at ALI, the tested particles induced reduced biological effects compared to submerged cultures, likely due to higher oxygen availability in ALI cultures.
The comparison of the biological responses of A549 cells under ALI (using a Vitrocell® system equipped with electrodes to enhance deposition by applying an electrostatic field, named ALIDA) or submerged conditions was assessed after exposure to two amorphous SiO2 NP produced by different synthesis methods (Aerosil200 produced by flame synthesis, and 50 nm SiO2 NP produced by the Stöber method). For ALI conditions, the attained deposited doses were 52 μg/cm2 and 117 μg/cm2, for Aerosil200 and 50 nm SiO2 NP, respectively, whereas in submerged conditions both NP were tested as liquid suspensions of 15.6 μg/cm2. The amorphous SiO2 NP aerosols were generated by two different methods: electrospray and atomizer. The electrospray method was only applicable for 50 nm SiO2 NP since it enabled generation of an aerosol containing monodisperse NP, whereas the Aerosil200 suspensions contained excessively large aggregates which disabled a stable operation. However, the deposited mass and surface dose of the 50 nm SiO2 NP was too low to induce cellular responses. On the other hand, the atomizer was applicable for both types of amorphous SiO2 particles; nevertheless, deposition of particle aggregates was observed, and therefore higher mass and surface doses were attained, which led to induction of significant biological effects on lung cells. Overall, both types of amorphous SiO2 NP induced similar cellular responses in both culture systems, although submerged exposure to 50 nm SiO2 NP triggered greater responses at much lower doses (Panas et al. Citation2014).
ALI exposure seems to be a particularly suitable approach when evaluating the toxicity of poorly soluble NP. In this regard, Loret et al. (Citation2016) examined the biological effects of 4 poorly soluble NP-one CeO2 NP and three TiO2 NP-, in A549 alveolar cell monocultures or in coculture with alveolar macrophages (THP-1) using different exposure methods, where the cultures were exposed for 24 hr to aerosolized NP in inserts or to NP liquid suspensions either in inserts or plates. Loret et al. (Citation2016) found that the total deposited doses were reached within 3 hr in inserts, either under ALI or submerged conditions, and within 24 hr in plates. Overall, cocultures were more sensitive than monocultures. It is noteworthy that decreased cell viability, oxidative stress and inflammatory responses were observed at lower doses in cultures exposed under ALI compared to the conventional submerged conditions (Loret et al. Citation2016).
Recently, Medina-Reyes et al. (Citation2020) compared the cytotoxicity, genotoxicity and oxidative stress in A549 cells exposed to TiO2 nanofibers and NP under ALI (using a Vitrocell® Cloud system; 2 and 10 μg/cm2 for 1 or 4 hr and maintained in culture for 24, 48 or 72 hr) and submerged conditions (1–50 μg/cm2 for 24, 48 or 72 hr). Overall, Medina-Reyes et al. (Citation2020) noted that cytotoxicity of TiO2 nanofibers and NP was similar in ALI and submerged cultures, although uptake was higher in submerged than in ALI cultures. However, TiO2 nanofibers induced higher DNA double-strand breaks (DSB) in A549 cultured under ALI than in submerged conditions, while TiO2 NP induced similar levels of DSB in both culture conditions (Medina-Reyes et al. Citation2020). Diabaté et al. (Citation2021) determined the in vitro toxicity of CeO2 and TiO2 NP in A549 monocultures cultured under ALI conditions vs cocultures of A549 with THP-1 macrophages under submerged conditions. Although not the same cell culture model, data demonstrated that cells cultured under ALI conditions were more sensitive to NP-induced toxicity compared to those cultured under submerged conditions, i.e., lower doses of deposited NP (0.2 and 1 µg/cm2) were sufficient to induce adverse outcomes at ALI, as also documented in rodent experiments (Diabaté et al. Citation2021). Similar results were observed by Bessa, Brandão, Fokkens, Cassee et al. (Citation2021), where more pronounced cytotoxicity in A549 cells exposed to aerosolized ATO, CeO2, and ZrO2 NP using a VitroCell® workstation system up to 4 hr exposure compared to A549 submerged cells exposed 24 hr to the same NP as a liquid suspension. Overall, A549 cells under ALI conditions were more susceptible to NP aerosols exposure. Although an increased primary DNA damage regardless of the exposure mode was observed, A549 cells seemed to be more sensitive to the genotoxic effects of ZrO2 NP aerosols than to the same NP in a liquid medium (Bessa, Brandão, Fokkens, Leseman et al. Citation2021).
Conclusions and future perspectives
Over the years, many in vitro studies have been carried out to investigate the potential adverse risks for human health of (nano)particle inhalation. In vitro systems offer highly controlled cell culture environments that may be easily scaled and replicated, enabling identification of the molecular mechanisms associated with (nano)particle exposure responses that are more difficult to evaluate using in vivo models, while complying with the 3Rs principles. Despite considerable progress on advanced in vitro cell and tissue culture technologies that has been made thus far, many aspects are still challenging, need to be improved and more comprehensively explored. For instance, it would be interesting to use these systems to address the translocation of (nano)particles from lung to the lymph nodes and bloodstream. In addition, exploring particle localization at their sites of interaction with living structures would be of relevance. Here, the application of imaging techniques such as Raman microspectroscopy and X-ray microscopy may be feasible, particularly for simultaneous visualization of (nano)particles within the airways and the determination of cell and tissue changes that occur. For example, these novel techniques would be valuable for a more comprehensive understanding of (nano)particles diminished phagocytosis by alveolar macrophages and its impact on lung toxicity.
Most in vitro research on the effects of (nano)particles in the human respiratory system are focused on acute exposures, but information on the repeated and long term (chronic) effects of relevance in occupational settings is still scarce. However, assessment of such effects in vitro is currently still extremely challenging. Therefore, future studies need to address this major research gap that may have a significant impact in the regulatory field, for establishing appropriate legislation and occupational exposure limits (OEL) for inhaled (nano)particles.
A common criticism of in vitro models is that they are usually more sensitive than in vivo models to (nano)particle exposure. Contributing to this fact are the high concentrations tested in vitro compared to the ones used in vivo, making in vitro vs. in vivo responses hard to compare. Advancement of knowledge on particulate environmental and occupational exposure levels that humans are exposed to is crucial for improving experimental design of in vitro (and in vivo) inhalation toxicity studies. Here, mathematical approaches for predicting particle deposition across the respiratory tract and for estimating in vitro concentrations to be tested from in vivo doses are also valuable. At the same time, it would also be important to identify which is the best metric for expressing (nano)particle dose.
Although the currently available respiratory in vitro systems fail to replicate the entire hierarchical and complex architecture and biomechanics of the human lung, these models provide useful information depending upon the way the study hypothesis is posed. The latest advancements on the use of human pluripotent stem cells in 3D models of lung development and disease, the use of computational methodologies in respiratory cell models and exposure systems, or development of lung-on-a-chip devices that simulates airflow and breathing patterns, are promising and certainly will assist scientists in the future to answer questions in the field of (nano)particle inhalation toxicology.
Acknowledgments
This work was supported by the Portuguese Foundation for Science and Technology (FCT) under grant SIINN/0004/2014, in the framework of the CERASAFE project. This work was also supported by the NanoBioBarriers project (PTDC/MED‐TOX/31162/2017), co-financed by the Operational Program for Competitiveness and Internationalization (POCI) through European Regional Development Funds (FEDER/FNR), by the NanoLegaTox project (POCI-01-0145-FEDER-029651) financed by FEDER through the COMPETE 2020 - POCI, and by Portuguese funds through Fundação para a Ciência e a Tecnologia (FCT), by the Spanish Ministry of Science and Innovation: MCIN/AEI/10.13039/501100011033 (Grant PID2020-114908GA-I00), and by Xunta de Galicia (ED431B 2022/16). M.J. Bessa and F. Brandão are recipients of FCT PhD scholarships, supported by the Human Capital Operating Program (POCH) and European Union funding, under grants SFRH/BD/120646/2016 and SFRH/BD/101060/2014, respectively. ICBAS – U.Porto provided additional fundamental support. A.T. Reis thanks the financial support of FCT through individual Grant SFRH/BPD/122112/2016. V. Valdiglesias acknowledges support from Spanish Ministry of Education, Culture and Sport (BEAGAL18/00142). S. Fraga thanks FCT for funding through program DL 57/2016 – Norma transitória (Ref. DL-57/INSA-06/2018). Thanks are also due to FCT/MCTES for the financial support to EPIUnit (UIDB/04750/2020) and ITR (LA/P/0064/2020).
Disclosure statement
No potential conflict of interest was reported by the authors.
Data availability statement
Data sharing is not applicable to this article as no new data were created or analyzed in this study.
Additional information
Funding
References
- Acosta, M. F., P. Muralidharan, S. A. Meenach, D. Hayes, M. Black S, and H. M. Mansour. 2016. In vitro pulmonary cell culture in pharmaceutical inhalation aerosol delivery: 2-D, 3-D, and in situ bioimpactor models. Curr. Pharm. Des. 22 (17):2522–31. doi:10.2174/1381612822666160202142104.
- Alsaleh, N. B. 2021. Adverse cardiovascular responses of engineered nanomaterials: Current understanding of molecular mechanisms and future challenges. Nanomedicine 37:102421. doi:10.1016/j.nano.2021.102421.
- Andujar, P., S. Lanone, P. Brochard, and J. Boczkowski. 2011. Respiratory effects of manufactured nanoparticles. Rev. Mal. Respir. 28 (8):e66–75. doi:10.1016/j.rmr.2011.09.008.
- Anjilvel, S., and B. Asgharian. 1995. A multiple-path model of particle deposition in the rat lung. Fundam. Appl. Toxicol. 28 (1):41–50. doi:10.1006/faat.1995.1144.
- Archer, F., A. Bobet-Erny, and M. Gomes. 2021. State of the art on lung organoids in mammals. Vet. Res. 52 (1): 77-77. doi:10.1186/s13567-021-00946-6.
- Aufderheide, M., and U. Mohr. 1999. CULTEX — a new system and technique for the cultivation and exposure of cells at the air/liquid interface. Exp. Toxicol. Pathol. 51 (6):489–90. doi:10.1016/S0940-2993(99)80121-3.
- Aufderheide, M., and U. Mohr. 2004. A modified CULTEX® system for the direct exposure of bacteria to inhalable substances. Exp. Toxicol. Pathol. 55 (6):451–54. doi:10.1078/0940-2993-00348.
- Bakand, S., and A. Hayes. 2016. Toxicological considerations, toxicity assessment, and risk management of inhaled nanoparticles. Int. J. Mol. Sci. 17 (6):929. doi:10.3390/ijms17060929.
- Bakand, S., A. Hayes, and F. Dechsakulthorn. 2012. Nanoparticles: A review of particle toxicology following inhalation exposure. Inhal. Toxicol. 24 (2):125–35. doi:10.3109/08958378.2010.642021.
- Barosova, H., A. G. Maione, D. Septiadi, M. Sharma, L. Haeni, S. Balog, O. O’Connell, G. R. Jackson, D. Brown, A. J. Clippinger, et al. 2020. Use of EpiAlveolar lung model to predict fibrotic potential of multiwalled carbon nanotubes. ACS Nano 14:3941–56. doi:10.1021/acsnano.9b06860.
- Bassi, G., M. A. Grimaudo, S. Panseri, and M. Montesi. 2021. Advanced multi-dimensional cellular models as emerging reality to reproduce in vitro the human body complexity. Int. J. Mol. Sci. 22 (3):1195. doi:10.3390/ijms22031195.
- Benainous, H., V. Kilin, S. Huang, L. Wiszniewski, J.-P. Wolf, L. Bonacina, S. Constant, and C. Mas. 2018. OncoCilair™: A physiological in vitro platform to assess the efficacy and the toxicity of lung cancer therapeutics. Toxicol. Lett. 295:S122. doi:10.1016/j.toxlet.2018.06.670.
- Bengalli, R., P. Mantecca, M. Camatini, and M. Gualtieri. 2013. Effect of nanoparticles and environmental particles on a cocultures model of the air-blood barrier. Biomed. Res. Int. 2013:801214. doi:10.1155/2013/801214.
- Bessa, M. J., F. Brandão, P. Fokkens, F. R. Cassee, A. Salmatonidis, M. Viana, A. Vulpoi, S. Simon, E. Monfort, J. P. Teixeira, et al. 2021. Toxicity assessment of industrial engineered and airborne process-generated nanoparticles in a 3D human airway epithelial in vitro model. Nanotoxicology 1–16. doi:10.1080/17435390.2021.1897698.
- Bessa, M. J., F. Brandão, P. H. B. Fokkens, D. L. A. C. Leseman, A. J. F. Boere, F. R. Cassee, A. Salmatonidis, M. V. A. Vulpoi, S. Simon, E. Monfort, et al. 2021. In vitro toxicity of industrially relevant engineered nanoparticles in human alveolar epithelial cells: Air-liquid interface versus submerged cultures. Nanomaterials 11:3225.
- Bierkandt, F. S., L. Leibrock, S. Wagener, P. Laux, and A. Luch. 2018. The impact of nanomaterial characteristics on inhalation toxicity. Toxicol. Res. (Camb.) 7 (3):321–46. doi:10.1039/c7tx00242d.
- Blank, F., P. Gehr, and B. Rothen-Rutishauser. 2009. In vitro human lung cell culture models to study the toxic potential of nanoparticles. Nanotoxicity: From in Vitro, in vivo Models to Health Risks.
- Blume, C., R. Reale, M. Held, T. M. Millar, J. E. Collins, D. E. Davies, H. Morgan, and E. J. Swindle. 2015. Temporal monitoring of differentiated human airway epithelial cells using microfluidics. PLoS One 10 (10):e0139872. doi:10.1371/journal.pone.0139872.
- Boraschi, D., P. Italiani, R. Palomba, P. Decuzzi, A. Dushl, B. Fadeel, and S. M. Moghimi. 2017. Nanoparticles and innate immunity: New perspectives on host defence. Semin. Immunol. 34:33–51. doi:10.1016/j.smim.2017.08.013.
- Braakhuis, H. M., S. K. Kloet, S. Kezic, F. Kuper, M. V. Park, S. Bellmann, M. van der Zande, S. Le Gac, P. Krystek, R. J. Peters, et al. 2015. Progress and future of in vitro models to study translocation of nanoparticles. Arch. Toxicol. 89 (9):1469–95. doi:10.1007/s00204-015-1518-5.
- Braakhuis, H. M., A. G. Oomen, and F. R. Cassee. 2016. Grouping nanomaterials to predict their potential to induce pulmonary inflammation. Toxicol. Appl. Pharmacol. 299:3–7. doi:10.1016/j.taap.2015.11.009.
- Braakhuis, H. M., M. V. Park, I. Gosens, W. H. De Jong, and F. R. Cassee. 2014. Physicochemical characteristics of nanomaterials that affect pulmonary inflammation. Part Fibre Toxicol. 11 (1):18. doi:10.1186/1743-8977-11-18.
- Brook, R. D., B. Franklin, W. Cascio, Y. Hong, G. Howard, M. Lipsett, R. Luepker, M. Mittleman, J. Samet, and S. C. Smith Jr. 2004. Air pollution and cardiovascular disease: A statement for healthcare professionals from the expert panel on population and prevention science of the American heart association. Circulation 109 (21):2655–71. doi:10.1161/01.CIR.0000128587.30041.C8.
- Bui, V., K. Hoang, J.-Y. Moon, M. Chae, D. Park, and Y.-C. Lee. 2020. Prediction of aerosol deposition in the human respiratory tract via computational models: A review with recent updates. Atmosphere 11 (2):137. doi:10.3390/atmos11020137.
- Camassa, L. M. A., E. Elje, E. Mariussen, E. M. Longhin, M. Dusinska, S. Zienolddiny-Narui, and E. Runden-Pran. 2022. Advanced respiratory models for hazard assessment of nanomaterials—Performance of Mono-, Co- and Tricultures. Nanomaterials 12 (15):2609. doi:10.3390/nano12152609.
- Carneiro, M. F. H., and F. Barbosa Jr. 2016. Gold nanoparticles: A critical review of therapeutic applications and toxicological aspects. J. Toxicol. Environ. Health B 19 (3–4):129–48. doi:10.1080/10937404.2016.1168762.
- Carvalho, V., N. Rodrigues, R. Ribeiro, P. F. Costa, R. A. Lima, and S. F. Teixeira. 2020. 3D printed biomodels for flow visualization in stenotic vessels: An experimental and numerical study. Micromachines 11 (6):549. doi:10.3390/mi11060549.
- Chen, Y.-W., S. X. Huang, A. L. R. T. de Carvalho, S.-H. Ho, M. N. Islam, S. Volpi, L. D. Notarangelo, M. Ciancanelli, J.-L. Casanova, J. Bhattacharya, et al. 2017. A three-dimensional model of human lung development and disease from pluripotent stem cells. Nat. Cell Biol. 19 (5):542–49. doi:10.1038/ncb3510.
- Chortarea, S., H. Barosova, M. J. D. Clift, P. Wick, A. Petri-Fink, and B. Rothen-Rutishauser. 2017. Human asthmatic bronchial cells are more susceptible to subchronic repeated exposures of aerosolized carbon nanotubes at occupationally relevant doses than healthy cells. ACS Nano 11 (8):7615–25. doi:10.1021/acsnano.7b01992.
- Chortarea, S., M. J. Clift, D. Vanhecke, C. Endes, P. Wick, A. Petri-Fink, and B. Rothen-Rutishauser. 2015. Repeated exposure to carbon nanotube-based aerosols does not affect the functional properties of a 3D human epithelial airway model. Nanotoxicology 9 (8):983–93. doi:10.3109/17435390.2014.993344.
- Clippinger, A. J., A. Ahluwalia, D. Allen, J. C. Bonner, W. Casey, V. Castranova, R. M. David, S. Halappanavar, J. A. Hotchkiss, A. M. Jarabek, et al. 2016. Expert consensus on an in vitro approach to assess pulmonary fibrogenic potential of aerosolized nanomaterials. Arch. Toxicol. 90 (7):1769–83. doi:10.1007/s00204-016-1717-8.
- Clippinger, A. J., D. Allen, A. M. Jarabek, M. Corvaro, M. Gaca, S. Gehen, J. A. Hotchkiss, G. Patlewicz, J. Melbourne, P. Hinderliter, et al. 2018. Alternative approaches for acute inhalation toxicity testing to address global regulatory and non-regulatory data requirements: An international workshop report. Toxicol. In Vitro 48:53–70. doi:10.1016/j.tiv.2017.12.011.
- Cores, J., P.-U. C. Dinh, T. Hensley, K. B. Adler, L. J. Lobo, and K. Cheng. 2020. A pre-investigational new drug study of lung spheroid cell therapy for treating pulmonary fibrosis. Stem Cells Transl. Med. 9 (7):786–98. doi:10.1002/sctm.19-0167.
- Costa, E. C., V. M. Gaspar, J. G. Marques, P. Coutinho, and I. J. Correia. 2013. Evaluation of nanoparticle uptake in co-culture cancer models. PloS One 8 (7):e70072. doi:10.1371/journal.pone.0070072.
- Crapo, J. D., B. E. Barry, P. Gehr, M. Bachofen, and E. R. Weibel. 1982. Cell number and cell characteristics of the normal human lung. Am. Rev. Respir. Dis. 126 (2):332–37. doi:10.1164/arrd.1982.126.2.332.
- Dankers, A. C. A., C. F. Kuper, A. J. Boumeester, B. O. Fabriek, I. M. Kooter, M. Grollers-Mulderij, P. Tromp, I. Nelissen, E. K. Zondervan-Van Den Beuken, and R. J. Vandebriel. 2018. A practical approach to assess inhalation toxicity of metal oxide nanoparticles in vitro. J. Appl. Toxicol. 38 (2):160–71. doi:10.1002/jat.3518.
- Darquenne, C. 2020. Deposition mechanisms. J. Aerosol. Med. Pulm Drug Delivery 33 (4):181–85. doi:10.1089/jamp.2020.29029.cd.
- de Bruijne, K., S. Ebersviller, K. G. Sexton, S. Lake, D. Leith, R. Goodman, J. Jetters, G. W. Walters, M. Doyle-Eisele, R. Woodside, et al. 2009. Design and testing of electrostatic aerosol in vitro exposure system (EAVES): An alternative exposure system for particles. Inhal. Toxicol. 21 (2):91–101. doi:10.1080/08958370802166035.
- Degobbi, C., P. H. N. Saldiva, and C. Rogers. 2011. Endotoxin as modifier of particulate matter toxicity: A review of the literature. Aerobiologia 27 (2):97–105. doi:10.1007/s10453-010-9179-6.
- De Jong, W. H., and P. J. A. Borm. 2008. Drug delivery and nanoparticles:Applications and hazards. Int. J. Nanomed. 3:133–49. doi:10.2147/IJN.S596.
- Delfino, R. J., C. Sioutas, and S. Malik. 2005. Potential role of ultrafine particles in associations between airborne particle mass and cardiovascular health. Environ. Health Perspect 113 (8):934–46. doi:10.1289/ehp.7938.
- Diabaté, S., L. Armand, S. Murugadoss, M. Dilger, S. Fritsch-Decker, C. Schlager, D. Béal, M.-E. Arnal, M. Biola-Clier, S. Ambrose, et al. 2021. Air–liquid interface exposure of lung epithelial cells to low doses of nanoparticles to assess pulmonary adverse effects. Nanomaterials 11 (1):65. doi:10.3390/nano11010065.
- Di Cristo, L., F. Boccuni, S. Iavicoli, and S. Sabella. 2020. A human-relevant 3D in vitro platform for an effective and rapid simulation of workplace exposure to nanoparticles: Silica nanoparticles as case study. Nanomaterials 10 (9):1761. doi:10.3390/nano10091761.
- Ding, Y., P. Weindl, A. G. Lenz, P. Mayer, T. Krebs, and O. Schmid. 2020. Quartz crystal microbalances (QCM) are suitable for real-time dosimetry in nanotoxicological studies using VITROCELL®Cloud cell exposure systems. Part Fibre Toxicol. 17 (1):44. doi:10.1186/s12989-020-00376-w.
- Docter, D., D. Westmeier, M. Markiewicz, S. Stolte, S. K. Knauer, and R. H. Stauber. 2015. The nanoparticle biomolecule corona: Lessons learned–challenge accepted? Chem. Soc. Rev. 44 (17):6094–121. doi:10.1039/C5CS00217F.
- Duret, C., N. Wauthoz, R. Merlos, J. Goole, C. Maris, I. Roland, T. Sebti, F. Vanderbist, and K. Amighi. 2012. In vitro and in vivo evaluation of a dry powder endotracheal insufflator device for use in dose-dependent preclinical studies in mice. Eur. J. Pharm. Biopharm. 81 (3):627–34. doi:10.1016/j.ejpb.2012.04.004.
- Dvorak, A., A. E. Tilley, R. Shaykhiev, R. Wang, and R. G. Crystal. 2011. Do Airway Epithelium Air–Liquid Cultures Represent the in vivo Airway Epithelium Transcriptome? Am. J. Respir. Cell Mol. Biol. 44 (4):465–73. doi:10.1165/rcmb.2009-0453OC.
- Edmondson, R., J. J. Broglie, A. F. Adcock, and L. Yang. 2014. Three-dimensional cell culture systems and their applications in drug discovery and cell-based biosensors. Assay Drug Dev. Technol. 12 (4):207–18. doi:10.1089/adt.2014.573.
- European Commission. 2022. Commission recommendation of 10 June 2022 on the definition of nanomaterial. https://eur-lex.europa.eu/legal-content/EN/TXT/?uri=CELEX%3A32022H0614%2801%29&qid=1655218986567.
- Faber, S. C., and S. D. McCullough. 2018. Through the looking glass: In vitro models for inhalation toxicology and interindividual variability in the airway. Appl. In Vitro Toxicol. 4 (2):115–28. doi:10.1089/aivt.2018.0002.
- Falahati, M., F. Attar, M. Sharifi, T. Haertlé, J. F. Berret, R. H. Khan, and A. A. Saboury. 2019. A health concern regarding the protein corona, aggregation and disaggregation. Biochim. Biophys. Acta Gen. Subj. 1863 (5):971–91. doi:10.1016/j.bbagen.2019.02.012.
- Ferraz, E. R. A., C. R. Rainho, A. S. Fernandes, and I. Felzenszwalb. 2016. Differential toxicity of an organic PM2.5 extract to human lung cells cultured in three dimensions (3D) and monolayers. J. Toxicol. Environ. Health Part A 79 (5):221–31. doi:10.1080/15287394.2016.1143902.
- Fizesan, I., S. Cambier, E. Moschini, A. Chary, I. Nelissen, J. Ziebel, J. N. Audinot, T. Wirtz, M. Kruszewski, A. Pop, et al. 2019. In vitro exposure of a 3D-tetraculture representative for the alveolar barrier at the air-liquid interface to silver particles and nanowires. Part Fibre Toxicol. 16 (1):14. doi:10.1186/s12989-019-0297-1.
- Frieke Kuper, C., M. Grollers-Mulderij, T. Maarschalkerweerd, N. M. Meulendijks, A. Reus, F. van Acker, E. K. Zondervan-van den Beuken, M. E. Wouters, S. Bijlsma, and I. M. Kooter. 2015. Toxicity assessment of aggregated/agglomerated cerium oxide nanoparticles in an in vitro 3D airway model: The influence of mucociliary clearance. Toxicol. In Vitro 29 (2):389–97. doi:10.1016/j.tiv.2014.10.017.
- Frijns, E., S. Verstraelen, L. C. Stoehr, J. V. Laer, A. Jacobs, J. Peters, K. Tirez, M. S. P. Boyles, M. Geppert, P. Madl, et al. 2017. A novel exposure system termed NAVETTA for in vitro laminar flow electrodeposition of nanoaerosol and evaluation of immune effects in human lung reporter cells. Environ. Sci. Technol. 51 (9):5259–69. doi:10.1021/acs.est.7b00493.
- Fröhlich, E. 2018. Comparison of conventional and advanced in vitro models in the toxicity testing of nanoparticles. Artif. Cells, Nanomed. Biotechnol. 46 (Suppl 2):1091–107. doi:10.1080/21691401.2018.1479709.
- Fröhlich, E., and S. Salar-Behzadi. 2014. Toxicological assessment of inhaled nanoparticles: Role of in vivo, ex vivo, in vitro, and in silico studies. Int. J. Mol. Sci. 15 (3):4795–822. doi:10.3390/ijms15034795.
- Fujitani, Y., Y. Sugaya, M. Hashiguchi, A. Furuyama, S. Hirano, and A. Takami. 2015. Particle deposition efficiency at air–liquid interface of a cell exposure chamber. J. Aerosol. Sci. 81:90–99. doi:10.1016/j.jaerosci.2014.10.012.
- Fytianos, K., B. Drasler, F. Blank, C. von Garnier, E. Seydoux, L. Rodriguez-Lorenzo, A. Petri-Fink, and B. Rothen-Rutishauser. 2016. Current in vitro approaches to assess nanoparticle interactions with lung cells. Nanomedicine (Lond.) 11 (18):2457–69. doi:10.2217/nnm-2016-0199.
- Geiser, M. 2010. Update on macrophage clearance of inhaled micro-and nanoparticles. J. Aerosol. Med. Pulm Drug Deliv. 23 (4):207–17. doi:10.1089/jamp.2009.0797.
- George, I., C. Uboldi, E. Bernard, M. S. Sobrido, S. Dine, A. Hagege, D. Vrel, N. Herlin, J. Rose, T. Orsiere, et al. 2019. Toxicological assessment of ITER-like tungsten nanoparticles using an in vitro 3D human airway epithelium model. Nanomater. (Basel) 9 (10):1374. doi:10.3390/nano9101374.
- George, I., S. Vranic, S. Boland, A. Courtois, and A. Baeza-Squiban. 2015. Development of an in vitro model of human bronchial epithelial barrier to study nanoparticle translocation. Toxicol. In Vitro 29 (1):51–58. doi:10.1016/j.tiv.2014.08.003.
- Ghio, A. J., L. A. Dailey, J. M. Soukup, J. Stonehuerner, J. H. Richards, and R. B. Devlin. 2013. Growth of human bronchial epithelial cells at an air-liquid interface alters the response to particle exposure. Part Fibre Toxicol. 10 (1):25. doi:10.1186/1743-8977-10-25.
- Gkatzis, K., S. Taghizadeh, D. Huh, D. Y. R. Stainier, and S. Bellusci. 2018. Use of three-dimensional organoids and lung-on-a-chip methods to study lung development, regeneration and disease. Eur. Respir. J. 52 (5):1800876. doi:10.1183/13993003.00876-2018.
- Goers, L., P. Freemont, and K. M. Polizzi. 2014. Co-culture systems and technologies: Taking synthetic biology to the next level. J. Roy. Soc. Interface 11 (96):20140065. doi:10.1098/rsif.2014.0065.
- Gonzalez-Mariscal, L., R. G. Contreras, J. J. Bolívar, A. Ponce, B. Chávez De Ramirez, and M. Cereijido. 1990. Role of calcium in tight junction formation between epithelial cells. Am. J. Physiol. 259 (6):C978–86. doi:10.1152/ajpcell.1990.259.6.C978.
- Gotoh, S., I. Ito, T. Nagasaki, Y. Yamamoto, S. Konishi, Y. Korogi, H. Matsumoto, S. Muro, T. Hirai, M. Funato, et al. 2014. Generation of alveolar epithelial spheroids via isolated progenitor cells from human pluripotent stem cells. Stem Cell Rep. 3 (3):394–403. doi:10.1016/j.stemcr.2014.07.005.
- Guo, C., A. Buckley, T. Marczylo, J. Seiffert, I. Romer, J. Warren, A. Hodgson, K. F. Chung, T. W. Gant, R. Smith, et al. 2018. The small airway epithelium as a target for the adverse pulmonary effects of silver nanoparticle inhalation. Nanotoxicology 12 (6):539–53. doi:10.1080/17435390.2018.1465140.
- Gustafson, H. H., D. Holt-Casper, D. W. Grainger, and H. Ghandehari. 2015. Nanoparticle uptake: The phagocyte problem. Nano Today 10 (4):487–510. doi:10.1016/j.nantod.2015.06.006.
- Hagens, W. I., A. G. Oomen, W. H. de Jong, F. R. Cassee, and A. J. Sips. 2007. What do we (need to) know about the kinetic properties of nanoparticles in the body? Regul. Toxicol. Pharmacol. 49 (3):217–29. doi:10.1016/j.yrtph.2007.07.006.
- Harkema, J. R., S. A. Carey, J. G. Wagner, S. M. Dintzis, and D. Liggitt. 2012. Nose, sinus, pharynx, and larynx. In Comparative anatomy and histology, ed. P. M. Treuting, and S. M. Dintzis, 71–94. San Diego: Academic Press. Chapter 6.
- Hastedt, J. E., P. Bäckman, A. R. Clark, W. Doub, A. Hickey, G. Hochhaus, P. J. Kuehl, C.-M. Lehr, P. Mauser, and J. McConville. 2016. Scope and relevance of a pulmonary biopharmaceutical classification system AAPS/FDA/USP workshop March 16-17th, 2015 in Baltimore, MD. AAPS Open 2 (1). doi:10.1186/s41120-015-0002-x.
- He, X. 2016. In vivo nanotoxicity assays in animal models. Toxicol. Nanomater. 153:1–14.
- Hiemstra, P. S., G. Grootaers, A. M. van der Does, C. A. M. Krul, and I. M. Kooter. 2018. Human lung epithelial cell cultures for analysis of inhaled toxicants: Lessons learned and future directions. Toxicol. In Vitro 47:137–46. doi:10.1016/j.tiv.2017.11.005.
- Hiemstra, P. S., P. B. McCray Jr., and R. Bals. 2015. The innate immune function of airway epithelial cells in inflammatory lung disease. Eur. Respir. J. 45 (4):1150–62. doi:10.1183/09031936.00141514.
- Hinderliter, P. M., K. R. Minard, G. Orr, W. B. Chrisler, B. D. Thrall, J. G. Pounds, and J. G. Teeguarden. 2010. ISDD: A computational model of particle sedimentation, diffusion and target cell dosimetry for in vitro toxicity studies. Particle Fibre Toxicol. 7 (1):36. doi:10.1186/1743-8977-7-36.
- Hofer, M., and M. P. Lutolf. 2021. Engineering organoids. Nat. Rev. Mater. 6 (5):402–20. doi:10.1038/s41578-021-00279-y.
- Huh, D. D. 2015. A human breathing lung-on-a-chip. Ann. Am. Thorac. Soc. 12 (Suppl 1):S42–44. doi:10.1513/AnnalsATS.201410-442MG.
- Huh, D., B. D. Matthews, A. Mammoto, M. Montoya-Zavala, H. Y. Hsin, and D. E. Ingber. 2010. Reconstituting organ-level lung functions on a chip. Science 328 (5986):1662–68. doi:10.1126/science.1188302.
- Ianni, E. D., N. R. Jacobsen, U. B. Vogel, and P. Moller. 2022. Systematic review on primary and secondary genotoxicity of carbon black nanoparticles in mammalian cells and animals. Mutat. Res. Rev. Mutat. Res. 790:108441. doi:10.1016/j.mrrev.2022.108441.
- Inhalation Sciences. 2021. XposeALI ® - 3D in vitro cell exposure. Accessed 2021. https://inhalation.se/products/xposeali-cell-exposures/.
- Jackson, E. L., and H. Lu. 2016. Three-dimensional models for studying development and disease: Moving on from organisms to organs-on-a-chip and organoids. Integr. Biol.: Quant. Biosci. Nano Macro. 8 (6):672–83. doi:10.1039/C6IB00039H.
- Jain, A., and S. Thareja. 2019. In vitro and in vivo characterization of pharmaceutical nanocarriers used for drug delivery. Artif. Cells, Nanomed. Biotechnol. 47 (1):524–39. doi:10.1080/21691401.2018.1561457.
- Jakobsson, J. K. F., H. L. Aaltonen, H. Nicklasson, A. Gudmundsson, J. Rissler, P. Wollmer, and J. Löndahl. 2018. Altered deposition of inhaled nanoparticles in subjects with chronic obstructive pulmonary disease. BMC Pulm Med. 18 (1):129. doi:10.1186/s12890-018-0697-2.
- Jeannet, N., M. Fierz, M. Kalberer, H. Burtscher, and M. Geiser. 2015. Nano aerosol chamber for in-vitro toxicity (NACIVT) studies. Nanotoxicology 9 (1):34–42. doi:10.3109/17435390.2014.886739.
- Jia, Y. Y., Q. Wang, and T. Liu. 2017. Toxicity research of PM2.5 compositions in vitro. Int. J. Environ. Res. Public Health 14 (3):232. doi:10.3390/ijerph14030232.
- Johnson, N. M., A. R. Hoffmann, J. C. Behlen, C. Lau, D. Pendleton, N. Harvey, R. Shore, Y. Li, J. Chen, and Y. Tian. 2021. Air pollution and children’s health—a review of adverse effects associated with prenatal exposure from fine to ultrafine particulate matter. Environ. Health Prev. Med. 26 (1):1–29. doi:10.1186/s12199-021-00995-5.
- Joris, F., B. B. Manshian, K. Peynshaert, S. C. De Smedt, K. Braeckmans, and S. J. Soenen. 2013. Assessing nanoparticle toxicity in cell-based assays: Influence of cell culture parameters and optimized models for bridging the in vitro–in vivo gap. Chem. Soc. Rev. 42 (21):8339–59. doi:10.1039/c3cs60145e.
- Kabadi, P. K., A. L. Rodd, A. E. Simmons, N. J. Messier, R. H. Hurt, and A. B. Kane. 2019. A novel human 3D lung microtissue model for nanoparticle-induced cell-matrix alterations. Part Fibre Toxicol. 16 (1):15. doi:10.1186/s12989-019-0298-0.
- Kan, H., D. Pan, and V. Castranova. 2018. Engineered nanoparticle exposure and cardiovascular effects: The role of a neuronal-regulated pathway. Inhal. Toxicol. 30 (9–10):335–42. doi:10.1080/08958378.2018.1535634.
- Kar, S. K., J. M. Wells, E. D. Ellen, M. F. W. Te Pas, O. Madsen, M. A. M. Groenen, and H. Woelders. 2021. Organoids: A promising new in vitro platform in livestock and veterinary research. Vet. Res. 52 (1):43. doi:10.1186/s13567-021-00904-2.
- Kasper, J., M. I. Hermanns, C. Bantz, M. Maskos, R. Stauber, C. Pohl, R. E. Unger, and J. C. Kirkpatrick. 2011. Inflammatory and cytotoxic responses of an alveolar-capillary coculture model to silica nanoparticles: Comparison with conventional monocultures. Part Fibre Toxicol. 8 (1):6. doi:10.1186/1743-8977-8-6.
- Kastlmeier, M., E. M. Guenther, T. Stoeger, and C. Voss. 2022. Lung organoids for hazard assessment of nanomaterials. Int. J. Mol. Sci. 23 (24):145666. doi:10.3390/ijms232415666.
- Katt, M. E., A. L. Placone, A. D. Wong, Z. S. Xu, and P. C. Searson. 2016. In vitro tumor models: Advantages, disadvantages, variables, and selecting the right platform. Front Bioeng. Biotechnol. 4:12. doi:10.3389/fbioe.2016.00012.
- Kelly, F. J., and J. C. Fussell. 2015. Air pollution and public health: Emerging hazards and improved understanding of risk. Environ. Geochem. Health 37 (4):631–49. doi:10.1007/s10653-015-9720-1.
- Kendall, M., N. J. Hodges, H. Whitwell, J. Tyrrell, and H. Cangul. 2015. Nanoparticle growth and surface chemistry changes in cell-conditioned culture medium. Phil. Trans. R S London. Series B, Biol. Sci. 370 (1661):20140100. doi:10.1098/rstb.2014.0100.
- Kendall, M., and S. Holgate. 2012. Health impact and toxicological effects of nanomaterials in the lung. Respirology 17 (5):743–58. doi:10.1111/j.1440-1843.2012.02171.x.
- Kermanizadeh, A., I. Gosens, L. MacCalman, H. Johnston, P. H. Danielsen, N. R. Jacobsen, A.-G. Lenz, T. Fernandes, R. P. F. Schins, F. R. Cassee, et al. 2016. A multilaboratory toxicological assessment of a panel of 10 engineered nanomaterials to human health—ENPRA project—The highlights, limitations, and current and future challenges. J. Toxicol. Environ. Health B 19 (1):1–28. doi:10.1080/10937404.2015.1126210.
- Kermanizadeh, A., L. G. Powell, and V. Stone. 2020. A review of hepatic nanotoxicology – summation of recent findings and considerations for the next generation of study designs. J. Toxicol. Environ. Health B 23 (4):136–76. doi:10.1080/10937404.2020.1751756.
- Khan, Y. S., and D. T. Lynch. 2018. Histology of the lung.
- Klein, S. G., T. Serchi, L. Hoffmann, B. Blömeke, and A. C. Gutleb. 2013. An improved 3D tetraculture system mimicking the cellular organisation at the alveolar barrier to study the potential toxic effects of particles on the lung. Part Fibre Toxicol. 10 (1):31. doi:10.1186/1743-8977-10-31.
- Kleinstreuer, C., and Z. Zhang. 2010. Airflow and particle transport in the human respiratory system. Annu. Rev. Fluid Mech. 42 (1):301–34. doi:10.1146/annurev-fluid-121108-145453.
- Kletting, S., S. Barthold, U. Repnik, G. Griffiths, B. Loretz, N. Schneider-Daum, C. de Souza Carvalho-Wodarz, and C.-M. Lehr. 2018. Co-culture of human alveolar epithelial (hAelvi) and macrophage (THP-1) cell lines. ALTEX 35:211–22. doi:10.14573/altex.1607191.
- Knaapen, A. M., P. J. Borm, C. Albrecht, and R. P. Schins. 2004. Inhaled particles and lung cancer. Part A: Mechanisms. Int. J. Cancer 109 (6):799–809. doi:10.1002/ijc.11708.
- Kobos, L. S. A., L. Xia, R. Coltellino, V. Kishman, C. McIlrath, D. Perez-Torres, and J. Shannahan. 2020. Comparison of silver nanoparticle-induced inflammatory responses between healthy and metabolic syndrome mouse models. J. Toxicol. Environ. Health Part A 83 (7):249–68. doi:10.1080/15287394.2020.1748779.
- Kolanjivil, A. V., and C. Kleinstreuer. 2013. Nanoparticle mass transfer from lung airways to systemic regions—Part I: Whole-lung aerosol dynamics. J. Biomech. Eng. 135 (12). doi:10.1115/1.4025332.
- Kooter, I. M., M. Gröllers-Mulderij, E. Duistermaat, F. Kuper, and E. D. Schoen. 2017. Factors of concern in a human 3D cellular airway model exposed to aerosols of nanoparticles. Toxicol. In Vitro 44:339–48. doi:10.1016/j.tiv.2017.07.006.
- Kreyling, W. G., M. Semmler-Behnke, and W. Möller. 2006. Health implications of nanoparticles. J. Nanopart Res. 8 (5):543–62. doi:10.1007/s11051-005-9068-z.
- Kumar, V., N. Sharma, and S. S. Maitra. 2017. In vitro and in vivo toxicity assessment of nanoparticles. Int. Nano Lett. 7 (4):243–56. doi:10.1007/s40089-017-0221-3.
- Lacroix, G., W. Koch, D. Ritter, A. C. Gutleb, S. T. Larsen, T. Loret, F. Zanetti, S. Constant, S. Chortarea, B. Rothen-Rutishauser, et al. 2018. Air–liquid interface in vitro models for respiratory toxicology research: Consensus workshop and recommendations. Appl. In Vitro Toxicol. 4 (2):91–106. doi:10.1089/aivt.2017.0034.
- Langhans, S. A. 2018. Three-dimensional in vitro cell culture models in drug discovery and drug repositioning. Front Pharmacol. 9:6. doi:10.3389/fphar.2018.00006.
- Leibrock, L., S. Wagener, A. V. Singh, P. Laux, and A. Luch. 2019. Nanoparticle induced barrier function assessment at liquid–liquid and air–liquid interface in novel human lung epithelial cell lines. Toxicol. Res. (Camb.) 8 (6):1016–27. doi:10.1039/c9tx00179d.
- Lenz, A. G., E. Karg, E. Brendel, H. Hinze-Heyn, K. L. Maier, O. Eickelberg, T. Stoeger, and O. Schmid. 2013. Inflammatory and oxidative stress responses of an alveolar epithelial cell line to airborne zinc oxide nanoparticles at the air-liquid interface: A comparison with conventional, submerged cell-culture conditions. Biomed. Res. Int. 2013:652632. doi:10.1155/2013/652632.
- Lenz, A. G., E. Karg, B. Lentner, V. Dittrich, C. Brandenberger, B. Rothen-Rutishauser, H. Schulz, G. A. Ferron, and O. Schmid. 2009. A dose-controlled system for air-liquid interface cell exposure and application to zinc oxide nanoparticles. Part Fibre Toxicol. 6 (1):32. doi:10.1186/1743-8977-6-32.
- Li, N., S. Georas, N. Alexis, P. Fritz, T. Xia, M. A. Williams, E. Horner, and A. Nel. 2016. A work group report on ultrafine particles (American Academy of allergy, asthma & immunology): Why ambient ultrafine and engineered nanoparticles should receive special attention for possible adverse health outcomes in human subjects. J. Allergy Clin. Immunol. 138 (2):386–96. doi:10.1016/j.jaci.2016.02.023.
- Li, J. J., S. Muralikrishnan, C.-T. Ng, L.-Y. L. Yung, and B.-H. Bay. 2010. Nanoparticle-induced pulmonary toxicity. Exp. Biol. Med. 235 (9):1025–33. doi:10.1258/ebm.2010.010021.
- Liu, S., R. Yang, Y. Chen, X. Zhao, S. Chen, X. Yang, Z. Cheng, B. Hu, X. Liang, N. Yin, et al. 2021. Development of human lung induction models for air pollutants’ toxicity assessment. Environ. Sci. Technol. 55 (4):2440–51. doi:10.1021/acs.est.0c05700.
- Li, Y., Q. Wu, X. Sun, J. Shen, and H. Chen. 2020. Organoids as a powerful model for respiratory diseases. Stem Cells Int. 2020:5847876. doi:10.1155/2020/5847876.
- Londahl, J., W. Moller, J. H. Pagels, W. G. Kreyling, E. Swietlicki, and O. Schmid. 2014. Measurement techniques for respiratory tract deposition of airborne nanoparticles: A critical review. J. Aerosol. Med. Pulm. Drug Deliv. 27 (4):229–54. doi:10.1089/jamp.2013.1044.
- Loret, T., E. Peyret, M. Dubreuil, O. Aguerre-Chariol, C. Bressot, O. le Bihan, T. Amodeo, B. Trouiller, A. Braun, C. Egles, et al. 2016. Air-liquid interface exposure to aerosols of poorly soluble nanomaterials induces different biological activation levels compared to exposure to suspensions. Part Fibre Toxicol. 13 (1):58. doi:10.1186/s12989-016-0171-3.
- Loret, T., F. Rogerieux, B. Trouiller, A. Braun, C. Egles, and G. Lacroix. 2018. Predicting the in vivo pulmonary toxicity induced by acute exposure to poorly soluble nanomaterials by using advanced in vitro methods. Part Fibre Toxicol. 15 (1):25. doi:10.1186/s12989-018-0260-6.
- Madissoon, E., A. J. Oliver, V. Kleshchevnikov, A. Wilbrey-Clark, K. Polanski, A. R. Orsi, L. Mamanova, L. Bolt, N. Richoz, and R. Elmentaite. 2021. A spatial multi-omics atlas of the human lung reveals a novel immune cell survival niche. bioRxiv. doi:10.1101/2021.11.26.470108.
- Malakar, A., S. R. Kanel, C. Ray, D. D. Snow, and M. N. Nadagouda. 2021. Nanomaterials in the environment, human exposure pathway, and health effects: A review. Sci. Total Environ. 759:143470. doi:10.1016/j.scitotenv.2020.143470.
- Medina-Reyes, E. I., N. L. Delgado-Buenrostro, D. L. Leseman, A. Deciga-Alcaraz, R. He, E. R. Gremmer, P. H. B. Fokkens, J. O. Flores-Flores, F. R. Cassee, and Y. I. Chirino. 2020. Differences in cytotoxicity of lung epithelial cells exposed to titanium dioxide nanofibers and nanoparticles: Comparison of air-liquid interface and submerged cell cultures. Toxicol. In Vitro 65:104798. doi:10.1016/j.tiv.2020.104798.
- Meghani, N., K. H. Kim, S. H. Kim, S. H. Lee, and K. H. Choi. 2020. Evaluation and live monitoring of pH-responsive HSA-ZnO nanoparticles using a lung-on-a-chip model. Arch. Pharm. Res. 43 (5):503–13. doi:10.1007/s12272-020-01236-z.
- Meyerholz, D. K., C. J. Suarez, S. M. Dintzis, and C. W. Frevert. 2018. Respiratory system. In Comparative anatomy and histology, ed. P. M. Treuting, S. M. Dintzis, and K. S. Montine, 121–134. 2nd ed. San Diego: Academic Press. Chapter 9.
- Miller, A. J., D. R. Hill, M. S. Nagy, Y. Aoki, B. R. Dye, A. M. Chin, S. Huang, F. Zhu, E. S. White, V. Lama, et al. 2018. In vitro induction and in vivo engraftment of lung bud tip progenitor cells derived from human pluripotent stem cells. Stem Cell Rep. 10 (1):101–19. doi:10.1016/j.stemcr.2017.11.012.
- Miller, M. R., and C. A. Poland. 2020. Nanotoxicology: The need for a human touch. Small 16 (36):e2001516. doi:10.1002/smll.202001516.
- Monopoli, M. P., C. Aberg, A. Salvati, and K. A. Dawson. 2012. Biomolecular coronas provide the biological identity of nanosized materials. Nat. Nanotechnol 7 (12):779–86. doi:10.1038/nnano.2012.207.
- Monopoli, M. P., D. Walczyk, A. Campbell, G. Elia, I. Lynch, F. B. Bombelli, and K. A. Dawson. 2011. Physical−chemical aspects of protein corona: Relevance to in vitro and in vivo biological impacts of nanoparticles. J. Am. Chem. Soc. 133 (8):2525–34. doi:10.1021/ja107583h.
- Moore, T. L., L. Rodriguez-Lorenzo, V. Hirsch, S. Balog, D. Urban, C. Jud, B. Rothen-Rutishauser, M. Lattuada, and A. Petri-Fink. 2015. Nanoparticle colloidal stability in cell culture media and impact on cellular interactions. Chem. Soc. Rev. 44 (17):6287–305. doi:10.1039/C4CS00487F.
- Moore, T. L., D. A. Urban, L. Rodriguez-Lorenzo, A. Milosevic, F. Crippa, M. Spuch-Calvar, S. Balog, B. Rothen-Rutishauser, M. Lattuada, and A. Petri-Fink. 2019. Nanoparticle administration method in cell culture alters particle-cell interaction. Sci. Rep. 9 (1):900. doi:10.1038/s41598-018-36954-4.
- Morawska, L., and G. Buonanno. 2021. The physics of particle formation and deposition during breathing. Nat. Rev. Physics 3 (5):300–01. doi:10.1038/s42254-021-00307-4.
- Movia, D., S. Bruni-Favier, and A. Prina-Mello. 2020. In vitro alternatives to acute inhalation toxicity studies in animal models-A perspective. Front Bioeng. Biotechnol. 8:549. doi:10.3389/fbioe.2020.00549.
- Nahar, K., N. Gupta, R. Gauvin, S. Absar, B. Patel, V. Gupta, A. Khademhosseini, and F. Ahsan. 2013. In vitro, in vivo and ex vivo models for studying particle deposition and drug absorption of inhaled pharmaceuticals. Eur. J. Pharmaceut. Sci. 49 (5):805–18. doi:10.1016/j.ejps.2013.06.004.
- Nemmar, A., J. A. Holme, I. Rosas, P. E. Schwarze, and E. Alfaro-Moreno. 2013. Recent advances in particulate matter and nanoparticle toxicology: A review of the in vivo and in vitro studies. Biomed. Res. Int. 2013:279371. doi:10.1155/2013/279371.
- Nossa, R., J. Costa, L. Cacopardo, and A. Ahluwalia. 2021. Breathing in vitro: Designs and applications of engineered lung models. J. Tissue. Eng. 12:20417314211008696. doi:10.1177/20417314211008696.
- Oberdorster, G., V. Castranova, B. Asgharian, and P. Sayre. 2015. Inhalation exposure to carbon nanotubes (CNT) and carbon nanofibers (CNF): Methodology and dosimetry. J. Toxicol. Environ. Health B 18 (3–4):121–212. doi:10.1080/10937404.2015.1051611.
- Oberdörster, G., E. Oberdörster, and J. Oberdörster. 2005. Nanotoxicology: An emerging discipline evolving from studies of ultrafine particles. Environ. Health Perspect. 113 (7):823–39. doi:10.1289/ehp.7339.
- Panas, A., A. Comouth, H. Saathoff, T. Leisner, M. Al-Rawi, M. Simon, G. Seemann, O. Dossel, S. Mulhopt, H. R. Paur, et al. 2014. Silica nanoparticles are less toxic to human lung cells when deposited at the air-liquid interface compared to conventional submerged exposure. Beilstein J. Nanotechnol. 5:1590–602. doi:10.3762/bjnano.5.171.
- Paur, H.-R., F. R. Cassee, J. Teeguarden, H. Fissan, S. Diabate, M. Aufderheide, W. G. Kreyling, O. Hänninen, G. Kasper, and M. Riediker. 2011. In-vitro cell exposure studies for the assessment of nanoparticle toxicity in the lung—A dialog between aerosol science and biology. J. Aerosol. Sci. 42 (10):668–92. doi:10.1016/j.jaerosci.2011.06.005.
- Pavlovska, I., A. Ramata-Stunda, Z. Martinsone, M. Boroduskis, L. Patetko, I. Martinsone, A. Seile, and I. Vanadzins. 2021. In vitro impact preliminary assessment of airborne particulate from metalworking and woodworking industries. Sci. Rep. 11 (1):20181. doi:10.1038/s41598-021-99815-7.
- Penn-CenturyTM. 2021. Penn-CenturyTM - Exclusive manufacturer of the MicroSprayer® Aerosolizer. Accessed 2021. https://penncentury.com/products/
- Pezzulo, A. A., T. D. Starner, T. E. Scheetz, G. L. Traver, A. E. Tilley, B.-G. Harvey, R. G. Crystal, P. B. McCray Jr, and J. Zabner. 2011. The air-liquid interface and use of primary cell cultures are important to recapitulate the transcriptional profile of in vivo airway epithelia. Am. J. Physiol. Lung Cell Mol. Physiol. 300 (1):L25–31. doi:10.1152/ajplung.00256.2010.
- Pfuhler, S., J. van Benthem, R. Curren, S. H. Doak, M. Dusinska, M. Hayashi, R. H. Heflich, D. Kidd, D. Kirkland, Y. Luan, et al. 2020. Use of in vitro 3D tissue models in genotoxicity testing: Strategic fit, validation status and way forward. Report of the working group from the 7th International Workshop on Genotoxicity Testing (IWGT). Mutat. Res. Genet. Toxicol. Environ. Mutagen. 850-851:503135. doi:10.1016/j.mrgentox.2020.503135.
- Pietroiusti, A., H. Stockmann-Juvala, F. Lucaroni, and K. Savolainen. 2018. Nanomaterial exposure, toxicity, and impact on human health. Wiley Interdiscip. Rev. Nanomed. Nanobiotechnol. 10 (5). doi:10.1002/wnan.1513.
- Polk, W. W., M. Sharma, C. M. Sayes, J. A. Hotchkiss, and A. J. Clippinger. 2016. Aerosol generation and characterization of multi-walled carbon nanotubes exposed to cells cultured at the air-liquid interface. Part Fibre Toxicol. 13 (1):20. doi:10.1186/s12989-016-0131-y.
- Prasad, M., R. Kumar, L. Buragohain, A. Kumari, and M. Ghosh. 2021. Organoid technology: A reliable developmental biology tool for organ-specific nanotoxicity evaluation. Front Cell Dev. Biol. 9:696668. doi:10.3389/fcell.2021.696668.
- Prytherch, Z., and K. Bérubé. 2015. 3-dimensional cell and tissue culture models of the human respiratory system: Approaches for in vitro safety testing and drug discovery. doi:10.1371/journal.pone.0126536.
- Prytherch, Z., C. Job, H. Marshall, V. Oreffo, M. Foster, and K. Bérubé. 2011. Tissue-specific stem cell differentiation in an in vitro airway model. Macromol. Biosci. 11:1467–77. doi:10.1002/mabi.201100181.
- Punde, T. H., W. H. Wu, P. C. Lien, Y. L. Chang, P. H. Kuo, M. D. Chang, K. Y. Lee, C. D. Huang, H. P. Kuo, Y. F. Chan, et al. 2015. A biologically inspired lung-on-a-chip device for the study of protein-induced lung inflammation. Integr. Biol. (Camb.) 7 (2):162–69. doi:10.1039/c4ib00239c.
- Rackley, C. R., and B. R. Stripp. 2012. Building and maintaining the epithelium of the lung. J. Clin. Invest. 122 (8):2724–30. doi:10.1172/JCI60519.
- Raesch, S. S., S. Tenzer, W. Storck, A. Rurainski, D. Selzer, C. A. Ruge, J. Perez-Gil, U. F. Schaefer, and C. M. Lehr. 2015. Proteomic and lipidomic analysis of nanoparticle corona upon contact with lung surfactant reveals differences in protein, but not lipid composition. ACS Nano. 9 (12):11872–85. doi:10.1021/acsnano.5b04215.
- Rahimi, R., S. S. Htwe, M. Ochoa, A. Donaldson, M. Zieger, R. Sood, A. Tamayol, A. Khademhosseini, A. M. Ghaemmaghami, and B. Ziaie. 2016. A paper-based in vitro model for on-chip investigation of the human respiratory system. Lab. Chip. 16 (22):4319–25. doi:10.1039/C6LC00866F.
- Rosario, F., P. Hoet, A. J. A. Nogueira, C. Santos, and H. Oliveira. 2018. Differential pulmonary in vitro toxicity of two small-sized polyvinylpyrrolidone-coated silver nanoparticles. J. Toxicol. Environ. Health Part A 81 (15):675–90. doi:10.1080/15287394.2018.1468837.
- Rothen-Rutishauser, B., F. Blank, C. Mühlfeld, and P. Gehr. 2008. In vitro models of the human epithelial airway barrier to study the toxic potential of particulate matter. Expert Opin. Drug Metab. Toxicol. 4 (8):1075–89. doi:10.1517/17425255.4.8.1075.
- Sakolish, C. M., M. B. Esch, J. J. Hickman, M. L. Shuler, and G. J. Mahler. 2016. Modeling barrier tissues in vitro: Methods, achievements, and challenges. EBioMed 5:30–39. doi:10.1016/j.ebiom.2016.02.023.
- Sambale, F., A. Lavrentieva, F. Stahl, C. Blume, M. Stiesch, C. Kasper, D. Bahnemann, and T. Scheper. 2015. Three dimensional spheroid cell culture for nanoparticle safety testing. J. Biotechnol. 205:120–29. doi:10.1016/j.jbiotec.2015.01.001.
- Savolainen, K., H. Alenius, H. Norppa, L. Pylkkänen, T. Tuomi, and G. Kasper. 2010. Risk assessment of engineered nanomaterials and nanotechnologies—A review. Toxicology 269 (2–3):92–104. doi:10.1016/j.tox.2010.01.013.
- Schlinkert, P., E. Casals, M. Boyles, U. Tischler, E. Hornig, N. Tran, J. Zhao, M. Himly, M. Riediker, G. J. Oostingh, et al. 2015. The oxidative potential of differently charged silver and gold nanoparticles on the three human lung epithelial cell types. J. Nanobiotechnol. 13 (1):1. doi:10.1186/s12951-014-0062-4.
- Schmid, O., and F. R. Cassee. 2017. On the pivotal role of dose for particle toxicology and risk assessment: Exposure is a poor surrogate for delivered dose. Part Fibre Toxicol. 14 (1):52. doi:10.1186/s12989-017-0233-1.
- Schulz, H., V. Harder, A. Ibald-Mulli, A. Khandoga, W. Koenig, F. Krombach, R. Radykewicz, A. Stampfl, B. Thorand, and A. Peters. 2005. Cardiovascular effects of fine and ultrafine particles. J. Aerosol. Med. 18 (1):1–22. doi:10.1089/jam.2005.18.1.
- Schuster, B. S., J. S. Suk, G. F. Woodworth, and J. Hanes. 2013. Nanoparticle diffusion in respiratory mucus from humans without lung disease. Biomaterials 34 (13):3439–46. doi:10.1016/j.biomaterials.2013.01.064.
- Secondo, L. E., N. J. Liu, and N. A. Lewinski. 2017. Methodological considerations when conducting in vitro air–liquid interface exposures to engineered nanoparticle aerosols. Crit. Rev. Toxicol. 47 (3):225–62. doi:10.1080/10408444.2016.1223015.
- Seiffert, J., A. Buckley, B. Leo, N. G. Martin, J. Zhu, R. Dai, F. Hussain, C. Guo, J. Warren, A. Hodgson, et al. 2016. Pulmonary effects of inhalation of spark-generated silver nanoparticles in Brown-Norway and Sprague–Dawley rats. Respir. Res. 17 (1):85. doi:10.1186/s12931-016-0407-7.
- Shannahan, J. H., U. P. Kodavanti, and J. M. Brown. 2012. Manufactured and airborne nanoparticle cardiopulmonary interactions: A review of mechanisms and the possible contribution of mast cells. Inhal Toxicol. 24 (5):320–39. doi:10.3109/08958378.2012.668229.
- Smith, J. R., A. Birchall, G. Etherington, N. Ishigure, and M. R. Bailey. 2014. A revised model for the deposition and clearance of inhaled particles in human extra-thoracic airways. Radiat. Prot. Dosimetry 158 (2):135–47. doi:10.1093/rpd/nct218.
- Smulders, S., K. Luyts, G. Brabants, L. Golanski, J. Martens, J. Vanoirbeek, and P. H. Hoet. 2015. Toxicity of nanoparticles embedded in paints compared to pristine nanoparticles, in vitro study. Toxicol. Lett. 232 (2):333–39. doi:10.1016/j.toxlet.2014.11.030.
- Snyder-Talkington, B. N., Y. Qian, V. Castranova, and N. L. Guo. 2012. New perspectives for in vitro risk assessment of multiwalled carbon nanotubes: Application of coculture and bioinformatics. J. Toxicol. Environ. Health B 15 (7):468–92. doi:10.1080/10937404.2012.736856.
- Sotty, J., G. Garcon, F.-O. Denayer, L.-Y. Alleman, Y. Saleh, E. Perdix, V. Riffault, P. Dubot, J.-M. Lo-Guidice, and L. Canivet. 2019. Toxicological effects of ambient (PM2.5-018) and ultrafine) particles in healthy and diseased 3D organo-typic mucociliary-phenotype models. Environ. Res. 176:108538. doi:10.1016/j.envres.2019.108538.
- Sreedharan, S., G. Zouganelis, S. J. Drake, G. Tripathi, and A. Kermanizadeh. 2022. Nanomaterial-induced toxicity in pathophysiological models of representative individuals with pre-existing medical conditions. J. Toxicol. Environ. Health B 1–27. doi:10.1080/10937404.2022.2153456.
- Steimer, A., E. Haltner, and C.-M. Lehr. 2005. Cell culture models of the respiratory tract relevant to pulmonary drug delivery. J. Aerosol. Med. 18 (2):137–82. doi:10.1089/jam.2005.18.137.
- Stone, V., M. R. Miller, M. J. D. Clift, A. Elder, N. L. Mills, P. Moller, R. P. F. Schins, U. Vogel, W. G. Kreyling, K. Alstrup Jensen, et al. 2017. Nanomaterials versus ambient ultrafine particles: An opportunity to exchange toxicology knowledge. Environ. Health Perspect. 125 (10):106002. doi:10.1289/EHP424.
- Stucki, A. O., J. D. Stucki, S. R. Hall, M. Felder, Y. Mermoud, R. A. Schmid, T. Geiser, and O. T. Guenat. 2015. A lung-on-a-chip array with an integrated bio-inspired respiration mechanism. Lab. Chip. 15 (5):1302–10. doi:10.1039/C4LC01252F.
- Sun, T., Y. Kang, J. Liu, Y. Zhang, L. Ou, X. Liu, R. Lai, and L. Shao. 2021. Nanomaterials and hepatic disease: Toxicokinetics, disease types, liver susceptibility and influencing factors. J. Nanobiotechnol. 19 (1):108. doi:10.1186/s12951-021-00843-2.
- Tan, Q., K. M. Choi, D. Sicard, and D. J. Tschumperlin. 2017. Human airway organoid engineering as a step toward lung regeneration and disease modeling. Biomaterials 113:118–32. doi:10.1016/j.biomaterials.2016.10.046.
- Teeguarden, J. G., P. M. Hinderliter, G. Orr, B. D. Thrall, and J. G. Pounds. 2007. Particokinetics in vitro: Dosimetry considerations for in vitro nanoparticle toxicity assessments. Toxicol. Sci. 95 (2):300–12. doi:10.1093/toxsci/kfl165.
- Thiruvengadam, M., G. Rajakumar, and I. M. Chung. 2018. Nanotechnology: Current uses and future applications in the food industry. Biotechnology 8 (1):74. doi:10.1007/s13205-018-1104-7.
- Thomas, R. J. 2013. Particle size and pathogenicity in the respiratory tract. Virulence 4 (8):847–58. doi:10.4161/viru.27172.
- Thomas, D. G., J. N. Smith, B. D. Thrall, D. R. Baer, H. Jolley, P. Munusamy, V. Kodali, P. Demokritou, J. Cohen, and J. G. Teeguarden. 2018. ISD3: A particokinetic model for predicting the combined effects of particle sedimentation, diffusion and dissolution on cellular dosimetry for in vitro systems. Part Fibre Toxicol. 15 (1):6. doi:10.1186/s12989-018-0243-7.
- Tippe, A., U. Heinzmann, and C. Roth. 2002. Deposition of fine and ultrafine aerosol particles during exposure at the air/cell interface. J. Aerosol. Sci. 33 (2):207–18. doi:10.1016/S0021-8502(01)00158-6.
- Travaglini, K. J., A. N. Nabhan, L. Penland, R. Sinha, A. Gillich, R. V. Sit, S. Chang, S. D. Conley, Y. Mori, J. Seita, et al. 2020. A molecular cell atlas of the human lung from single-cell RNA sequencing. Nature 587 (7835):619–25. doi:10.1038/s41586-020-2922-4.
- Tsuda, A., F. S. Henry, and J. P. Butler. 2013. Particle transport and deposition: Basic physics of particle kinetics. Compr. Physiol. 3:1437–71.
- Upadhyay, S., and L. Palmberg. 2018. Air-liquid interface: Relevant in vitro models for investigating air pollutant-induced pulmonary toxicity. Toxicol. Sci. 164 (1):21–30. doi:10.1093/toxsci/kfy053.
- Urban, D. A., L. Rodriguez-Lorenzo, S. Balog, C. Kinnear, B. Rothen-Rutishauser, and A. Petri-Fink. 2016. Plasmonic nanoparticles and their characterization in physiological fluids. Colloids Surf. B Biointerfaces 137:39–49. doi:10.1016/j.colsurfb.2015.05.053.
- Vis, M. A. M., K. Ito, and S. Hofmann. 2020. Impact of culture medium on cellular interactions in in vitro co-culture systems. Front Bioeng. Biotechnol. 8. doi:10.3389/fbioe.2020.00911.
- Wang, Y., A. Adamcakova-Dodd, B. R. Steines, X. Jing, A. K. Salem, and P. S. Thorne. 2020. Comparison of in vitro toxicity of aerosolized engineered nanomaterials using air-liquid interface mono-culture and co-culture models. NanoImpact 18:100215. doi:10.1016/j.impact.2020.100215.
- Wang, G., X. Zhang, X. Liu, J. Zheng, R. Chen, and H. Kan. 2019. Ambient fine particulate matter induce toxicity in lung epithelial-endothelial co-culture models. Toxicol. Lett. 301:133–45. doi:10.1016/j.toxlet.2018.11.010.
- Weibel, E. R., B. Sapoval, and M. Filoche. 2005. Design of peripheral airways for efficient gas exchange. Respir. Physiol. Neurobiol. 148 (1–2):3–21. doi:10.1016/j.resp.2005.03.005.
- Whitsett, J. A., and T. Alenghat. 2015. Respiratory epithelial cells orchestrate pulmonary innate immunity. Nat. Immunol. 16 (1):27–35. doi:10.1038/ni.3045.
- Wick, P., S. Chortarea, O. T. Guenat, M. Roesslein, J. D. Stucki, S. Hirn, A. Petri-Fink, and B. Rothen-Rutishauser. 2015. In vitro-ex vivo model systems for nanosafety assessment. Eur. J. Nanomed. 7 (3):169–79. doi:10.1515/ejnm-2014-0049.
- Wiemann, M., A. Vennemann, U. G. Sauer, K. Wiench, L. Ma-Hock, and R. Landsiedel. 2016. An in vitro alveolar macrophage assay for predicting the short-term inhalation toxicity of nanomaterials. J. Nanobiotechnol. 14 (1):16. doi:10.1186/s12951-016-0164-2.
- Wilkinson, D. C., J. A. Alva-Ornelas, J. M. S. Sucre, P. Vijayaraj, A. Durra, W. Richardson, S. J. Jonas, M. K. Paul, S. Karumbayaram, B. Dunn, et al. 2016. Development of a three-dimensional bioengineering technology to generate lung tissue for personalized disease modeling. Stem Cells Transl. Med. 6 (2):622–33. doi:10.5966/sctm.2016-0192.
- Wohlleben, W., M. D. Driessen, S. Raesch, U. F. Schaefer, C. Schulze, B. Vacano, A. Vennemann, M. Wiemann, C. A. Ruge, H. Platsch, et al. 2016. Influence of agglomeration and specific lung lining lipid/protein interaction on short-term inhalation toxicity. Nanotoxicology 10 (7):970–80. doi:10.3109/17435390.2016.1155671.
- Yamamoto, Y., S. Gotoh, Y. Korogi, M. Seki, S. Konishi, S. Ikeo, N. Sone, T. Nagasaki, H. Matsumoto, S. Muro, et al. 2017. Long-term expansion of alveolar stem cells derived from human iPS cells in organoids. Nat. Meth. 14 (11):1097–106. doi:10.1038/nmeth.4448.
- Yang, X., K. Li, X. Zhang, C. Liu, B. Guo, W. Wen, and X. Gao. 2018. Nanofiber membrane supported lung-on-a-chip microdevice for anti-cancer drug testing. Lab. Chip. 18 (3):486–95. doi:10.1039/C7LC01224A.
- Zacharias, W. J., D. B. Frank, J. A. Zepp, M. P. Morley, F. A. Alkhaleel, J. Kong, S. Zhou, E. Cantu, and E. E. Morrisey. 2018. Regeneration of the lung alveolus by an evolutionarily conserved epithelial progenitor. Nature 555 (7695):251–55. doi:10.1038/nature25786.
- Zanoni, M., M. Cortesi, A. Zamagni, C. Arienti, S. Pignatta, and A. Tesei. 2020. Modeling neoplastic disease with spheroids and organoids. J. Hematol. Oncol. 13 (1):97. doi:10.1186/s13045-020-00931-0.
- Zhang, F., G. V. Aquino, A. Dabi, and E. D. Bruce. 2019. Assessing the translocation of silver nanoparticles using an in vitro co-culture model of human airway barrier. Toxicol. In Vitro 56:1–9. doi:10.1016/j.tiv.2018.12.013.
- Zhang, M., C. Xu, L. Jiang, and J. Qin. 2018. A 3D human lung-on-a-chip model for nanotoxicity testing. Toxicol. Res. 7 (6):1048–60. doi:10.1039/C8TX00156A.
- Zhao, J., and V. Castranova. 2011. Toxicology of nanomaterials used in nanomedicince. J. Toxicol. Environ. Health B 14 (8):593–632. doi:10.1080/10937404.2011.615113.