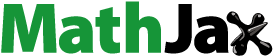
ABSTRACT
Wild boar populations have increased worldwide, but the consequences of their disturbances on boreal forest ecosystems are largely unknown. We investigated how wild boars affect soil processes in a Swedish boreal forest. We estimated effects on ecosystem functioning using phospholipid fatty acid analyses (PLFA) to characterise microbial groups, and by measuring soil respiration, soil carbon (C), nitrogen (N) and phosphorus (P) concentrations, as well as the availability of NO3− and NH4+. We compared samples collected inside wild boar enclosures with adjacent reference areas without wild boar disturbance. We found no difference in soil microbial composition, except for a consistently higher fungi:bacteria ratio in the enclosures. These results are contrary to our hypothesis that rooting raises nitrogen levels, which in turn result in more bacteria. Soil nutrient levels showed inconsistent patterns, suggesting that substrate changes – as opposed to nutrient changes – stimulated fungal growth. Soil respiration was lower in the enclosures, contradicting earlier findings suggesting increased soil CO2 emissions from wild boar rooting. Overall, our study suggests that increased wild boar abundance has a minor impact on soil processes in boreal forests. Future studies should determine if the modest impacts remain across time and boreal forests.
RÉSUMÉ
Les populations de sanglier ont augmenté à l’échelle planétaire, mais les conséquences de leurs perturbations sur les écosystèmes forestiers boréaux sont peu connues. Nous avons évalué les effets des sangliers sur les processus du sol dans une forêt boréale en Suède. Nous avons estimé les effets sur le fonctionnement écosystémique en utilisant des analyses d’acides gras phospholipidiques (AGPL) pour caractériser les groupes microbiens, et en mesurant la respiration, le carbone (C), l’azote (N) et le phosphore (P) du sol, ainsi que la disponibilité en NO3− et NH4+. Nous avons comparé des échantillons prélevés dans des enclos à sangliers et dans des sites témoins non perturbés. Nous n’avons trouvé aucune différence de composition microbienne du sol, sauf un rapport champignons/bactéries systématiquement plus élevé dans les enclos. Ces résultats vont à l’encontre de notre hypothèse selon laquelle la fouille du sol par les sangliers augmenterait la concentration en azote, et par conséquent la quantité de bactéries. Les patrons irréguliers des concentrations en nutriments du sol suggèrent que ce sont des changements de substrat – et non des changements de nutriments – qui stimulent la croissance fongique. La respiration du sol était plus faible dans les enclos, contrairement aux résultats d’études précédentes suggérant que la fouille du sol par les sangliers augmenterait les émissions de CO2. Globalement, notre étude suggère que l’augmentation d’abondance du sanglier a un effet mineur sur les processus du sol en forêt boréale. Des études supplémentaires sont requises afin de déterminer si ces effets modestes sont persistants et s’ils sont observés dans d’autres forêts boréales.
Introduction
Large animals can greatly alter ecosystem processes, even to the point of causing complete shifts in habitat type, e.g., from forest to grassland (Vázguez Citation2002). Wild boars (Sus scrofa), for example, are readily re-colonizing new environments, and populations are increasing in many parts of the world (Jensen Citation2017). The ways that large animals affect ecosystem functioning vary greatly, and depend both on type and density of animal, and may vary even within a specific ecosystem (Kolstad et al. Citation2018; Leroux et al. Citation2020). Low fertility ecosystems, such as boreal forests, are particularly sensitive to disturbances by animals due to their potential impact on soil microbial activity and nitrogen (N) mineralization, which in turn can aggravate the N limitation of these ecosystems (Leroux et al. Citation2020). Although the ecosystem effects of many herbivorous species are fairly well known (Bernes et al. Citation2018), the effects of wild boars in northern climates (hemiboreal, boreal) are less known due to the recent increase of their northern range edge (Gray et al. Citation2020).
In Sweden, wild boars were reintroduced through escapes and releases from enclosures in the 1970s after being extirpated for several centuries. (Swedish Environmental Protection Agency Citation2019). Since their reintroduction, the wild boar population has increased from a few individuals to an estimated population of 300 000 in 2019, and now wild boars inhabit more than half of Sweden (Svenska jägareförbundet Citation2018). Similar to many large herbivores and omnivores, wild boars increase nutrient availability through urine and faeces, and change the species composition through selective and intense foraging that can benefit less competitive species (Mack and D’Antonio Citation1998; Liu et al. Citation2015). However, due to their rooting behaviour, the wild boar effects on ecosystem functioning will likely be different from those inflicted by other ungulates. The rooting behaviour has the potential to cause great changes to the landscape they inhabit by reducing vegetation cover and changing soil chemistry (Gray et al. Citation2020). Consequently, expanding wild boar populations are considered problematic in many parts of the world (Barrios-Garcia and Ballari Citation2012). To date, however, we have little quantitative data on the degree to which the rapid expansion of wild boars in boreal regions is affecting ecosystem functions (Maaroufi et al. Citation2022).
Soil microbial activity is tightly linked to extracting and transforming organic compounds into plant-available nutrients, which will affect soil carbon (C) and N stocks (Clemmensen et al. Citation2013). Changes in biomass or composition of microbial communities may thus alter ecosystem functions related to C and N dynamics (Bardgett and Wardle Citation2010). Mechanical soil disturbances, such as tilling, are known to alter both the biomass and composition of microbial communities, and reduced fungal biomass and increased biomass of bacteria, either in absolute or relative terms, appear to be common responses to physical disturbance (e.g., Jackson et al. Citation2003; Allison et al. Citation2005). Further, mycorrhizal forming fungi appear to be more sensitive to physical disturbance than saprophytic fungi (Allison et al. Citation2005). Although mechanical disturbance like tilling differs from rooting by wild boars, it seems likely that the bioturbation (i.e., soil mixing) that the rooting imposes can have important implications for microbial biomass and community composition. In addition, the bioturbation that wild boars generate can increase litter decomposition and enhance mineralization rates (Gutiérrez and Jones Citation2006), and could thereby potentially influence soil processes such as C and N turnover. The expected higher levels of available nutrients can increase the productivity of the system and, for example, may promote tree growth in N limited systems such as boreal forests (Sullivan et al. Citation2015).
The knowledge of wild boars’ effect on soil processes stems mostly from studies conducted in temperate forests or deserts (Lacki and Lancia Citation1986; Cuevas et al. Citation2012; Liu et al. Citation2020), while studies from northern ecosystems such as boreal forests are lacking. Previous studies on wild boar presence have found mixed results on various forest soil and plant responses (Lacki and Lancia Citation1986; Cuevas et al. Citation2012; Liu et al. Citation2020). As boreal forest soils are characterised by low bioturbation and have an organic topsoil layer that is important for nutrient cycling and carbon sequestration (Perry et al. Citation2008), it is likely that the effects of wild boars’ rooting differ in these ecosystems from those previously studied. In Argentina, litter decomposition and soil bulk densities were lower in rooted areas compared to unrooted areas, while there was no difference in nutrient cycling or soil nutrient stocks (Cuevas et al. Citation2012). Studies in deciduous forests in Switzerland reported higher C and N concentrations (but lower plant available N), higher microbial biomass and a higher soil respiration rate in rooted than in unrooted areas (Risch et al. Citation2010; Wirthner et al. Citation2012). Recent estimates suggest that rooting from wild boars in areas outside their native distribution range generates CO2 emissions of global concern (O’Bryan et al. Citation2021). These estimates have, however, been questioned (Don Citation2022), and more data on the potential effects that wild boars may have on C dynamics and productivity is needed.
Our objective was to estimate how wild boar may impact ecosystem functioning by investigating how wild boar influence C and nutrient dynamics in spruce and pine-dominated boreal forests. Specifically, we wanted to address whether wild boars (i) alter soil microbial composition, nutrient dynamics, and/or C and N stocks, and (ii) promote soil respiration. We expected that wild boar disturbance would shift the microbial composition towards a lower fungi:bacteria ratio leading to faster organic soil turnover with higher levels of available nutrients, higher soil respiration, and less soil carbon.
Methods
Study area and experimental design
Our study areas consist of two sampling areas within wild boar enclosures (hereafter referred to as enclosures A and B) and two reference areas (adjacent to the enclosures) in south-east Sweden (N 59.856; E 18.091) (). The area has a mean annual temperature of ~ 5°C, ranging from a monthly average of -4°C in February to 15°C in July, and a mean annual precipitation of ~600 mm (SMHI Citation2009). The soil consists of sandy moraine and glacial clay.
Figure 1. Map of the study area with wild boar enclosures (filled circles) and reference areas (crossed circles) in a pine forest (green) and spruce forest (blue). Black lines with white dots indicate the fences. A minor gravel road divides the pine forest enclosure from the pine reference area.

We used a paired plot setup with two sampling areas within wild boar enclosures (a Pinus sylvestris and a Picea abies forest, hereafter pine and spruce forest, respectively, as these are the predominant tree species in boreal Fennoscandia), paired with two reference areas, making a total of four sample areas. The two forests were established by planting after logging and are homogenous and even-aged (pine ~32 years; spruce ~40 years). The enclosures – each with two wild boars – were established in 2012. During a few periods not longer than six months, the enclosures had no wild boar. Although wild boars in the enclosures receive some supplemental feeding, it has little effect on rooting because it is a natural part of their exploratory behaviour (Jensen Citation2017). In each of the sampling areas, we established five 10 × 10 m sampling plots. To minimise the effect of site differences between enclosures and references, we selected reference areas that were similar to the enclosures in terms of tree species composition, tree age, and tree stem density and diameter but without signs of rooting by wild boar. Enclosure A has an area of 1.6 ha and is in a forest dominated by Scots pine with a few subdominant deciduous trees and an understory vegetation dominated by Vaccinium spp., ferns and grasses. The mean (± standard deviation) stem diameter was 21 ± 3.3 cm. Four of our sampling plots were located in enclosure A and a fifth sampling plot was located in an adjacent 2.5 ha enclosure within the same pine stand. The adjacent enclosure also had two wild boars since 2012 and was included due to its similarities with the reference area. The corresponding reference stand adjacent to enclosure A, only divided by a road, was also dominated by Scots pine with an understory dominated by Vaccinium spp., ferns and grasses. The mean stem diameter was 20 ± 1.3 cm. Enclosure B has an area of 2.3 ha and is located in a spruce-dominated forest with the understory consisting mainly of mosses, scattered ferns, and a few herbaceous plants. The mean stem diameter was 26 ± 0.7 cm. The enclosure fence cut through the spruce forest and we used the area just outside the fence as a reference, where there was an intact moss layer with sparse ferns, grasses, and herbaceous plants. The understory vegetation in both enclosures was heavily reduced due to the wild boar disturbance, and the soil was, in most places, bare or had only a very sparse vegetation cover. While natural wild boar densities are difficult to quantify (Swedish Environmental Protection Agency Citation2019), the densities in these enclosures are higher than what can be expected (1.25 and 0.87 wild boars ha−1 in Enclosure A and Enclosure B, respectively).
Soil samples
On the 21st and 22nd of September 2020, we collected 25 soil samples in each plot using a soil borer with a diameter of 3 cm. Sampling locations within the plot were semi-randomly chosen by blindfolded throwing of a plastic stick, while at the same time ensuring that the whole plot area was sampled. The minimum distance between two sampling points was set to 0.5 m and we never collected samples closer than 0.5 m to a tree. Due to the clear stratification of boreal forest soils, and the fact that different soil horizons differ in nutrient and microbial activity (Tamm Citation1991; Berg and McClaugherty Citation2014), the soil samples were separated into two layers during sampling. These layers were sampled to represent the topsoil (0–2 cm) and lower soil (2–5 cm). Soil mixing caused by bild boar rooting eliminated the soil horizons in the enclosures, making it challenging to compare soil characteristics such as C stock between reference sites and rooted sites. There is no easy way to compare soils with different layering (Carter et al. Citation2007), and we used a fixed depth approach. This method restricts interpretation to the upper portion of the soil profile, where greatest impact of wild boar rooting is expected. After collection, all topsoil and lower soil samples of each plot were pooled, resulting in one topsoil and one lower soil layer for each plot, in both the reference and enclosure areas. The samples were placed in sealed plastic bags and brought back to the lab, where we homogenised each sample independently. Before further analysis, the samples were freeze dried, sifted through a 5 mm mesh to remove larger objects, and then ground in a ball mill (Retsch PM 100 CM). The milled samples were weighed for dry bulk density and used to analyse proportion organic matter, total C, N, and phosphorus (P), as well as the microbial groups.
Phospholipid fatty acid analysis
We used phospholipid fatty acid analysis (PLFA) to examine differences in microbial community composition and to estimate the abundance of bacteria and fungi (Yao et al. Citation2000; modified from Frostegård et al. Citation1991). Fatty acids were extracted from 3 g of the dried soil samples and analysed on an MS-GC (Agilent 5977A MSD). The data was analysed using MSD Chem Station for the relative concentrations of phospholipids. The amounts of PLFAs were expressed as µmol per gram dry soil. The PLFAs representing bacteria used in this study were pentadecanoate (15:0, i15:0, a15:0), hexadecanoate (i16:0, 16:1 ω9), heptadecanoate (17:0, i17:0, cy17:0), and nonadecanoate (cy19:0), and the PLFA representing fungi was octadecenoate (18:2 ω6). Besides these, the following general PLFAs not representing either bacteria or fungi were tetradecanoate (14:0), hexadecanoate (16:0) and octadecenoate (18:0). Due to inconsistencies in identifying PLFA 16:1 ω5 as either fungi or bacteria, this too was identified as a general PLFA (Frostegård and Bååth Citation1996; Yao et al. Citation2000; Bååth and Andersson Citation2003).
Soil respiration rate
In 2020, we measured soil respiration between 9 am and 4 pm during two consecutive days (one reference and enclosure per day) each month between June and September, and once again in November. We determined five semi-random sampling points by throwing PVC-collars in all directions of the plot and sampling where the collar landed within each plot. Whenever a sampling position ended up on a rock, a previously sampled point, or where they were closer than 1 m to a tree, sampling points were adjusted. Where vegetation was present, the living vegetation was removed before placing the PVC-collar (diameter = 30 cm; height = 14.5 cm; internal volume = 10.2 dm3). The PVC-collar was carefully inserted circa 1 cm into the soil to ensure an airtight seal between the soil and the collar. To measure the CO2 flux within the chamber, we placed a lid equipped with a fan, a Vaisala GM70 CO2 probe and a Vaisala HM70 humidity probe on the collar. The CO2 flux within the chamber was measured every fifteen seconds for three minutes. After three minutes, we measured the soil temperature and moisture using a lab thermometer and a soil moisture probe (Meter TEROS 12), respectively. Soil respiration rate (CO2 flux) was expressed as μmol per hour and m2.
Organic matter content
Organic matter concentration was measured through loss on ignition (Hoogsteen et al. Citation2015). From each soil sample, approximately 15 g of dried soil was placed in a Nabertherm muffle furnace at 550°C for 4 hours. Organic matter concentration was calculated as the weight lost divided by the initial weight.
Total C, N, and P
To measure C and N concentrations, we used circa 15 mg of the dried and homogenised soil samples and analysed the samples in a Costech elemental combustion system 4010. Phosphorus concentrations were measured using absorbance measurements of extracted soil total P (Andersen Citation1976). For each plot, we calculated C, N and P stocks (g m−2) for the top 5 cm of the soil, using element concentrations and bulk density for the two sampled soil layers. This measure includes the top layer (2 cm) and 3 cm of the lower layer. We chose 5 cm as this was the minimum sample depth used in our study.
Available nitrogen
To measure the relative differences in N availability between plots, we used 10 cm2 resin membranes. The membranes were shaken at 40 rpm in a 0.5 M HCl solution for one hour and a 0.5 M NaHCO3 solution for five hours, with the solution renewed every hour (https://lter.kbs.msu.edu/protocols/105; Qian and Schoenau Citation2005). The membranes were kept moist, and on August 28, they were buried a few centimetres under the soil surface at a 45° angle. For each plot we buried five anion and five cation membranes. The membranes were retrieved from the field on the 21st and 22nd of September. We were able to relocate most of the membranes (87%), but 26 resin membranes (18 in the reference areas and 8 in the enclosures) were not relocated. After collection, the membranes were brought back to the lab where they, after removing attached soil particles with deionized water, were shaken at 40 rpm in 175 ml of a 2 M KCl solution for one hour. The NO3− concentration of the solution was measured using a Metrohm 883 Basic IC plus. To obtain the NH4+ concentration, the extract from the cation membranes was treated according to Kempers and Zweers (Citation2008) and then analysed using a Perkin Elmer spectrometer. To receive the resin-available concentrations of both NO3− and NH4+, the following formula was used:
Where C = the anions/cations (µg ml−1) of ion chromatograph and absorbance measurements; D = the dilution level of samples for the ion chromatograph; KCl = the amount of potassium chloride (ml) used to extract anions/cations from resin membranes; A = the initial area (cm2) of resins placed in the ground; R = the remaining area (%) of resins after extraction from the ground; and T = the time (days) that the resins stayed in the ground.
Statistical analyses
We treated each sampling plot as an independent sample (i.e., five replicates and N = 20). A more conservative interpretation would be that we only have two replicates (two pairs, i.e., N = 4), and for each forest type (environment), only one replicate. We acknowledge that our design is limited if we want to generalise our results to a landscape level, but here we limit our evaluation to the investigated area. Landscape manipulation in ecology is challenging, and even if the statistical evaluation often needs to be restricted in space, we believe that the ecological interpretation is valuable (see Davies and Gray (Citation2015) for a discussion on pseudoreplication in landscape ecology). We argue that samples within the enclosures are independent, i.e., there is no spatial autocorrelation. To test if the spatial distance between plots influenced the results, we created a distance matrix using the spatial distance in metres between every pair of plots using the QGIS software and the Distance Matrix tool (QGIS Development Team Citation2020). A distance matrix was then created using the differences in the data regarding response variables between every pair of plots. No differences in the measured variables showed correlations with spatial distances.
We tested the effects of wild boar enclosures, environment (dominating tree species), and soil layer (when the response was sampled at two layers) on each of our response variables (soil density, organic matter, nutrient concentration, and stocks) with linear models. If soil layer was included as an explanatory variable, we used the R package lme4 (Bates et al. Citation2015) to fit linear mixed-models (LMMs) with plot as a random factor to account for the within-plot dependence between top and lower soil depth. Variance homogeneity and normality of residuals were checked visually. Models were evaluated with ANOVA (Type 2 test) using either the R package car (Fox and Weisberg Citation2019) or the lmerTest package (Kuznetsova et al. Citation2017) for LMMs.
To test whether the PLFA composition differed between areas and soil layers, including potential interaction effects, we used the R package vegan (Oksanen et al. Citation2020) to perform a multivariate analysis (PERMANOVA, adonis2 function). In this analysis, we used Euclidean distances, where the PLFA matrix was the response and enclosure treatment (enclosure, reference), environment (pine, spruce forest), and soil depth (top, lower layer) were the explanatory variables. In addition, we fitted models to explore how nutrient and carbon concentration correlated with PLFA composition. A principal component analysis (PCA) was used to visualize the distribution of the PLFA composition.
To test the effect of wild boars on soil respiration, soil temperature, and soil moisture, we used LMMs with enclosure, environment, and month as explanatory variables and plot as a random factor. The respiration data was log-transformed to achieve equal variance of the residuals.
All statistical analyses were conducted in R 4.1.2 (R Core Team Citation2021). For descriptive analyses we used the R package psych (Revelle Citation2020), and graphs were created using the packages ggplot2 (Wickham Citation2016) and ggfortify (Tang et al. Citation2016).
Results
Soil properties and nutrient concentrations
The enclosures had a 15% higher soil bulk density (across lower and upper layer) than the reference areas in the pine forest, while no difference was detected in the spruce forest (environment x enclosure effect: F1,16 = 4.40, p = 0.05, ). This pattern was driven by a higher bulk density in the top layer of the pine enclosures (layer x enclosure effect: F1,16 = 5.05, p = 0.04). Enclosures and reference areas showed no difference in organic matter content in the soil (enclosure effect: F1,16 = 0.03, p = 0.87) () regardless of forest types (environment x enclosure effect: F1,16 = 1.13, p = 0.30) and soil depth (layer x enclosure effect: F1,16 = 0.20, p = 0.66).
Figure 2. Soil bulk density and soil organic matter content in the top (0–2 cm) and lower (2–5 cm) soil layer in reference and enclosures pine (a, c) and spruce (b, d) forests. Error bars show standard error (n = 5).

We did not detect effects of wild boar on soil C or N concentration (mg g−1) nor stock (g m−2) (p > 0.48; Figure S1; ). However, the pine enclosure had a lower P concentration and stock than the reference area, but no such difference was observed in the spruce forest (environment x enclosure effect: p < 0.01). Absorbed NO3− showed a strong interaction between the enclosure treatment and the forest type (environment x enclosure effect: F1,16 = 19.22, p < 0.001) (Figure S2a). In the pine forest, the enclosure had a lower NO3− concentration than the reference areas (p = 0.03). On the other hand, in the spruce forest the enclosure had a higher NO3− concentration than the reference areas (p = 0.002). Absorbed NH4+ was similar in enclosures and reference areas (enclosure effect: F1,16 = 1.25, p = 0.28) (Figure S2b), regardless of the forest type (environment x enclosure effect: F1,16 = 0.28, p = 0.60).
PLFA
The PLFA composition was different between the top and the lower layer (PERMANOVA, layer effect: R2 = 0.20, p = 0.0002), but as the layer effect was consistent across treatments, we pooled the two soil layers prior to further analyses. Enclosures and reference areas had a similar PLFA composition (PERMANOVA, enclosure effect: R2 = 0.02, p = 0.60) (), but showed clear differences between the pine and spruce forests (PERMANOVA, environment effect: R2 = 0.28, p = 0.004). Including nutrients and C concentration as predictor variables, one variable at a time, did not reveal an enclosure effect, but showed that these factors strongly influenced the PLFA composition, explaining up to 45% of the variation (). Testing individual PLFAs did not reveal any clear differences between enclosures and reference areas (all p > 0.10). The total PLFA concentration was not found to differ between enclosures and reference areas (enclosure effect: F1,16 = 0.18, p = 0.68) (), but the concentration was about 22% higher in the spruce forest (environment effect: F1,16 = 6.15, p = 0.03) compared to the pine forests, and 27% higher in the top soil layer (layer effect: F1,16 = 49.6, p < 0.0001) compared to the lower soil layer. We detected a higher fungi:bacteria ratio in the enclosures than in the reference areas (enclosure effect: F1,16 = 5.28, p = 0.04) () and these effects were consistent across soil depths and forest types (all p > 0.16). The ratio was generally lower in the lower soil layer, which was driven by particularly low values in the spruce forests (environment x layer effect: F1,16 = 19.8, p = 0.0001).
Figure 4. Principal component analysis (PCA) of phospholipid fatty acid (PLFA) composition of soil samples. (a) illustrates the scores for each plot (N = 20) together with the distribution of enclosures and reference areas, and (b) illustrates the individual PLFAs.

Figure 5. Total amount of PLFAs in enclosures and reference areas for the top (0–2 cm) soil (a) and the lower (2–5 cm) soil layer (b) fungi:bacteria ratio in the top (c) and lower layer (d) error bars show standard error (n = 5).

Table 1. PERMANOVA results showing explained variation (R2) of soil PLFA composition by experimental variables, soil nutrients and carbon concentrations. The first model shows the experimental factors while models 2–7 show the explanatory power of measured soil nutrients or soil carbon. In the second set of models the enclosure effect was similar to the effect in the first model and not shown here. The terms in the models are tested sequentially.
Soil temperature, moisture and respiration
Overall, the wild boars had limited impact on soil temperature and moisture. Soil temperature was somewhat higher in the enclosures during the summer months June – August and slightly lower in November (enclosure x month effect: F4,63.3 = 53.59, p < 0.001) (). These results between environments were not consistent over time (enclosure x month x environment effect: F4,63.3 = 10.99, p < 0.001), but this effect was small and did not change the overall pattern of how the treatment effect varied over the season. Soil moisture was on average consistently higher in the enclosures than the reference areas, but the effect was small and not statistically significant (enclosure effect: F1,16 = 2.16, p = 0.16) (), even between months. (enclosure x month effect: F3,48 = 1.12, p = 0.35) and forest types (environment x enclosure effect: F1,16 = 0.00, p = 0.97). The soil in the spruce forest was moister than the soil in the pine forest (F1,16 = 11.65, p = 0.004).
Figure 6. Temporal patterns of soil temperature (a), soil moisture (b), and soil respiration rate (c) over the measuring period June to November. Error bars show standard error (temperature: n = 10, moisture: n = 5, respiration: n = 10). Moisture data for November have been removed due to a technical failure.

The respiration rate across the measuring period was 24% lower (enclosure effect: F1,16 = 7.46, p = 0.01) in the enclosures than in the reference areas, with the greatest difference in September (-36%) and the smallest in June (-9%) (). These results were consistent throughout all months (month x enclosure effect: F4,464 = 1.86, p = 0.12) and for both forest types (environment x enclosure effect: F1,16 = 0.28, p = 0.60).
In general, soil temperature was negatively correlated with respiration (most notably in August: r = -0.37, p < 0.01) except in November where soil temperature was strongly positively correlated with respiration (r = 0.57, p < 0.01). Soil moisture had a less clear correlation with respiration, with the greatest correlation in August (r = 0.24, p = 0.016). Removing the effect of differences in soil temperature and soil moisture on soil respiration increased the support for a lower respiration in the enclosures (enclosure effect: p = 0.07 and p = 0.09, respectively). In September, when soil samples were collected for analysis, the total PLFA concentration was not correlated with respiration (r = 0.02, p = 0.24). However, the fungi:bacteria ratio was clearly negatively correlated with the respiration (r = -0.46, p = 0.04).
Discussion
Large animals can affect ecosystems in many ways. The rooting behaviour of wild boars presents a unique disturbance among ungulates, which can potentially alter important soil processes. Despite the apparent disturbance that the rooting imposed, we showed that many soil characteristics and processes did not differ between enclosures and reference sites, or the difference was small. Overall, our results were inconsistent with our expectations that wild boars would promote organic soil turnover, result in higher nutrient availability, bacteria abundance, and soil respiration, which raises new questions regarding how wild boars may influence forest ecosystem functioning.
Soil nutrients and microbial biomass
Soil nitrogen stocks were similar in enclosure and reference areas, while phosphorus was lower inside the pine enclosures. This indicates that the N and P added to the enclosures through occasional supplementary feeding did not result in an overall accumulation of nutrients inside the enclosures. Furthermore, wild boar had no consistent effect on nitrogen availability (NO3−, NH4+), which contrasts earlier studies from tropical, temperate and desert ecosystems that reported higher N and NH4+ concentrations in wild boar rooted areas than in unrooted areas (Siemann et al. Citation2009; Cuevas et al. Citation2012; Wirthner et al. Citation2012; Long et al. Citation2017). Higher nutrient availability is often ascribed to increased microbial activity after soil disturbance (e.g., Siemann et al. Citation2009; Wirthner et al. Citation2012). In our study, however, we found neither positive effects on microbial biomass (here, total PLFA:s), nor positive effects on soil respiration rate in the heavily disturbed soils in the enclosures, which indicate lower microbial activity and may potentially explain the lack of response in nutrient availability. Further, we found no shift in phospholipid fatty acid (PLFA) composition following disturbance by wild boars, which indicates that despite the severe soil disturbance, there was no major shift in the composition of microbial groups. The overall minor change in the PLFA composition was unexpected, as microbial communities often respond to disturbances through a shift in composition or change in abundance (Allison and Martiny Citation2008). For example, moulds and yeast fungi are expected to increase together with bacteria during soil mixing (Lindahl et al. Citation2010; Sterkenburg et al. Citation2015). Such an increase in fungi is likely the reason behind the detected minor increase in fungi:bacteria ratio across forest types and soil depths. This is in contrast with our hypothesised decrease of the fungi:bacteria ratio, which was based on our prediction that rooting would increase nutrient availability and, consequently, bacteria abundance (Wang et al. Citation2019). Higher nitrogen availability due to rooting has been reported in previous studies in non-boreal systems (Siemann et al. Citation2009; Cuevas et al. Citation2012; Wirthner et al. Citation2012; Long et al. Citation2017), and it is possible that boreal systems react differently due to the importance of fungi in this ecosystem (Lindahl et al. Citation2007). Alternatively, there is a pulse of increased nutrients that is associated with microbial changes immediately after rooting that declines over time, which is similar to other disturbances in boreal forests where nutrient availability is known to increase (Lindahl et al. Citation2010). Our study did not measure nutrients or PLFA immediately after rooting, and studies following the succession after rooting are needed.
Instead of wild boar presence being an important driver of the PLFA composition, we found that nitrogen concentration explained the PLFA composition well. Several studies have found that differences in N concentrations affect the microbial community (Gallo et al. Citation2004; Demoling et al. Citation2008; Liu et al. Citation2013). Possibly, the similarities between the areas inside and outside the enclosures regarding N content explain the similarities in microbial communities.
Do wild boars increase soil respiration?
In contrast to our expectations, we found lower soil respiration rates inside the wild boar enclosures than outside. Thus, our results contrast with studies conducted in deciduous forests in China and Switzerland (Risch et al. Citation2010; Liu et al. Citation2020), and in a salt marsh in the USA (Persico et al. Citation2017), which all report greater soil respiration due to wild boar rooting. One study, like ours, reported lower soil respiration under wild boar rooting, but it investigated a desert habitat that has little in common with boreal forests (Cuevas et al. Citation2012). These previous studies were also the basis for a synthesis that predicted potentially large global CO2 emissions due to soil damage caused by wild boars (O’Bryan et al. Citation2021). Our results clearly demonstrate that the effects of wild boars are less consistent than previously believed, and highlight the need for additional studies that could clarify the influence wild boar on soil processes and their context dependency, before conclusions regarding wild boar impact on soil carbon can be made at a global scale.
There are many proposed mechanisms for how wild boar rooting can alter soil respiration. Risch et al. (Citation2010) and Cuevas et al. (Citation2012) suggested that rooting affects soil moisture, which is known to influence soil respiration. This is similar to the idea put forward by Long et al. (Citation2017) that rooting leads to increased soil bulk density, which often means wetter and less aerated soil. However, Liu et al. (Citation2020) found no differences in soil moisture content between areas with and without wild boars, but reported differences in soil respiration. We found small differences in soil bulk density and moisture between enclosures and reference areas, but the correlation between moisture and soil respiration was weak. Risch et al. (Citation2010) and Liu et al. (Citation2020) discuss another possible mechanism related to the mixing of the soil layers. Mixing should increase the substrate available for soil microorganisms, resulting in an increased soil respiration rate. This mechanism may contribute to a respiration pulse directly after wild boar rooting. Although we might have missed such a respiration peak as we measured respiration in areas that had been disturbed for a long period, and currently had very sparse ground vegetation, we did not observe any change in soil carbon stock despite eight years of wild boar disturbance. This is in line with other wild boar studies (Long et al. Citation2017; Liu et al. Citation2020) and soil mixing studies (Don et al. Citation2019), and does not support recent claims that wild boar presence will decrease soil carbon stock (O’Bryan et al. Citation2021).
It is also possible that wild boar soil damage affects root respiration (i.e., autotrophic) and heterotrophic respiration differently. In a boreal forest, root respiration can account for > 50% of the total soil respiration (Högberg et al. Citation2001), and our finding that wild boar enclosures produced lower respiration rates could be the result of changes in root respiration while heterotrophic respiration remained unaffected. The low cover of ground vegetation in the wild boar enclosures may have potentially contributed to the lower soil respiration, but it is unlikely the main driver of the lower respiration rates in our study as the result was consistent also in the spruce forest, which had very sparse ground vegetation both inside and outside the enclosure.
In conclusion, we showed that despite high population densities, wild boars can have a limited impact on boreal soil carbon stock, respiration, and microbial community structure (e.g., fungi:bacteria). Interestingly, the fungi:bacteria ratio decreased in rooted areas, which is in contrast with the idea that wild boar rooting increases nutrient availability. Our results were consistent across both a pine and a spruce dominated forest. Although this study used enclosures, and should be interpreted cautiously as we only investigated two stands, the results do not support previous reports on increased carbon losses from soil rooted by wild boar. The generality of these effects needs further investigations that test the effects of wild boars under natural population densities and across a broader range of boreal forests and regions.
Open source statement
Data and R-code are available for reviewers at: https://github.com/ggranath/wildboar_enclosure/
Acknowledgments
We thank Almunge Hundcenter for access to the enclosures and B. Muscarella for valuable comments on this manuscript.
Disclosure statement
No potential conflict of interest was reported by the authors.
Additional information
Funding
References
- Allison S, Martiny J. 2008. Resistance, resilience and redundancy in microbial communities. null. 105(Supp 1):11512–11519. doi:10.1073/pnas.0801925105.
- Allison V, Miller M, Jastrow J, Matamala R, Zak D. 2005. Changes in soil microbial community structure in a tallgrass prairie chronosequence. Soil Sci Soc Am J. 69(5):1412–1421. doi:10.2136/sssaj2004.0252.
- Andersen J. 1976. An ignition method for determination of total phosphorous in lake sediments. Water Res. 10(4):329–331. doi:10.1016/0043-1354(76)90175-5.
- Bååth E, Andersson T. 2003. Comparison of soil fungal/bacterial ratios in a pH gradient using physiological and PLFA-based techniques. Soil Biol Biochem. 35(7):955–963. doi:10.1016/S0038-0717(03)00154-8.
- Bardgett D, Wardle D. 2010. Aboveground-belowground linkages. Oxford: Oxford University Press.
- Barrios-Garcia M, Ballari S. 2012. Impact of wild boar (Sus scrofa) in its introduced and native range: a review. Biol Invasions. 14(11):2283–2300. doi:10.1007/s10530-012-0229-6.
- Bates D, Maechler M, Bolker B, Walker S. 2015. Fitting linear mixed-effects models using lme4. J Stat Softw. 67(1):1–48. doi:10.18637/jss.v067.i01.
- Berg B, McClaugherty C. 2014. Plant litter decomposition, humus formation, carbon sequestration. 3rd ed. Berlin: Springer.
- Bernes C, Macura B, Jonsson B, Junninen K, Muller J, Sandstrom J, Lohmus A, Macdonald E. 2018. Manipulating ungulate herbivory in temperate and boreal forests: effects on vegetation and invertebrates. A systematic review. Environ Evid. 7(1):13. doi:10.1186/s13750-018-0125-3.
- Carter M, Gregorich E, Carter MR, Gregorich EG. 2007. Soil sampling and methods of analysis. Ottawa: Canadian Society of Soil Science.
- Clemmensen K, Bahr A, Ovaskainen O, Dahlberg A, Ekblad A, Wallander H, Stenlid J, Finlay R, Wardle D, Lindahl B. 2013. Roots and associated fungi drive long-term carbon sequestration in boreal forests. Science. 339(6127):1615–1618. doi:10.1126/science.1231923.
- Cuevas M, Mastrantonio L, Ojeda R, Jaksic M. 2012. Effects of wild boar disturbance on vegetation and soil properties in the Monte Desert, Argentina. Mamm Biol. 77(4):299–306. doi:10.1016/j.mambio.2012.02.003.
- Davies GM, Gray A. 2015. Don’t let spurious accusations of pseudoreplication limit our ability to learn from natural experiments (and other messy kinds of ecological monitoring). Ecol Evol. 5(22):5295–5304. doi:10.1002/ece3.1782.
- Demoling F, Nilsson L, Bååth E. 2008. Bacterial and fungal response to nitrogen fertilization in three coniferous forest soils. Soil Biol Biochem. 40(2):370–379. doi:10.1016/j.soilbio.2007.08.019.
- Don A. 2022. No threat to global soil carbon stocks by wild boar grubbing. Glob Change Biol. 28(3):685–686. doi:10.1111/gcb.15990.
- Don A, Hagen C, Grüneberg E, Vos C. 2019. Simulated wild boar bioturbation increases the stability of forest soil carbon. Biogeosci. 16(21):4145–4155. doi:10.5194/bg-16-4145-2019.
- Fox J, Weisberg S. 2019. An R companion to applied regression. Thousand Oaks CA: Sage.
- Frostegård Å, Bååth E. 1996. The use of phospholipid fatty acid analysis to estimate bacterial and fungal biomass in soil. Boil Fert Soils. 22(1–2):59–65. doi:10.1007/BF00384433.
- Frostegård Å, Tunlid A, Bååth E. 1991. Microbial biomass measured as total lipid phosphate in soils of different organic content. J Microbiol Meth. 14(3):151–163. doi:10.1016/0167-7012(91)90018-L.
- Gallo M, Amonette R, Lauber C, Sinsabaugh R, Zak D. 2004. Microbial community structure and oxidative enzyme activity in nitrogen-amended north temperate forest soils. Microb Ecol. 48(2):218–229. doi:10.1007/s00248-003-9001-x.
- Gray S, Roloff G, Kramer D, Etter D, Vercauteren K, Montgomery R. 2020. Effects of wild pig disturbance on forest vegetation and soils. J Wild Mgmt. 84(4):739–748. doi:10.1002/jwmg.21845.
- Gutiérrez J, Jones C. 2006. Physical ecosystem engineers as agents of biogeochemical heterogeneity. BioScience. 56(3):227–236. doi:10.1641/0006-356820060560227:PEEAAO2.0.CO;2
- Högberg P, Nordgren A, Buchmann N, Taylor A, Ekblad A, Högberg M, Nyberg G, Ottosson-Löfvenius M, Read D. 2001. Large-scale forest girdling shows that current photosynthesis drives soil respiration. Nature. 411(6839):789–792. doi:10.1038/35081058.
- Hoogsteen J, Lantinga E, Bakker E, Groot J, Tittonell P. 2015. Estimating soil organic carbon through loss on ignition: effects of ignition conditions and structural water loss. Eur J Soil Sci. 66(2):320–328. doi:10.1111/ejss.12224.
- Jackson L, Calderonb F, Steenwertha K, Scowc K, Rolstonc D. 2003. Responses of soil microbial processes and community structure to tillage events and implications for soil quality. Geoderma. 114(3–4):305–317. doi:10.1016/S0016-7061(03)00046-6.
- Jensen P. 2017. The ethology of domestic animals: an introductory text. Wallingford, UK: CABI.
- Kempers A, Zweers A. 2008. Ammonium determination in soil extracts by the salicylate method. Communicat Soil Sci Plant Analy. 17(7):715–723. doi:10.1080/00103628609367745.
- Kolstad A, Austrheim G, Solberg E, Venete A, Woodin S, Speed J. 2018. Cervid exclusion alters boreal forest properties with little cascading impacts on soils. Ecosystems. 21(5):1027–1041. doi:10.1007/s10021-017-0202-4.
- Kuznetsova A, Brockhoff P, Christensen R. 2017. lmertest package: tests in linear mixed effects models. J Stat Softw. 82(13):1–26.
- Lacki M, Lancia R. 1986. Effects of wild pigs on beech growth in great smoky mountains national park. J Wildlife Manage. 50:655–659. doi:10.2307/3800976.
- Leroux S, Wiersma Y, Wal E. 2020. Herbivore impacts on carbon cycling in boreal forests. Trends Ecol Evol. 35(11):1001–1010. doi:10.1016/j.tree.2020.07.009.
- Lindahl B, De Boer W, Finlay R. 2010. Disruption of root carbon transport into forest humus stimulates fungal opportunists at the expense of mycorrhizal fungi. Isme J. 4(7):872–881. doi:10.1038/ismej.2010.19.
- Lindahl B, Ihrmark K, Boberg J, Trumbore S, Högberg P, Stenlid J, Finlay R. 2007. Spatial separation of litter decomposition and mycorrhizal nitrogen uptake in a boreal forest. New Phytol. 173(3):611–620. doi:10.1111/j.1469-8137.2006.01936.x.
- Liu J, Feng C, Wang D, Wang L, Wilsey B, Zhong Z, Firn J. 2015. Impacts of grazing by different large herbivores in grassland depend on plant species diversity. J Appl Ecol. 52(4):1053–1062. doi:10.1111/1365-2664.12456.
- Liu Y, Liu X, Yang Z, Li G, Liu S. 2020. Wild boar grubbing causes organic carbon loss from both top- and sub-soil in an oak forest in central China. Forest Ecol Manag. 464:118059. doi:10.1016/j.foreco.2020.118059.
- Liu L, Zhang T, Gilliam F, Gundersen P, Zhang W, Chen H, Mo J. 2013. Interactive effects of nitrogen and phosphorous on soil microbial communities in a tropical forest. PLos One. 8:e61188. doi:10.1371/journal.pone.0061188.
- Long M, Litton C, Giardina C, Deenik J, Cole R, Sparks J. 2017. Impact of nonnative feral pig removal on soil structure and nutrient availability in Hawaiian tropical montane wet forests. Biol Invas. 19(3):749–763. doi:10.1007/s10530-017-1368-6.
- Maaroufi N, Taylor A, Ehnes R, Andrén H, Kjellander P, Björkman C, Kätterer T, Klapwijk M. 2022. Northward range expansion of rooting ungulates decreases detritivore and predatory mite abundances in boreal forests. Royal Soc Open Sci. 9(7):211283. doi:10.1098/rsos.211283.
- Mack M, D’Antonio C. 1998. Impacts of biological invasions on disturbance regimes. Trends Ecol Evol. 13(5):195–198. doi:10.1016/S0169-5347(97)01286-X.
- O’Bryan C, Patton N, Hone J, Lewis J, Berdejo-Espinola V, Risch D, Holden M, McDonald-Madden E. 2021. Unrecognized threat to global soil carbon by a widespread invasive species. Glob Change Biol. 28(3):877–882. doi:10.1111/gcb.15769.
- Oksanen F, Blanchet G, Friendly M, Kindt R, Legendre P, McGlinn D, Minchin P, O’Hara R, Simpson G, Solymos P, et al. 2020. Vegan: community ecology package. R Package Version 2.5-7. https://CRAN.R-project.org/package=vegan.
- Perry D, Oren R, Hart S. 2008. Forest ecosystems. Baltimore: Johns Hopkins University Press.
- Persico E, Sharp S, Angelini C. 2017. Feral hog disturbance alters carbon dynamics in southeastern US salt marshes. Mar Ecol Prog Ser. 580:57–68. doi:10.3354/meps12282.
- QGIS Development Team. 2020. QGIS geographic information system. Open Source Geospatial Foundation Project. https://www.qgis.org/en/site/.
- Qian P, Schoenau J. 2005. Use of ion-exchange membrane to assess nitrogen-supply power of soils. J Plant Nutr. 28(12):2193–2200. doi:10.1080/01904160500324717.
- R Core Team. 2021. R: a language and environment for statistical computing. Vienna, Austria: R Foundation for Statistical Computing. https://www.R-project.org/.
- Revelle W. 2020. Psych: procedures for psychological, psychometric and personality research. Evanston, Illinois: Northwestern University.
- Risch A, Wirthner S, Busse M, Pade-Dumroese D, Schütz M. 2010. Grubbing by wild boars (Sus scrofa L.) and its impact on hardwood forest soil carbon dioxide emissions in Switzerland. Oecologia. 164(3):773–784. doi:10.1007/s00442-010-1665-6.
- Siemann E, Carrillo J, Gabler C, Zipp R, Rogers W. 2009. Experimental test of the impacts of feral hogs on forest dynamics and processes in the southeastern US. Forest Ecol Manag. 258(5):546–553. doi:10.1016/j.foreco.2009.03.056.
- SMHI. 2009. Normal årsmedeltemperatur. Norrköping, Sweden: Swedish Meteorological and Hydrological Institute.
- Sterkenburg E, Barh A, Durling M, Clemmensen K, Lindahl B. 2015. Changes in fungal communities along a boreal forest soil fertility gradient. New Phytol. 207(4):1145–1158. doi:10.1111/nph.13426.
- Sullivan P, Ellison S, McNown R, Brownlee A, Sveinbjörnsson B. 2015. Evidence of soil nutrient availability as the proximate constraint on growth of treeline trees in Northwest Alaska. Ecology. 96(3):716–727. doi:10.1890/14-0626.1.
- Svenska jägareförbundet. 2018. Förekomst och förvaltning av vildsvin i Sverige – en analys från svenska jägareförbundets viltövervakning 2017. Nyköping, Sweden: Svenska Jägareförbundet.
- Swedish Environmental Protection Agency. 2019. Nationell förvaltningsplan för vildsvin. Stockholm, Sweden: Swedish Environmental Protection Agency.
- Tamm C. 1991. Nitrogen in terrestrial ecosystems: questions of productivity, vegetational changes, and ecosystem stability. Berlin: Springer-Verlag.
- Tang Y, Horikoshi M, Li W. 2016. Ggfortify: unified interface to visualize statistical result of popular R packages. R J. 8(2):474–485.
- Vázguez D. 2002. Multiple effects of introduced mammalian herbivores in a temperate forest. Biol Invasions. 4(1/2):175–191. doi:10.1023/A:1020522923905.
- Wang X, Zhang W, Shao Y, Zhao J, Zhou L, Zou X, Fu S. 2019. Fungi to bacteria ratio: historical misinterpretations and potential implications. Acta Oecologica. 95:1–11. doi:10.1016/j.actao.2018.10.003.
- Wickham H. 2016. Ggplot2: elegant graphics for data analysis. New York: Springer-Verlag.
- Wirthner S, Schütz M, Page-Dumroese D, Busse M, Kirchner J, Risch A. 2012. Do changes in soil properties after rooting by wild boars (Sus scrofa) affect understory vegetation in Swiss hardwood forests? Can J Forest Res. 42(3):585–592. doi:10.1139/x2012-013.
- Yao H, He Z, Wilson M, Campbell C. 2000. Microbial biomass and community structure in a sequence of soils with increasing fertility and changing land use. Microb Ecol. 40(3):223–237. doi:10.1007/s002480000053.