Abstract
Laboratory medicine is integral to public health and healthcare provision, and it relies on numerous analytical techniques to provide timely, objective data to healthcare professionals to guide disease prevention, diagnosis, treatment, and monitoring. Driven and defined by a culture of innovation, recent technological advances have revolutionized modern laboratory medicine and added significant value and visibility to its role in healthcare and clinical decision-making. Noteworthy innovations in laboratory automation, genomics, nuclear magnetic resonance spectroscopy, mass spectrometry, microfluidics, and electronic tools have changed the face of omics research. The growing application of these technologies, as well as their integration with microtechnology and point-of-care testing, has contributed to improved patient outcomes and narrowing of the clinical-laboratory interface to facilitate a patient-centered approach to healthcare. However, to adequately capitalize on these advancements, new tools such as artificial intelligence and data mining are needed to harness the exciting potential of medical big data derived from these novel techniques. The current review provides an overview of the recent technological advancements in laboratory medicine, with a critical discussion on their clinical utility and future perspectives. The promise and potential for precision and personalized medicine is also discussed and appraised, with specific attention paid to the contingency of its success on advanced information technology capabilities.
Introduction
Laboratory medicine plays an integral role in healthcare by providing healthcare professionals with objective data to guide disease prevention, risk assessment, diagnosis, prognosis, treatment, and monitoring of patients, which ensures safe, appropriate, and effective clinical decision-making. In recent years, major technological advances in laboratory medicine have greatly improved clinical laboratory diagnostics and monitoring, further enhancing the quality of patient care. In turn, these technological innovations have added recognizable value to laboratory medicine in the healthcare sector.
Traditionally, clinical chemistry, hematology, and microbiology testing was extremely laborious for laboratory staff, who were responsible for the often many steps involved in the analytical process, from obtaining biological specimen to their eventual discard. In recent decades, however, clinical chemistry and hematology laboratories have adopted largely automated analyzers that have broadly improved laboratory testing efficiency, costs, and laboratory-driven errors [Citation1–4]. Furthermore, noteworthy analytical advancements in genetics and genomics, nuclear magnetic resonance (NMR) spectroscopy, mass spectrometry (MS), and microfluidics have paralleled increased laboratory automation to enable a new era of laboratory medicine. Indeed, the development and evolution of next-generation and single-cell sequencing has revolutionized the field of genomics and transcriptomics to allow whole-genome DNA and RNA sequencing with high-throughput and lower cost [Citation5]. Proteomics and metabolomics are currently defined by advancements in NMR and MS technologies, expanding their application across several clinical disciplines [Citation6–8]. Further, the novel application of microfluidic technology has been readily applied to several laboratory tools, including lab-on-a-chip technology [Citation9], single-cell omics research [Citation10, Citation11], and point-of-care testing (POCT) devices [Citation12]. POCT systems have also been uniquely positioned to exploit the technological advancements made in laboratory medicine and adapt them to a portable or miniature size for application in near-patient settings. Furthermore, advancements in bioinformatics and information technology have been concurrently developing. Indeed, the generation of big data from advanced analytical techniques must be matched with sophisticated machine learning and artificial intelligence (AI) suitable for data mining and deep learning [Citation13]. Moreover, the culture of innovation within laboratory medicine technologies and advanced AI may pave the way for an era of precision and personalized medicine, adding significant value to the critical role of the laboratory within healthcare provision. In this review, we will discuss the latest technological advancements within laboratory medicine, including their clinical applications, current challenges, and opportunities for future directions. In addition, we will comment on the promise for a future of precision medicine based on advanced analytic techniques and the contingency of advanced bioinformatics ().
Figure 1. Laboratory medicine is defined by advanced analytical innovations, and the promise of precision and personalized medicine is found at the intersection of the data derived from these novel techniques and the computational analyses required to derive clinical meaning. In amalgamating the extensive data derived from advanced sample analytics, sophisticated information technology may facilitate a new, patient-centered, era of laboratory medicine.
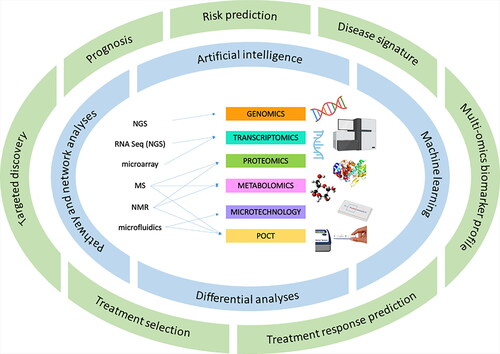
Total laboratory automation
Modern laboratory medicine is defined by an era of automation. The concept of laboratory automation was originally introduced by the AutoAnalyzer [Citation14], which performed continuous flow analysis, and the Robot Chemist [Citation15, Citation16], which automated traditional manual analytical steps. Subsequent generations of stand-alone analyzers have drastically changed the face and scope of laboratory medicine, through improved efficiency, higher throughput, larger assay menus, and reduced errors [Citation1–4]. Further automation was introduced to tackle time-consuming and laborious steps within the pre-analytical phase, including sample identification, sorting, and centrifugation, as well as within the post-analytical phase, including specimen storage and archiving. These technological advancements ultimately culminated in the generation of total laboratory automation (TLA): several instruments that are run in parallel using a robotic track system and AI to link all stages of the analytical process. Marzinke has previously described the image of centralized TLA systems in detail [Citation17].
Laboratory applications and recent advancements
Though all modern clinical chemistry and hematology analyzers are highly automated, only a few TLA systems have been developed. The Cobas® (Roche Diagnostics) is an automated core laboratory instrument, capable of independently handling sample processing, analysis, and storage. When combined with one or multiple connection modules on a track system, this instrument can also perform sample sorting, de-capping, quality control, aliquoting, and re-capping with in vitro diagnostic specimen tubes. Similarly, Abbott Diagnostics recently released the Accelerator, which is a highly automated system for pre-analytic tasks that can be connected to a multi-instrument core laboratory system. The Accelerator has the advantage of being ‘open’ to facilitate integration with third-party systems, improving the consolidation of testing across laboratory disciplines. Other examples of commercially available TLA systems are the Power Express Clinical Automation system (Beckman), TCAutomation™ (Thermo Fisher), Aptio® Automation (Siemens Healthineers), and VITROS® Automation Solutions (Ortho Biomedical). These centralized systems have been well-evidenced to reduce human error and improve analyzer performance [Citation18–23].
In addition, recent advancements in the digitization of culture plate images and the ability to electronically read incubated plates have facilitated the introduction of TLA to microbiology and the subsequent revolution of this field [Citation4, Citation22, Citation24, Citation25]. Currently, there are two commercially available microbiology automation systems that have been adopted by clinical laboratories: the Kiestra™ (Beckton Diagnostics) and WASPLab® (Copan Diagnostics Inc.). Both instruments can employ varying degrees of automation, from front-end processing alone (i.e. automated plating) to TLA [Citation4, Citation22, Citation24, Citation26–29]. Due to the many manual and laborious components of clinical microbiology, increased automation offers extensive benefits, including reduced costs [Citation19, Citation24, Citation30, Citation31], freeing technicians for more skilled responsibilities (e.g. microscopy, plate interpretation, antimicrobial susceptibility testing) [Citation22, Citation25, Citation32], improved efficiency (assessed via LEAN or other benchmarks) [Citation19, Citation31, Citation33], reduced turnaround time (TAT) [Citation34–36], improved performance [Citation34], and consolidation of laboratory space [Citation29].
Challenges and considerations of TLA
With increased automation comes inherent changes in clinical laboratory workflow and personnel requirements. Thomson and McElvania determined that a reduction in 6 full-time equivalents (FTEs) was necessary following implementation, given appropriate training of retained staff [Citation26]. In addition, it is suggested that there will be an inherent loss of technical knowledge and skills as tasks conventionally performed manually become automated [Citation22, Citation37]. Similarly, staff training must be adjusted to focus on skills that best complement the TLA system. In microbiology, for example, experience with plate streaking and colony recognition will be less important than skills associated with visual pattern recognition of the relative numbers of pathogen versus specimen commensals present on agar surfaces and fluency with computer software. Changes in workflow and technician scheduling may also ensue; for example, TLA systems allow plate reading to be completed 24/7 as opposed to during traditional day-shifts only [Citation38]. Furthermore, the laboratory must also confirm compatibility of the TLA system with the laboratory information system (LIS) [Citation29]. Finally, during TLA downtime, track malfunctions, routine reagent replacement, or waste disposal, the entire system will be non-operational. Thus, laboratories must be equipped to proceed via manual processes in the interim if needed or have backup systems in place.
A case example is demonstrated by Hoi-Ying et al., who described the clinical and laboratory outcomes following implementation of a TLA system suited for hematology, coagulation, and chemistry testing [Citation39]. Here, discrete processing steps in specimen handling were reduced by 86% using TLA or only a partially automated laboratory [Citation39]. Their testing footprint was reduced by 45% and they ultimately required 2 FTE fewer testing personnel [Citation39]. Finally, hands-on time associated with add-on processes was reduced by 82% [Citation39], underscoring the improved workflow efficiency achieved by TLA and resultant reduction in personnel requirements. It is noteworthy to mention that automation should only be implemented to the extent that is necessary and optimal for a given clinical laboratory. Marzinke provides a representative workflow analysis that may be used to both identify areas for improved efficiency and predict the impact of TLA in the clinical environment [Citation17]. Furthermore, while several reports have documented the improvements to clinical workflow and TAT that ensue with increased automation [Citation19, Citation36], seldom studies have assessed the impact of these instruments on patient outcomes. As prolonged TAT has known negative patient impact [Citation40], future work should evaluate the clinical impact of TLA systems to better inform their clinical implementation.
‘Omics’ revolutionized
Recent advancements in biotechnology over the previous decades have allowed omics research to span the breadth of molecular biology, now covering fields of genomics, transcriptomics, metabolomics, and proteomics [Citation41]. Each technology exploits specific analytical principles to target a distinct type of molecule and ultimately generates a systemic profile of a biological system [Citation41, Citation42].
Advancements in genetic sequencing techniques
Led by significant technological advancements over the previous two decades, the fields of genomics and transcriptomics are undergoing a paradigm shift, away from bulk tissue analysis and towards the comprehensive study of single-cell sequencing (SCS) [Citation43]. This breakthrough was the result of several milestones in genetic sequencing analytics, which have previously been summarized in greater detail [Citation43, Citation44]. Briefly, quantitative microarray technologies capable of measuring genome-wide DNA and RNA were developed in the 1990s but required too much input material for single-cell analysis. In the 1990s to early 2000s, whole-transcriptome amplification (WTA) [Citation45] and whole-genome amplification (WGA) [Citation46, Citation47] were developed to amplify genome-wide DNA and RNA, overcoming the limitations of polymerase chain reaction (PCR) technology. Next-generation sequencing (NGS) was subsequently developed, which allowed for genome-wide DNA and RNA sequencing at a drastically increased throughput and reduced cost compared to sequencing techniques used since the Human Genome Project [Citation48]. These advances ultimately culminated in the invention of the first genome-wide single-cell DNA [Citation49] and RNA [Citation47] sequencing methods. In accounting for inter-cell variability, SCS techniques improved upon traditional bulk DNA and RNA analyses by enabling the accurate quantification of cellular and genetic heterogeneity within a tissue sample [Citation5].
Advancements in genomics and clinical applications
Omics research has lent itself most readily to the field of oncology [Citation50, Citation51], and one emerging application of NGS clinical assays is for the quantification of circulating tumor DNA (ctDNA) from plasma. Various NGS technologies may be employed, including Cancer Personalized Profiling by deep Sequencing (CAPP-Seq) [Citation52], which detects ctDNA, or cell-free methylated NDA immunoprecipitation-sequencing (cfMeDIP-seq) [Citation53, Citation54], which identifies regions of the genome with DNA methylation. The detection of cancer from the blood has introduced the concept of liquid biopsy in oncology, which can enable the molecular landscape of a tumor to be profiled in real-time, offering clear advantages from traditional tissue biopsies in terms of invasiveness and accessibility. This technology holds exciting promise in the field of precision oncology, wherein clinical applications include prediction of therapy response, monitoring for residual disease post-surgical resection, serial monitoring for emergence of treatment resistance, and early cancer detection [Citation55] (). The clinical validity of ctDNA assays has already been shown in lung and colorectal cancers [Citation56], targeting EGFR and KRAS, respectively. A growing number of groups are investigating and expanding the utility for these assays in various cancers and applications [Citation57–59], and future work should continue to evaluate the potential of these novel methods outside of oncology.
Figure 2. Laboratory methods and clinical applications of liquid biopsy in cancer screening, monitoring, therapeutic prediction, and treatment selection. From a liquid biopsy (i.e. blood sample), circulating tumour cells, point mutations, amplifications and deletions, chromosomal abnormalities, protein expression and phosphorylation, translocations, and epigenetic modifications can be detected using a variety of genetic sequencing technologies. Followed with sophisticated computational analyses, this novel laboratory technique can be used in early cancer detection, disease monitoring, prognosis, predicting response to therapy, and providing personalized drug selection.

Advancements in transcriptomics and clinical applications
Advancements in high-throughput RNA sequencing have highlighted the role for transcriptomics in biological and clinical research. Transcriptomics can be performed via microarrays or RNA sequencing using NGS technologies [Citation60]. In clinical research, microarrays are currently more popular due to their large throughput, high detection speed, simple and timely data processing, and relatively low cost [Citation60]. However, single-cell over bulk RNA-seq has also emerged in the literature over the previous decade, with a major advancement marked by the development of single-cell tagged reverse transcription sequencing (STRT-seq) in 2011 [Citation61], proceeding the initial sc-RNA seq feasibility experiment [Citation47]. In 2015, Klein et al. [Citation62] and Macosko et al. [Citation63] published the major breakthrough in RNA-seq technologies, inDrop® and Drop-seq, wherein single cells are encapsulated within droplet microreactors via microfluidic and nucleotide barcoding technology. Several groups have since sought to assess the clinical utility of RNA-seq methods. For example, Chang et al. recently developed Hydro-Seq, a scalable hydro-dynamic scRNA-seq barcoding technique for the high-throughput analysis of circulating tumor cells (CTCs) [Citation64]. With this technology, the authors were able to identify drug targets for targeted and hormone therapy in breast cancer [Citation64], thereby underscoring the clinical potential for sc-RNA-seq in precision medicine.
Advancements in metabolomics and proteomics and their clinical applications
Advances in NMR [Citation8] and MS [Citation6, Citation7] technologies have largely defined the current fields of proteomics and metabolomics. Concerned with the identification and quantification of all proteins and metabolites in a given specimen, these omics fields are predominantly focused on identifying novel biomarkers and therapeutic targets for various disease pathologies. The combination of liquid chromatography (LC), a sample separation technique, with tandem MS (MS/MS), which involves multiple steps of MS selection with fragmentation between stages, is a common analytical platform in laboratory medicine. For example, in newborn screening, LC-MS/MS has expanded its breadth to detect a wide range of inborn errors of metabolism [Citation65–67]. In addition, this technique holds immense potential in biomarker discovery for many proteomic and/or metabolic diseases. For example, Xu et al. used this analytical technique to characterize the metabolic profile of Type 2 Diabetes at various stages of pathogenesis [Citation68]. Similarly, NMR is also commonly used in metabolomics [Citation8]. NMR and MS differ in the analytical principles they exploit, and as such, they offer distinct advantages and optimal uses. For example, MS has high sensitivity, a wide detection range, and can be coupled with various separation techniques (e.g. LC), whereas NMR has a higher throughput, requires minimal sample manipulation, is non-destructive to samples, and has better reproducibility [Citation69–71]. Nonetheless, the methods are largely concordant with matched sample analysis. Yet, the objective of an analysis may warrant the use of one method over the other, to capitalize on their strengths and ensure optimal test performance (). In fact, combining MS and NMR datasets from the same samples has recently been suggested to enhance the accuracy of metabolite identification and provide a more detailed analysis of the metabolome than with either technique alone [Citation72].
Challenges and future potential of omics
Recently, single-cell genome-wide approaches have presented the opportunity to measure several types of molecules (e.g. DNA, RNA, protein, chromatin) simultaneously to ultimately establish profiles of different molecules from a single cell in parallel. This notion has been coined ‘multi-omics’: the combination of multiple omics data sets to generate a comprehensive biological model. From a single cell, the genome, methylome, or chromatin accessibility can be assayed from genomic DNA, RNA can be used for transcriptome profiling, proteins to profile the proteome, and small metabolites for metabolomics. These advances contribute to the emerging paradigm of precision medicine and personalized laboratory medicine, which take into account a breadth of biological markers that define disease pathology, as opposed to a single biomarker of disease [Citation41, Citation42, Citation73]. However, the fruition of analytical omics advancements is contingent on our ability to manage and analyze the mass amount of data generated from such techniques. Indeed, omics technologies are increasingly able to generate an abundance of potentially clinically useful data, although stringent data mining techniques will be required for filtering. By leveraging the intersection of machine learning, statistics, and database systems, we can discover patterns in large datasets containing laboratory test results and patient information. Harnessing these methods has the potential to revolutionize the clinical applications of omics technology. For example, Rose et al. conducted a longitudinal cohort study of integrative personalized omics profiling with samples collected quarterly over eight years [Citation74]. Combined with wearable monitoring data, these authors used multi-omics techniques to identify 67 health discoveries meritorious of clinical action as well as several molecular pathways associated with metabolic, cardiovascular, and oncologic pathologies, including prediction modeling for insulin resistance [Citation74]. Taken together, multi-omics profiling may hold the potential to revolutionize laboratory medicine, and future work should investigate bioinformatics approaches required to meet said potential.
Microtechnology
Microtechnology has become increasingly applied in laboratory medicine. Prominent examples of this are microfluidic devices such as the ‘lab-on-a-chip’ (LOC) or micro total analysis system (μTAS), originally proposed by Manz et al. [Citation75]. These miniature devices are composed of a network of microchannels or nanochannels that allow for the integration of multiple laboratory processes [Citation76]. A variety of microfluidic fabrication techniques may be used to manufacture these chips on various materials, including metal, silicon, glass, ceramics, paper, and various polymers, depending on their intended use [Citation77].
Diagnostic applications and recent advancements
Microfluidic devices serve several diagnostic applications, including nucleic acid testing and immunoassay [Citation78]. For example, these microfluidic systems are commonly used for diagnosing viral detection using nucleic acid amplification testing (NAAT) (e.g. PCR) and immunoassay (e.g. ELISA) methods [Citation79]. The recent COVID-19 pandemic has further highlighted the value of these devices for rapid viral detection at the point-of-care (POC), and many microfluidic chip devices were developed to detect SARS-CoV-2 RNA and protein biomarkers such as antigens and antibodies [Citation80]. For example, Lin et al. recently developed an integrated microfluidic LOC device for simultaneous detection of SARS-COV-2 antigen and IgG/IgM antibodies in only 15 min [Citation9]. Similar microfluidic devices are being used in the context of cardiovascular disease. In 2019, Dinter et al. developed a microfluidic microbead-based chip for simultaneous detection and quantification of cell-free mitochondrial DNA (cfmDNA) and various protein biomarkers, including C-reactive protein, brain natriuretic peptide, and low-density lipoprotein [Citation81].
Due to their small size, microfluidic devices are also uniquely suited to perform single-cell isolation, lysis, and analysis for the purposes of studying single-cell genomics, transcriptomics, proteomics, and metabolomics, particularly in the context of cancer and autoimmune diseases [Citation10, Citation11]. For example, single-cell microfluidic devices have been developed for the isolation and analysis of CTCs for early diagnosis and prognosis of cancer patients [Citation82]. These platforms offer potential alternative methods that are lower in cost as well as higher in sensitivity and efficiency compared to the current FDA-approved CTC detection system, CELLSEARCH® (Menarini Group, Florence, Italy) [Citation82]. Microfluidic technology can also be applied for detection of cell-free DNA (cfDNA) using microfluidic droplet digital PCR and quantification of protein biomarkers using microfluidic digital or analog immunoassays for cancer diagnostics [Citation83]. Recently, microfluidic devices for single immune cell analysis have been developed and successfully implemented in research settings [Citation84]. Ultimately, single-cell-based microfluidic devices have the potential to revolutionize laboratory medicine by providing more personalized diagnostic test results, thereby paving the way for precision medicine.
In recent years, artificial intelligence has been incorporated into microfluidic technology [Citation85]. In particular, deep learning architecture, which is a subset of machine learning, has applications in cell classification (e.g. microfluidic label-free flow cytometry), DNA base calling, and cell segmentation [Citation86]. The addition of artificial intelligence and communication tools to microfluidic devices will facilitate their automation. Furthermore, merging communication technologies with microfluidic devices has led to the eHealth movement, as discussed in the section on Electronic Tools.
Microfluidic devices offer many advantages over conventional laboratory techniques, including low cost, low sample volume, low reagent volume, high throughput, high parallelization, high control over various testing conditions, as well as fast response time and diagnosis [Citation76]. Additionally, these devices are often user-friendly and require little technical training. These advantages make microfluidic LOC devices optimal for POCT, particularly in low-resource settings where highly trained technicians and/or expensive equipment are unavailable, as discussed further within the section on POCT.
Challenges and considerations of microfluidic devices
One of the main barriers to utilizing microfluidic devices, especially for POCT, is the requirement for external sample preparation and/or reagent addition. However, fully integrated systems are now available, such as the lab-on-a-disc (LOAD), which is a centrifugal microfluidic device that can extract, amplify, and detect nucleic acids for diagnostic purposes [Citation87]. Other fully integrated microfluidic devices are also available for ‘sample-to-answer’ qualitative, quantitative, and digital nucleic acid testing, facilitating rapid diagnosis of infectious diseases and/or pathogens, genetic diseases, and oncogenes at the POC [Citation88]. Another major barrier in implementing microfluidic devices in clinical practice is the lack of clinical validation. Additionally, there is a lack of discussion and consensus on how to standardize these devices for integration with external analysis systems, which is essential for their commercialization [Citation89]. Further clinical studies and collaborative discussions across disciplines will help improve widespread adoption of microfluidic devices in clinical care.
Point-of-care testing
In recent decades, POCT has become widely adopted in clinical care due to its unique ability to rapidly turn around critical laboratory test results. Although there was prominent hesitation from the medical community regarding the accuracy and precision of these novel devices, recent technological advances have provided confidence in their performance and the added clinical utility of POCT has since been widely reported [Citation90]. POCT devices can range from wearable devices to portable handheld or benchtop systems. These analyzers employ various analytical techniques to assess key laboratory parameters and turn around test results in often <1 min, with impressive specificity and sensitivity.
Diagnostic applications and recent analytical advancements
To facilitate multi-plex analysis, many POCT devices use cartridges with microfluidic technology. For example, the i-STAT® Alinity™ (Abbott Diagnostics) offers various cartridges with distinct diagnostic test menus, including hematology, cardiac, blood gas, or chemistry assays. These cartridges contain test reagents located on biosensor chips (i.e. ion selective electrodes) made from thin film technology, which are microfabricated onto silicon chips. These devices require only 2–3 drops of blood to generate a potentiometric signal that is converted into the test result. The small sample requirement is another key advantage of POCT over traditional phlebotomy, especially in pediatric and neonatal populations. As previously discussed, LOC technology is integral to POCT and has had demonstrative value during the ongoing COVID-19 pandemic [Citation9]. In addition, microfluidic-based LOC assays have recently been developed for the POC detection of Zika virus, with demonstrated clinical utility and accuracy [Citation91].
Microfluidic-based lateral flow assays have also had a prominent role in providing rapid SARS-CoV-2 antigen testing. The Panbio™ COVID-19 Ag Rapid Test Device (Abbott Diagnostics), for example, uses a membrane strip pre-coated with immobilized anti-SARS-CoV-2 antibody and mouse monoclonal anti-chicken IgY on the test and control line, respectively. For a patient with a positive result, a test line will form due to the presence of human IgG specific to SARS-CoV-2 Ag gold conjugate and anti-SARS-CoV-2 antibody. This methodology has been applied by several additional POC COVID-19 assays [Citation92–95] and other clinical tests [Citation96–102] to date.
NAATs are another emerging application for microfluidic-based POCT for molecular diagnostics. For example, the rapid detection of pathogens in infected wounds is imperative for optimal clinical outcomes, and as wound exudate can be extracted non-invasively, this presents a unique opportunity for POCT. Brunauer et al. recently developed a nucleic acid lateral flow assay for the direct detection of isothermally amplified DNA with minimal sample preparation [Citation12]. Though still in its early stages, evidence to date has been promising and certainly demonstrates potential for reliable POCT in pathogen detection and subsequent target-oriented therapy.
One additional and novel area of POCT application is for MS- and NMR-based laboratory tests. Technological advancements in ambient ionization and in LC have facilitated direct and rapid analysis by MS with little pre-treatment requirements [Citation103]. For MS POCT, ambient ionization occurs under open-air as opposed to suction conditions [Citation103] and sample preparation is drastically simplified [Citation104–106]. Several devices are currently in development and commercialization [Citation107–109]. For example, the Mini 12 is a POC ion trap with a discontinuous atmospheric pressure introduction valve containing paper spray as an ambient ion source that is evidenced to detect amitriptyline in whole blood [Citation107] and synthetic cannabinoids in blood and urine [Citation110], underscoring the clinical utility for MS POCT in drug screening. Additionally, a triple quadrupole with a differential pumping interface has been developed to facilitate the quantification of small molecules [Citation111, Citation112]. Few studies have reported the application of NMR in POCT, although the T2 Biosystems platform (T2MR®) is an NMR POCT system that has been FDA-cleared for the detection of candida in sepsis [Citation113]. Future research avenues for potential NMR applications include hemostasis testing at the POC [Citation114, Citation115].
Modern POCT devices have profited off the substantial advancements made in analytical chemistry, and together with innovative bioengineering, have enabled the miniaturization of many traditional core laboratory tests (). Though POC tests are not designed to replace the core laboratory, technological advancements within these devices and their applications will help narrow the clinical-laboratory interface and improve timely clinical decision-making. In addition, many POCT devices have been linked to electronic tools, such as smartphone-based devices, improving the accessibility of test result interpretation. The application of these devices is discussed in further detail below.
Electronic tools
Smartphone-based devices
Advancements in molecular analysis, biosensors, mathematical algorithms, microfabrication, 3 D printing, and microfluidics have enabled smartphones to serve as read-out platforms for diagnostic tests, bringing complex and expensive tests from the central clinical laboratory to the POC due to their portability, connectivity, and cost-effectiveness [Citation116]. In particular, applications of smartphone-based diagnostics include infectious disease (e.g. malaria), pulmonology (e.g. cystic fibrosis), virology (e.g. HIV, hepatitis, flu), microbiology (e.g. gastroenteritis), oncology (e.g. skin, prostate, and colorectal cancer), endocrinology (e.g. hormones), hematology (e.g. anemia, leukemia), and more [Citation116]. Recently, there has been an emergence of smartphone-based devices for quantitative nucleic acid detection using paper-based sensors, microfluidic chips, and digital droplet assays, particularly for viral detection [Citation117]. As previously mentioned, the merge of communication technologies with microfluidic devices has facilitated the eHealth movement [Citation118]. For example, Bluetooth® or WiFi® technology can be incorporated into portable or wearable microfluidic devices, enabling data transmission from the device to a centralized location for real-time, continuous monitoring at the POC [Citation118].
Wearable devices
Wearable diagnostic devices are particularly relevant in the field of laboratory medicine, as they facilitate the measurement of biomarkers in various fluids, including sweat, tears, saliva, and interstitial fluid [Citation119]. These wearable devices are becoming more accurate, reliable, and efficient, thereby substantially improving their clinical utility and opportunity for commercialization [Citation119]. In fact, Ates et al. recently suggested wearable devices for early detection of asymptomatic and pre-symptomatic cases of COVID-19 [Citation120]. As with other types of microfluidic devices, clinical validation and integration with external systems must be considered for these devices to have true clinical utility and commercialization potential.
Concluding remarks
In conclusion, laboratory medicine continues to be driven forward by advancements in innovative technologies, which ultimately improve the preventative, diagnostic, prognostic, and monitoring capabilities of the laboratory. Extensive progress has been made in the automation of core laboratory analyzers, and with adequate training and education, evidence to date supports several key benefits to the laboratory environment, including improved clinical workflow, reduced TAT and cost, and increased overall efficiency. Novel advancements in analytical techniques, namely, genetic sequencing, NMR, MS, and microtechnology, have also defined the current era of laboratory medicine and the expansive role of the laboratory within healthcare provision. POCT has also benefited from these novel technologies and adapted them to a portable size to narrow the clinical-laboratory interface and directly improve patient outcomes. Overall, this culture of innovation within laboratory medicine has highlighted the integral role of laboratory testing in clinical decision-making. However, the mass amount of data produced from these novel techniques must be harnessed by AI technologies to see its true potential. Indeed, at the intersection of advanced technologies that produce medical big data and the information technology required to analyze it, the face of laboratory medicine may change drastically, to improve both laboratory processes and patient care. Furthermore, advanced laboratory analytics go beyond impacting general patient care by enabling the development of precision and personalized medicine. Advancements in omics and single-cell microtechnology have significantly aided our progress towards this future patient-centered approach, and exciting progress has already been established in the field of oncology. However, advanced AI is nonetheless required to meet this promise. Future work should seek to expand the clinical applications of AI and precision medicine across disciplines in the context of laboratory medicine as well as assess considerations for clinical implementation.
Acknowledgements
were created with BioRender.com.
Disclosure statement
The authors report no conflict of interest.
Data availability statement
Data sharing is not applicable to this article as no new data were created or analyzed in this study.
Additional information
Funding
References
- Genzen JR, Burnham C-AD, Felder RA, et al. Challenges and opportunities in implementing total laboratory automation. Clin Chem. 2018;64(2):259–264.
- Lou AH, Elnenaei MO, Sadek I, et al. Evaluation of the impact of a total automation system in a large core laboratory on turnaround time. Clin Biochem. 2016;49(16-17):1254–1258.
- Armbruster DA, Overcash DR, Reyes J. Clinical chemistry laboratory automation in the 21st Century – Amat Victoria curam (victory loves careful preparation). Clin Biochem Rev. 2014;35(3):143–153.
- Bourbeau PP, Ledeboer NA. Automation in clinical microbiology. J Clin Microbiol. 2013;51(6):1658–1665.
- Chambers DC, Carew AM, Lukowski SW, et al. Transcriptomics and single-cell RNA-sequencing. Respirology. 2019;24(1):29–36.
- Li X, Wang W, Chen J. Recent progress in mass spectrometry proteomics for biomedical research. Sci China Life Sci. 2017;60(10):1093–1113.
- Dettmer K, Aronov PA, Hammock BD. Mass spectrometry-based metabolomics. Mass Spectrom Rev. 2007;26(1):51–78.
- Nicholson K, Lindon JC, Holmes E . ‘Metabonomics’: understanding the metabolic responses of living systems to pathophysiological stimuli via multivariate statistical analysis of biological NMR spectroscopic data. Xenobiotica. 1999;29(11):1181–1189.
- Lin Q, Wen D, Wu J, et al. Microfluidic immunoassays for sensitive and simultaneous detection of IgG/IgM/antigen of SARS-CoV-2 within 15 min. Anal Chem. 2020;92(14):9454–9458.
- Hochstetter A. Lab-on-a-chip technologies for the single cell level: separation, analysis, and diagnostics. Micromachines. 2020;11(5):468.
- Xu X, Wang J, Wu L, et al. Microfluidic single-cell omics analysis. Small. 2020;16(9):1903905–1903917.
- Brunauer A, Verboket RD, Kainz DM, et al. Rapid detection of pathogens in wound exudate via nucleic acid lateral flow immunoassay. Biosensors. 2021;11(3):74.
- Ma C, Wang X, Wu J, et al. Real-world big-data studies in laboratory medicine: current status, application, and future considerations. Clin Biochem. 2020;84:21–30.
- Rosenfeld L. Clinical chemistry since 1800: growth and development. Clin Chem. 2002;48(1):186–197.
- Rosenfeld L. A golden age of clinical chemistry: 1948–1960. Clin Chem. 2000;46(10):1705–1714.
- Kricka LJ, Savory J. International year of chemistry 2011. A guide to the history of clinical chemistry. Clin Chem. 2011;57:1118–1126.
- Marzinke MA. Chapter 14 – Laboratory automation. In: Clarke W, Marzinke MA, editors. Contemporary practice in clinical chemistry. 4th ed. UK & USA: Academic Press; 2020. p. 235–246. https://doi.org/https://doi.org/10.1016/B978-0-12-815499-1.00014-4.
- Miler M, Gabaj NN, Dukic L, et al. Systems-level quality improvement key performance indicators to measure improvement after implementation of total laboratory automation abbott accelerator a3600. J Med Syst. 2018;42(2):28.
- Mutters NT, Hodiamont CJ, de Jong MD, et al. Performance of kiestra total laboratory automation combined with MS in clinical microbiology practice. Ann Lab Med. 2014;34(2):111–117. [24624346]
- Faron ML, Buchan BW, Relich RF, et al. Evaluation of the WaSPLAb segregation software to automatically analyze urine cultures using routine blood and MacConkey agars. J. Clin. Microbiol. 2020;58(4):e01683-19. https://doi.org/https://doi.org/10.1128/JCM.01683-19.
- Lippi G, Da Rin G. Advantages and limitations of total laboratory automation: a personal overview. Clin Chem Lab Med. 2019;57(6):802–811.
- Bailey AL, Ledeboer N, Burnham CAD. Clinical microbiology is growing up: the total laboratory automation revolution. Clin Chem. 2019;65(5):634–643.
- Giavarina D, Cappelletti A, Carta M. Improved workflow in routine-stat integration. Clin Chim Acta. 2019;493(Supplement 1):S52–S53.
- Novak SM, Marlowe EM. Automation in the clinical microbiology laboratory. Clin Lab Med. 2013;33(3):567–588.
- Burckhardt I. Laboratory automation in clinical microbiology. Bioeng. 2018;5(4):102.
- Thomson RB, McElvania E. Total laboratory automation: what is gained, what is lost, and who can afford it? Clin Lab Med. 2019;39(3):371–389.
- Croxatto A, Dijkstra K, Prod’hom G, et al. Comparison of inoculation with the InoqulA and WASP automated systems with manual inoculation. J Clin Microbiol. 2015;53(7):2298–2307.
- Greub G, Prod’hom G. Automation in clinical bacteriology: what system to choose? Clin Microbiol Infect. 2011;17(5):655–660.
- Croxatto A, Prod’hom G, Faverjon F, et al. Laboratory automation in clinical bacteriology: what system to choose? Clin Microbiol Infect. 2016;22(3):217–235.
- Archetti C, Montanelli A, Finazzi D, et al. Clinical laboratory automation: a case study. J Public Health Res. 2017;6(1):881–836.
- Da Rin G, Zoppelletto M, Lippi G. Integration of diagnostic microbiology in a model of total laboratory automation. Lab Med. 2016;47(1):73–82.
- Croxatto A, Marcelpoil R, Orny C, et al. Towards automated detection, semi-quantification and identification of microbial growth in clinical bacteriology: a proof of concept. Biomed J. 2017;40(6):317–328.
- Samuel L, Novak-Weekley S. The role of the clinical laboratory in the future of health care: lean microbiology. J Clin Microbiol. 2014;52(6):1812–1817.
- Dauwalder O, Landrieve L, Laurent F, et al. Does bacteriology laboratory automation reduce time to results and increase quality management? Clin Microbiol Infect. 2016;22(3):236–243.
- Graham M, Tilson L, Streitberg R, et al . Improved standardization and potential for shortened time to results with BD Kiestra™ total laboratory automation of early urine cultures: a prospective comparison with manual processing. Diagn Microbiol Infect Dis. 2016;86(1):1–4.
- Theparee T, Das S, Thomson RB. Total laboratory automation and matrix-assisted laser desorption ionization–time of flight mass spectrometr improve turnaround times in the clinical microbiology laboratory: a retrospective analysis. J. Clin. Microbiol. 2018;56(1):e01242–17.
- Ledeboer NA, Dallas SD. The automated clinical microbiology laboratory: fact or fantasy? J Clin Microbiol. 2014;52(9):3140–3146.
- O’Brien JM, Kumar A, Metersky ML. Does Value-Based purchasing enhance quality of care and patient outcomes in the ICU? Crit Care Clin. 2013;29(1):91–112.
- Yu HYE, Lanzoni H, Steffen T, et al . Improving laboratory processes with Total Laboratory Automation. Lab Med. 2019;50(1):96–102.
- Crocker JB, Lee-Lewandrowski E, Lewandrowski N, et al. Implementation of point-of-Care testing in an ambulatory practice of an academic medical center. Am J Clin Pathol. 2014;142(5):640–646.
- Žitnik IP, Černe D, Mancini I, et al. Marc and behalf of EFLM/ESPT working group of personalised laboratory medicine, personalized laboratory medicine: a patient-centered future approach. Clin Chem Lab Med. 2018;56(12):1981–1991.
- Al-Mozaini MA, Mansour MK. Personalized medicine. Is it time for infectious diseases? Saudi Med J. 2016;37(12):1309–1311.
- Wang Y, Navin NE. Advances and applications of single-cell sequencing technologies. Mol Cell. 2015;58(4):598–609.
- Hu Y, An Q, Sheu K, et al. Single cell multi-omics technology: methodology and application. Front Cell Dev Biol. 2018;6:28.
- Van Gelder RN, von Zastrow ME, Yool A, et al. Amplified RNA synthesized from limited quantities of heterogeneous cDNA. Proc Natl Acad Sci USA. 1990;87(5):1663–1667.
- Dean FB, Hosono S, Fang L, et al. Comprehensive human genome amplification using multiple displacement amplification. Proc Natl Acad Sci USA. 2002;99(8):5261–5266.
- Tang F, Barbacioru C, Wang Y, et al. mRNA-Seq whole-transcriptome analysis of a single cell. Nat Methods. 2009;6(5):377–382.
- Mardis ER . A decade’s perspective on DNA sequencing technology. Nature. 2011;470(7333):198–203.
- Navin N, Kendall J, Troge J, et al . Tumour evolution inferred by single-cell sequencing. Nature. 2011;472(7341):90–94.
- de Anda-Jáuregui G, Hernández-Lemus E. Computational oncology in the multi-omics era: state of the art, front. Front Oncol. 2020;10:423.
- Gawad C, Koh W, Quake SR. Single-cell genome sequencing: current state of the science. Nat Rev Genet. 2016;17(3):175–188.
- Newman AM, Bratman SV, To J, et al. An ultrasensitive method for quantitating circulating tumor DNA with broad patient coverage. Nat Med. 2014;20(5):548–554.
- Taiwo O, Wilson GA, Morris T, et al. Methylome analysis using MeDIP-seq with low DNA concentrations. Nat Protoc. 2012;7(4):617–636.
- Shen SY, Burgener JM, Bratman SV, et al. Preparation of cfMeDIP-seq libraries for methylome profiling of plasma cell-free DNA. Nat Protoc. 2019;14(10):2749–2780.
- Wan JCM, Massie C, Garcia-Corbacho J, et al. Liquid biopsies come of age: towards implementation of circulating tumour DNA. Nat Rev Cancer. 2017;17(4):223–238.
- Merker JD, Oxnard GR, Compton C, et al. Circulating tumor DNA analysis in patients with cancer: American Society of Clinical Oncology and College of American Pathologists joint review. J Clin Oncol. 2018;36(16):1631–1641.
- Guardant Health Providing Liquid Biopsy for Japanese GI Cancer Basket Trial | Genomeweb. (2018). [accessed 2021 Jul 14]. Available from: https://www.genomeweb.com/cancer/guardant-health-providing-liquid-biopsy-japanese-gi-cancer-basket-trial#.XMMi5jBKiw5
- Mok TSK, Gadgeel S, Kim ES, et al. Blood first line ready screening trial (B-F1RST) and blood first assay screening trial (BFAST) enable clinical development of novel blood-based biomarker assays for tumor mutational burden (TMB) and somatic mutations in 1L advanced or metastatic NSCLC. Ann. Oncol. 2017;28:v494–v495.
- Bratman SV, Yang SYC, Iafolla MAJ, et al. Personalized circulating tumor DNA analysis as a predictive biomarker in solid tumor patients treated with pembrolizumab. Nat Cancer. 2020;1(9):873–881.
- Yang X, Kui L, Tang M, et al. High-throughput transcriptome profiling in drug and biomarker discovery. Front Genet. 2020;11:19.
- Islam S, Kjällquist U, Moliner A, et al. Characterization of the single-cell transcriptional landscape by highly multiplex RNA-seq. Genome Res. 2011;21(7):1160–1167.
- Klein AM, Mazutis L, Akartuna I, et al. Droplet barcoding for single-cell transcriptomics applied to embryonic stem cells. Cell. 2015;161(5):1187–1201.
- Macosko EZ, Basu A, Satija R, et al. Highly parallel genome-wide expression profiling of individual cells using nanoliter droplets. Cell. 2015;161(5):1202–1214.
- Cheng YH, Chen YC, Lin E, et al. Hydro-Seq enables contamination-free high-throughput single-cell RNA-sequencing for circulating tumor cells. Nat Commun. 2019;10(1):2163. https://doi.org/https://doi.org/10.1038/S41467-019-10122-2.
- Ombrone D, Giocaliere E, Forni G, et al. Expanded newborn screening by mass spectrometry: new tests, future perspectives. Mass Spectrom Rev. 2016;35(1):71–84.
- Millington DS, Kodo N, Norwood DL, et al. Tandem mass spectrometry: a new method for acylcarnitine profiling with potential for neonatal screening for inborn errors of metabolism. J Inherit Metab Dis. 1990;13(3):321–324.
- Arnold GL. Inborn errors of metabolism in the 21st century: past to present. Ann Transl Med. 2018;6(24):467–467.
- Xu F, Tavintharan S, Sum CF, et al. Metabolic signature shift in type 2 diabetes mellitus revealed by mass spectrometry-based metabolomics. J Clin Endocrinol. Metab. 2013;98(6):E1060–E1065.
- Emwas A-HM. The strengths and weaknesses of NMR spectroscopy and mass spectrometry with particular focus on metabolomics research. Methods Mol Biol. 2015;1277:161–193. https://doi.org/https://doi.org/10.1007/978-1-4939-2377-9_13.
- Dunn WB, Broadhurst DI, Atherton HJ, et al. Systems level studies of mammalian metabolomes: the roles of mass spectrometry and nuclear magnetic resonance spectroscopy. Chem Soc Rev. 2011;40(1):387–426.
- Emwas A-HM, Roy R, McKay RT, et al. NMR spectroscopy for metabolomics research. Metabolites. 2019;9(7):123.
- Marshall DD, Lei S, Worley B, et al . Combining DI-ESI-MS and NMR datasets for metabolic profiling. Metabolomics. 2015;11(2):391–402.
- Jensen SO, van Hal SJ. Personalized medicine and infectious disease management. Trends Microbiol. 2017;25(11):875–876.
- Rose SMS-F, Contrepois K, Moneghetti KJ, et al. A longitudinal big data approach for precision health. Nat Med. 2019;25(5):792–804.
- Manz A, Graber N, Widmer HM. Miniaturized total chemical analysis systems: a novel concept for chemical sensing. Sensors Actuators B Chem. 1990;1(1-6):244–248.
- Whitesides GM. The origins and the future of microfluidics. Nature. 2006;442(7101):368–373.
- Niculescu AG, Chircov C, Bîrcă AC, et al. Fabrication and applications of microfluidic devices: a review. IJMS. 2021;22(4):2011–2026.
- Yang Y, Chen Y, Tang H, et al. Microfluidics for biomedical analysis. Small. 2020;4(4):1900451.
- Zhu H, Fohlerová Z, Pekárek J, et al. Recent advances in lab-on-a-chip technologies for viral diagnosis. Biosens Bioelectron. 2020;153:112041
- Tymm C, Zhou J, Tadimety A, et al. Scalable COVID-19 detection enabled by lab-on-chip biosensors. Cel Mol Bioeng. 2020;13(4):313–329.
- Dinter F, Burdukiewicz M, Schierack P, et al. Simultaneous detection and quantification of DNA and protein biomarkers in spectrum of cardiovascular diseases in a microfluidic microbead chip. Anal Bioanal Chem. 2019;411(29):7725–7735.
- Lin Z, Luo G, Du W, et al. Recent advances in microfluidic platforms applied in cancer metastasis: circulating tumor cells’ (CTCs) isolation and tumor-on-a-chip. Small. 2020;16(9):1903899.
- Garcia-Cordero JL, Maerkl SJ. Microfluidic systems for cancer diagnostics. Curr Opin Biotechnol. 2020;65:37–44.
- Jammes FC, Maerkl SJ. How single-cell immunology is benefiting from microfluidic technologies, microsystems nanoeng. Microsyst Nanoeng. 2020;6:45.
- Isozaki A, Harmon J, Zhou Y, et al. AI on a chip. Lab Chip. 2020;20(17):3074–3090.
- Riordon J, Sovilj D, Sanner S, et al. Deep learning with microfluidics for biotechnology. Trends Biotechnol. 2019;37(3):310–324.
- Van Nguyen H, Nguyen VD, Nguyen HQ, et al. Nucleic acid diagnostics on the total integrated lab-on-a-disc for point-of-care testing. Biosens Bioelectron. 2019;141:111466.
- Li Z, Bai Y, You M, et al. Fully integrated microfluidic devices for qualitative, quantitative and digital nucleic acids testing at point of care. Biosens Bioelectron. 2021;177:112952
- Convery N, Gadegaard N. 30 years of microfluidics. Micro Nano Eng. 2019;2:76–91.
- Lewandrowski EL, Lewandrowski K. Implementing point-of-care testing to improve outcomes. J Hosp Adminis. 2013;2(2):125–132.
- Sharma S, Kabir MA, Asghar W. Lab-on-a-chip zika detection with reverse transcription loop-mediated isothermal amplification-based assay for point-of-care settings. Arch Pathol Lab Med. 2020;144(11):1335–1343.
- Liu D, Shen H, Zhang Y, et al. A microfluidic-integrated lateral flow recombinase polymerase amplification (MI-IF-RPA) assay for rapid COVID-19 detection. Lab Chip. 2021;21(10):2019–2026.
- Zheng Y-Z, Chen J-T, Li J, et al. Reverse transcription recombinase-aided amplification assay with lateral flow dipstick assay for rapid detection of 2019 novel coronavirus. Front Cell Infect Microbiol. 2021;11:24.
- Conklin SE, Martin K, Manabe YC, et al. Evaluation of serological SARS-CoV-2 lateral flow assays for rapid point-of-care testing. J. Clin. Microbiol. 2020;59(2):e02020-20. https://doi.org/https://doi.org/10.1128/JCM.02020-20.
- Charlton CL, Kanji JN, Johal K, et al. Evaluation of six commercial mid-to high-volume antibody and six point-of-care lateral flow assays for detection of SARS-CoV-2 antibodies. J Clin Microbiol. 2020;58(10):e01361-20. https://doi.org/https://doi.org/10.1128/JCM.01361-20.
- Kim H, Chung D-R, Kang M. A new point-of-care test for the diagnosis of infectious diseases based on multiplex lateral flow immunoassays. Analyst. 2019;144(8):2460–2466.
- Lin B, Guan Z, Song Y, et al. Lateral flow assay with pressure meter readout for rapid point-of-care detection of disease-associated protein. Lab Chip. 2018;18(6):965–970.
- Dalirirad S, Steckl AJ. Aptamer-based lateral flow assay for point of care cortisol detection in sweat. Sensors Actuators B Chem. 2019;283:79–86.
- Gong Y, Zheng Y, Jin B, et al. A portable and universal upconversion nanoparticle-based lateral flow assay platform for point-of-care testing. Talanta. 2019;201:126–133.
- Li Z, Chen H, Wang P. Lateral flow assay ruler for quantitative and rapid point-of-care testing. Analyst. 2019;144(10):3314–3322.
- Deng J, Yang M, Wu J, et al. A self-contained chemiluminescent lateral flow assay for point-of-care testing. Anal Chem. 2018;90(15):9132–9137.
- Ru Choi J, Hu J, Gong Y, et al. An integrated lateral flow assay for effective DNA amplification and detection at the point of care. Analyst. 2016;141(10):2930–2939.
- Ferreira CR, Yannell KE, Jarmusch AK, et al. Ambient ionization mass spectrometry for point-of-care diagnostics and other clinical measurements. Clin Chem. 2016;62(1):99–110.
- Pulliam CJ, Wei P, Snyder DT, et al. Rapid discrimination of bacteria using a miniature mass spectrometer. Analyst. 2016;141(5):1633–1636.
- Kumano S, Sugiyama M, Yamada M, et al. Probe heating method for the analysis of solid samples using a portable mass spectrometer. Mass Spectrom (Tokyo)). 2015;4(1):A0038.
- Gómez-Ríos GA, Vasiljevic T, Gionfriddo E, et al. Towards on-site analysis of complex matrices by solid-phase microextraction-transmission mode coupled to a portable mass spectrometer via direct analysis in real time. Analyst. 2017;142(16):2928–2935.
- Li L, Chen T-C, Ren Y, et al. Mini 12, miniature mass spectrometer for clinical and other applications-introduction and characterization. Anal Chem. 2014;86(6):2909–2916.
- Kaiser RE, Graham Cooks R, Stafford GC, et al. Operation of a quadrupole ion trap mass spectrometer to achieve high mass/charge ratios. Int J Mass Spectrom Ion Process. 1991;106:79–115.(91)85013-C.
- Lammert SA, Rockwood AA, Wang M, et al. Miniature toroidal radio frequency ion trap mass analyzer. J Am Soc Mass Spectrom. 2006;17(7):916–922.
- Ma Q, Bai H, Li W, et al. Rapid analysis of synthetic cannabinoids using a miniature mass spectrometer with ambient ionization capability. Talanta. 2015;142:190–196.
- Zhai Y, Feng Y, Wei Y, et al. Development of a miniature mass spectrometer with continuous atmospheric pressure interface. Analyst. 2015;140(10):3406–3414.
- Wright S, Malcolm A, Wright C, et al. A microelectromechan ical systems-enabled, miniature triple quadrupole mass spectrometer. Anal Chem. 2015;87(6):3115–3122.
- Issadore D, Min C, Liong M, et al. Miniature magnetic resonance system for point-of-care diagnostics. Lab Chip. 2011;11(13):2282–2287.
- Cuker A, Husseinzadeh H, Lebedeva T, et al. Rapid evaluation of platelet function with T2 magnetic resonance. Am J Clin Pathol. 2016;146(6):681–693.
- ]Skewis LR, Lebedeva T, Papkov V, et al . T2 magnetic resonance: a diagnostic platform for studying integrated hemostasis in whole blood-proof of concept. Clin Chem. 2014;60(9):1174–1182.
- Hernández-Neuta I, Neumann F, Brightmeyer J, et al. Smartphone-based clinical diagnostics: towards democratization of evidence-based health care. J Intern Med. 2019;285(1):19–39.
- Rajendran VK, Bakthavathsalam P, Bergquist PL, et al . Smartphone technology facilitates point-of-care nucleic acid diagnosis: a beginner’s guide. Crit Rev Clin Lab Sci. 2021;58(2):77–100.
- Mejía-Salazar JR, Cruz KR, Vásques EMM, et al. Microfluidic point-of-care devices: new trends and future prospects for eHealth diagnostics. Sensors. 2020;20(7):1951–1919.
- Kim J, Campbell AS, de Ávila BEF, et al. Wearable biosensors for healthcare monitoring. Nat Biotechnol. 2019;37(4):389–406.
- Ates HC, Yetisen AK, Güder F, et al. Wearable devices for the detection of COVID-19. Nat Electron. 2021;4(1):13–14.