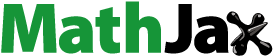
Abstract
Background
Hereditary transthyretin (TTR) amyloidosis (ATTRv) initially presents as a polyneuropathy and/or a cardiomyopathy. Central nervous system (CNS) pathology in ATTRv amyloidosis, including focal neurological episodes, dementia, cerebrovascular bleeding, and seizures, appears around a decade later. Wild-type (WT) TTR amyloidosis (ATTRwt) causes a cardiomyopathy. CNS pathology risk likely also increases in these patients as cardiomyopathy progresses. Herein, we study tafamidis-mediated TTR kinetic stabilisation in cerebrospinal fluid (CSF).
Methods
Varying tafamidis concentrations (50–1000 nM) were added to CSF from healthy donors or ATTRv patients, and TTR stabilisation was measured via the decrease in dissociation rate.
Results
Tafamidis meglumine (Vyndaqel) can be dosed at 20 or 80 mg QD. The latter dose is bioequivalent to a 61 mg QD dose of tafamidis free acid (Vyndamax). The tafamidis CSF concentration in ATTRv patients on 20 mg Vyndaqel is ∼125 nM. By linear extrapolation, we expect a CSF concentration of ∼500 nM at the higher dose. When tafamidis is added to healthy donor CSF at 125 or 500 nM, the WT TTR dissociation rate decreases by 42% or 87%, respectively.
Conclusions
Tafamidis stabilises TTR in CSF to what is likely a clinically meaningful extent at CSF concentrations achieved by the normal tafamidis dosing regimen.
Introduction
Transthyretin (TTR) amyloidosis is a systemic autosomal dominant or sporadic degenerative disease [Citation1]. Genetic and pharmacological evidence suggest that tetramer dissociation, monomer misfolding, and aggregation of newly synthesised TTR cause the dysfunction of and ultimately the loss of post mitotic tissues [Citation1–5]. Hereditary TTR (ATTRv; v stands for variant) amyloidosis presents as a principal axonal polyneuropathy, with autonomic nervous system dysfunction, often with variable deterioration of other organ systems including the heart, kidneys, and gastrointestinal tract [Citation6–10]. Alternatively, ATTRv amyloidosis can present as a principal cardiomyopathy with dysautonomia, often with less prominent sensory polyneuropathy [Citation4,Citation11,Citation12]. Central nervous system (CNS) manifestations, which generally appear later in the course of ATTRv amyloidosis, include focal neurological episodes, dementia, cerebrovascular bleeding, seizures, and irritability [Citation13–20]. Sporadic WT TTR aggregation typically results in cardiomyopathy and, without treatment, can lead to congestive heart failure within a decade [Citation4,Citation11,Citation21,Citation22]. Approximately 70% of WT ATTR cardiomyopathy patients have sensory polyneuropathy, generally without motor involvement [Citation12]. Many wild-type ATTR cardiomyopathy patients report cerebrovascular bleeds that appear to be TTR aggregation-dependent; this hypothesis merits further scrutiny [Citation23].
Certain TTR mutations in heterozygotes form extremely destabilised tetramers comprising mutant and WT subunits; aggregation from these misfolded TTR subunits leads to an initial CNS presentation [Citation24,Citation25]. The tetramers rich in variant subunits are not efficiently secreted by the liver into the blood [Citation26]. Ironically however, they are secreted at near WT TTR levels by the choroid plexus (e.g. Asp18Gly and Ala25Thr) into the cerebrospinal fluid (CSF) [Citation26,Citation27]. This leads to TTR leptomeningeal amyloidosis/cerebral amyloid angiopathy as the initial TTR amyloid disease presentation [Citation7,Citation18,Citation20,Citation27–30].
Transthyretin is a 55-kDa tetrameric protein consisting of four β-sheet rich subunits, each comprising 127 amino acid residues [Citation31]. The TTR tetramer binds and transports thyroxine and the holo-retinol binding protein. Binding of either slows TTR tetramer dissociation [Citation31–34]. Following rate-limiting tetramer dissociation and partial monomer denaturation, TTR can aggregate into numerous non-native structures, including cross-β-sheet amyloid fibrils. The soluble non-native TTR structures seem to be the main driver of degenerative phenotypes [Citation1,Citation35]. Many pathogenic mutations destabilise the resulting TTR tetramers comprising mutant and WT TTR subunits, causing faster dissociation and leading to misfolding, aggregation, and initial degenerative phenotypes such as polyneuropathy (earlier called familial amyloid polyneuropathy (FAP)), or cardiomyopathy, or neuropathic leptomeningeal amyloidosis, depending on the identity of the TTR mutation [Citation26]. Although more than 130 TTR mutations are associated with degenerative pathology, the most common mutation worldwide is Val30Met (V30M, p.TTRV50M). The V30M mutation causes a debilitating hereditary neuropathy involving sensory, motor, and autonomic nervous system dysfunction with many patients manifesting symptoms such as sensorimotor polyneuropathy; autonomic dysfunction; and gastrointestinal, heart, and kidney dysfunction [Citation6–9].
Liver transplantation was one of the first effective treatments for V30M polyneuropathy [Citation36,Citation37]. In this TTR gene replacement approach that is only efficacious in the periphery, a liver producing both mutant and wild-type (WT) TTR is replaced with a liver producing only WT TTR. This procedure slows or stops the progression of hereditary polyneuropathy in the majority of patients by eliminating the less stable WT/V30M heterotetramers and the V30M homotetramer in the blood [Citation37,Citation38]. Subsequently discovered pharmacological strategies that slow or stop TTR amyloid disease progression in the majority of hereditary ATTR polyneuropathy patients include kinetic stabilisation of the TTR tetramer by the drug tafamidis [Citation3–5] and TTR mRNA degradation, either by anti-sense oligonucleotides or siRNA-based drugs [Citation39,Citation40].
Several groups have reported CNS pathology developing in V30M polyneuropathy patients [Citation13,Citation14,Citation25,Citation30], especially after being treated by liver transplantation ≈ 14 years earlier [Citation16–18,Citation30,Citation41], or in untreated older hereditary polyneuropathy patients exhibiting slow peripheral disease progression [Citation19,Citation20]. The CNS pathology comes from TTR secreted by the choroid plexus aggregating in the CSF and in the brain parenchyma [Citation20,Citation27], causing transitory focal neurological episodes (TFNEs), cognitive dysfunction/dementia, seizures, and cerebrovascular bleeds [Citation16,Citation18–20]. The aggregation of TTR on the CSF facing side of the leptomeningeal vessels appears to be prominent, ultimately compromising vessel integrity [Citation7,Citation13,Citation18,Citation20,Citation30], similar to the pathology of cerebral amyloid angiopathy caused by the aggregation of several different peptides and proteins [Citation18].
Tafamidis is a safe, potent, orally bioavailable TTR kinetic stabiliser that is widely used for the treatment of both ATTRv and wild-type TTR cardiomyopathy [Citation1,Citation3,Citation4]. It is available commercially as Vyndaqel® (20 or 80 mg QD) or Vyndamax® (61 mg QD), which are distinct formulations [Citation42]. Tafamidis selectively binds to TTR tetramers in both plasma and CSF [Citation43,Citation44]. Preferential native state tetramer binding by tafamidis over binding to the TTR dissociative transition state dose-dependently slows the rate-limiting step of TTR aggregation: tetramer dissociation [Citation1,Citation45]. This slowing in tetramer dissociation and aggregation stops or slows the progression of ATTR amyloidosis in the majority of patients [Citation3,Citation4,Citation45–47].
Herein, we reveal the tafamidis concentration requirements for slowing TTR tetramer dissociation (the rate-limiting step of aggregation) in human CSF sourced from homozygotic WT healthy carriers and heterozygotic V30M/WT ATTR polyneuropathy patients. The tafamidis concentration-dependent reduction in TTR tetramer dissociation rate that we report is useful for establishing the oral dose of tafamidis that has the potential to delay or prevent the onset of CNS neuropathy in patients initially presenting with non-CNS symptoms [Citation16–19,Citation41]. Whether the reduction in the rate of TTR tetramer dissociation expected in the CSF of V30M polyneuropathy patients on long-term 20 mg Vyndaqel® therapy (resulting in a 125 nM tafamidis-CSF concentration) will continue to prevent CNS disease onset remains to be determined. Increasing the oral dose of Vyndaqel® to 80 mg QD could quadruple the CSF tafamidis concentration to ≈ 500 nM. This assumes a linear relationship exists between oral dose and CSF exposure. Predicting the relationship between dose and CSF exposure is complex as there are many factors. If blood pharmacokinetics proves to be a dominant determinant, the CSF exposure could be less than linear. The 61 mg Vyndamax® dose is bioequivalent to 80 mg of Vyndaqel® in terms of plasma tafamidis concentration achieved with once daily dosing, albeit with a different formulation [Citation42]. The data herein show that a 500 nM CSF tafamidis concentration affords an 87% decrease in WT TTR dissociation rate in the CSF and a 50% decrease in V30M/WT TTR tetramer dissociation rate in the CSF. While the extent to which TTR must be kinetically stabilised in order to observe the maximal clinical response is currently not known, recent reports suggest that moderate TTR stabilisation translates into a clinical response [Citation48]. Therefore, we envision that the 125 nM and 500 nM CSF concentrations will help slow or stop the onset of CNS pathology.
Materials and methods
CSF sample procurement
Three 5 mL pooled cerebrospinal fluid (CSF) samples originating from healthy donors were purchased from Innovative Research Inc. (Manufacturer No.: IRHUCSF5ML) to perform subunit exchange experiments in biological triplicate. These samples were centrifuged at 18,400 × g for 30 min at 4 °C, filtered through a 0.22 µM PVDF filter, and stored at −80 °C as 1 mL CSF aliquots until conducting the subunit exchange experiments. No information was provided regarding the demographic characteristics of these samples.
In addition, four heterozygous V30M polyneuropathy patient CSF samples previously analysed in Monteiro et al. [Citation43] were used for these experiments. These patients were routinely followed in the Unidade Corino de Andrade in the Neurology Department of the Hospital de Santo Antonio, Porto, Portugal. Three of the samples were from polyneuropathy patients being treated with 20 mg Vyndaqel® (tafamidis) QD (labelled D002, D004, D008; previous ‘tafamidis group’) while one sample was taken from a patient not being treated with tafamidis (labeled D006; previous ‘no tafamidis group’). No information regarding age, gender, or disease status was provided.
Recombinant protein expression and purification
Recombinant wild-type TTR (WT TTR) was expressed and purified from Escherichia coli as previously described [Citation49]. A (His)6 tag was used to accelerate purification and ameliorate minor proteolytic cleavage seen previously with samples purified by ion exchange and size-exclusion chromatography. The (His)6-dual-FLAG tagged Cys10Ala TTR ((His)6-FT2-C10A TTR) plasmid was transformed into BL21(DE3) Escherichia coli by heat shock and the resulting colonies were used to grow 2 × 2 mL LB starter cultures containing 100 µL/mL Kanamycin. The starter cultures were grown for 2 h at 37 °C with shaking (180 rpm) prior to transferring to 2 × 25 mL cultures. After an additional 2 h of growth under the same conditions, the 25 mL cultures were transferred to 2 × 1-liter cultures and grown until an O.D. of 0.5 was reached, at which point the bacterial cultures were induced with isopropyl β-D-1-thio-glactopyranoside (IPTG) at a final concentration of 1 mM. Induction was allowed to proceed overnight at 25 °C with shaking. Cells were pelleted via centrifugation (15,970 × g, 30 min, 4 °C) and resuspended in 20 mL IMAC buffer A (50 mM NaPO42–/50 mM NaCl, pH 8.0). The resuspended pellet was sonicated, centrifuged, and the supernatant was filtered through PVDF filter prior to loading onto a HiTrap IMAC FF 5 mL nickel affinity column using an Äkta pure protein purification system (GE Life Sciences). The protein was eluted using a gradient from 0% to 100% IMAC buffer B (50 mM NaPO42–/50 mM NaCl/400 mM Imidazole, pH 8.0) over 30 min. The collected protein was dialysed overnight against IEX buffer A (25 mM Tris, pH 8.0).
Recombinant (His)6-dual-FLAG-tagged Cys10Ala Val30Met TTR ((His)6-FT2-C10A-V30M TTR) was transfected and expressed using the same method mentioned in the previous paragraph; however, a different purification method was utilised. After centrifugation and harvesting, bacterial cells were resuspended in IMAC buffer C (500 mM NaCl/50 mM NaPO42–, pH 7.4). The resuspended pellet was sonicated, centrifuged, and the resulting supernatant was filtered through a PVDF filter prior to loading onto a HiTrap IMAC FF 5 mL nickel affinity column attached to an Äkta pure protein purification system (GE Life Sciences). Instead of using a linear gradient to elute the purified protein, a stepwise isocratic gradient was used at 0%, 20%, 25%, and 100% IMAC buffer D (500 mM NaCl/50 mM NaPO42–/700 mM Imidazole, pH 7.4) at a flow rate of 2 mL/min. (His)6-FT2-C10A-V30M TTR, which eluted at 100% IMAC buffer D, was collected and dialysed overnight in IEX buffer A (25 mM Tris, pH 8). Protein purity and identity was assessed through native gel electrophoresis, liquid chromatography/mass spectrometry (LC/MS), and ultra-performance liquid chromatography (UPLC) analysis.
HPLC quantification of tafamidis concentration in CSF
Tafamidis concentrations were quantified in three V30M/WT polyneuropathy patient CSF samples (D002, D004, D008) employing a high-performance liquid chromatography (HPLC) method previously described [Citation43]. A standard curve was generated by ex vivo addition of tafamidis (using a 50× stock solution in dimethyl sulfoxide (DMSO)) to pooled healthy donor CSF samples composed of WT TTR (37 °C, 20 h incubation). The final tafamidis concentrations employed for the standard curve were: 0, 25, 50, 100, 250, and 500 nM. Tafamidis extraction was then performed by mixing 40 µL of each CSF standard with 200 µL of acetonitrile containing 1% (w/v) trichloroacetic acid. Samples were shaken at 1500 rpm for 30 min (25 °C) and then centrifuged for 10 min at maximal speed in a tabletop centrifuge. Ten µL of the microcentrifuged supernatant was injected into a Waters Symmetry C18 column (3.5 µm, 4.6 × 75 mm). A chromatographic gradient of 5% to 95% solution B over 20 min (solution A: 95% water, 4.9% acetonitrile, 0.1% trifluoracetic acid; solution B: 4.9% water, 95% acetonitrile, 0.1% trifluoroacetic acid) was performed at a flow rate of 1 mL/min using an Agilent 1260 Infinity HPLC pump. Fluorescence detection (excitation: 310 nm, emission: 370 nm) was employed to detect tafamidis in both the standard curve and for patient samples. The tafamidis peak eluted at ∼15.5 min for the CSF standards and for the CSF derived from polyneuropathy patients treated orally with 20 mg Vyndaqel®.
Measurement of native TTR in CSF
Native TTR levels in CSF were quantified using fluorogenic small molecule A2 employing a Waters Acquity H-Class Bio-UPLC (ultra-performance liquid chromatography) instrument fitted with a Waters Protein-Pak Hi Res Q ion exchange column (strong anion exchange, 5 µM particle size, 4.6 × 100 mm column). A standard curve was prepared using recombinant WT TTR at concentrations of 0, 125, 250, 500, and 1000 nM in IEX buffer A (25 mM Tris, pH 8). The freshly prepared standard curve samples (90 µL) were incubated with 10 µL of fluorogenic small molecule A2 (final concentration = 500 µM) for 3 h at ≈ 25 °C [Citation50, Citation51]. After incubation, 90 µL of the standard sample was injected into the ion exchange column and separated using a linear 24% to 29% IEX B gradient (25 mM Tris/1 M NaCl, pH 8.0) over 10 min at a flow rate of 0.5 mL/min. The TTR-(A2)2 fluorescent conjugate peak (excitation: 328 nm, emission: 430 nm) was integrated and used to construct the standard curve. The WT TTR in pooled healthy CSF samples (90 µL) was also derivatized by incubation with 500 µM A2 for 3 h at ≈ 25 °C prior to injection of each sample into the anion exchange column and TTR concentration was quantified using the standard curve. All samples were prepared and measured in triplicate [Citation43].
CSF-TTR subunit exchange assay to assess kinetic stability
Subunit exchange rates were first measured for WT TTR in pooled CSF from healthy donors as previously described [Citation43], except that (His)6-FT2-C10A TTR was added to CSF in place of dual-FLAG tagged WT TTR (FT2-WT TTR). The (His)6-FT2-C10A TTR construct was used to prevent Cys10 air oxidation, which can occur on the time scale of subunit exchange, affording additional peaks. The (His)6 tag is not expected to affect subunit exchange kinetics, as it is separated from the TTR tetramer by the dual FLAG tag. A pipet was used to add 21.95 µL of a 39.17 µM recombinant (His)6-FT2-C10A TTR solution to 4.28 mL of CSF to afford a final (His)6-FT2-C10A concentration of 200 nM. Five hundred eighty-eight µL of the CSF/(His)6-FT2-C10A TTR mixture was then incubated with 12 µL of a 50× tafamidis stock solution to afford final tafamidis concentrations of 50, 125, 250, 500, 750, and 1000 nM (final DMSO concentration in all reactions = 2%). Samples were then incubated at 25 °C over a period of nine days and time points were collected at 0, 24, 72, 120, 168, and 216 h. For each timepoint, the reaction was stopped by the addition of fluorogenic small molecule A2 at a final concentration of 500 µM. Samples were allowed to incubate with small molecule A2 for at least 3 h to allow for complete covalent labelling of the Lys15 residues in two of the four TTR subunits within each native tetramer. The samples were then injected into a Waters Protein-Pak Hi-Res Q ion exchange column (5 µM, 4.6 × 100 mm) using a Waters UPLC pumping at a flow rate of 0.5 mL/min. The five peaks (see ) resulting from subunit exchange were separated using a linear gradient of 24% to 46% IEX buffer B over 35 min (IEX buffer A: 25 mM Tris, pH 8.0; IEX buffer B: 25 mM Tris/1 M NaCl, pH 8.0) employing fluorescence detection (excitation: 328 nm, emission: 430 nm). The rate of subunit exchange was calculated using the rate of appearance of peak 3 (corresponding to tetramers with a 2:2 flag-tagged:non-flag tagged subunit stoichiometry) in biological triplicate [Citation44].
Figure 1. Tafamidis is a WT TTR kinetic stabiliser in pooled human cerebrospinal fluid (CSF) from healthy donors. (A) Schematic of the steps involved in TTR tetramer dissociation and reassociation of protomers associated with subunit exchange between untagged endogenous WT TTR subunits (blue squares) and (His)6-FT2-C10A TTR reporter subunits (yellow squares) Note that only one of three possible subunit arrangements is shown for the 2:2 tetramer. (B) The kinetics of TTR subunit exchange in CSF over nine days between endogenous WT TTR (∼203 nM) and (His)6-FT2-C10A reporter TTR (200 nM) as a function of increasing tafamidis concentration. (C) Average kinetic rate constants obtained from fitting the data, revealing dose-dependent stabilisation of WT TTR in CSF with increasing tafamidis concentration (D) Ex vivo incubation of tafamidis in healthy pooled CSF shows that decreasing the [TTR tetramer]/[tafamidis] ratio increases stability of endogenous WT TTR in human CSF. The colours in all panels correspond to the key shown in panel B.
![Figure 1. Tafamidis is a WT TTR kinetic stabiliser in pooled human cerebrospinal fluid (CSF) from healthy donors. (A) Schematic of the steps involved in TTR tetramer dissociation and reassociation of protomers associated with subunit exchange between untagged endogenous WT TTR subunits (blue squares) and (His)6-FT2-C10A TTR reporter subunits (yellow squares) Note that only one of three possible subunit arrangements is shown for the 2:2 tetramer. (B) The kinetics of TTR subunit exchange in CSF over nine days between endogenous WT TTR (∼203 nM) and (His)6-FT2-C10A reporter TTR (200 nM) as a function of increasing tafamidis concentration. (C) Average kinetic rate constants obtained from fitting the data, revealing dose-dependent stabilisation of WT TTR in CSF with increasing tafamidis concentration (D) Ex vivo incubation of tafamidis in healthy pooled CSF shows that decreasing the [TTR tetramer]/[tafamidis] ratio increases stability of endogenous WT TTR in human CSF. The colours in all panels correspond to the key shown in panel B.](/cms/asset/4ce21cda-d114-4a75-bbf5-8c57c217b0f5/iamy_a_2167595_f0001_c.jpg)
Transthyretin subunit exchange rates were also measured in four heterozygotic V30M/WT polyneuropathy patient CSF samples. Three of the four patients from whom CSF was obtained were being treated orally with 20 mg Vyndaqel® QD (D002, D004, and D008). Tafamidis-CSF concentrations were first reassessed using the analytical HPLC tafamidis quantification method described in the previous section. After quantifying the tafamidis-CSF concentration in each sample, additional tafamidis was added ex vivo to increase the concentration to 500 nM. To initiate subunit exchange, recombinant (His)6-FT2-C10A-V30M was added to each CSF sample to afford a final reporter TTR concentration of 200 nM. Subunit exchange samples were collected at 0, 72, 144, and 216 h timepoints. At each timepoint, 1 µL of a 5 mM small molecule A2 solution was added to 99 µL of the CSF/(His)6-FT2-C10A-V30M reaction mixture, affording a final A2 concentration of 50 µM. Although this concentration is ten times less than what was used for the subunit exchange with pooled healthy CSF [Citation43], it provides sufficient excess to completely label TTR in CSF, as assessed by control experiments, in part because TTR concentration in the CSF is lower than it is in the plasma [Citation52]. The conjugation reaction was allowed to incubate for at least 3 h at 25 °C prior to injection into the UPLC. The five peaks were separated using the same linear gradient described in the previous paragraph. The rate of subunit exchange was once again calculated using peak 3. Due to the limited quantity of CSF, we were only able to complete one kinetic time course for each V30M/WT TTR CSF sample.
Tafamidis was also added to CSF sample D006, from the V30M/WT polyneuropathy patient who was not taking tafamidis orally, to measure the rate of subunit exchange. To initiate subunit exchange, recombinant (His)6-FT2-C10A-V30M was added to each CSF sample to afford a final reporter TTR concentration of 200 nM. Tafamidis was then added ex vivo to the CSF from patient D006 at a final concentration of 500 nM. The remainder of the analysis proceeded exactly as described in the previous paragraph.
Nonlinear fits to TTR binding data obtained from subunit exchange measurements
A model for the kinetics of TTR subunit exchange in the presence of tafamidis (which binds to TTR and inhibits subunit exchange) and albumin (which competes for tafamidis) has been reported previously [Citation44]. We fit this model to the subunit exchange data for WT TTR in CSF using an identical procedure to that reported previously by Nelson et al. [Citation44]. We assumed values of Kd1 = 3.1 nM and Kd2 = 238 nM for the first and second dissociation constants of tafamidis binding to TTR. The fits yielded estimates for the dissociation rate constant of TTR in CSF (kdiss) and the dissociation constant for binding of tafamidis to albumin (Kd,Alb).
Study approval
This study was approved before patient enrolment by the relevant institutional review boards at the Centro Hospitalar do Porto and Scripps Research. All participants provided written informed consent.
Results
Tetramer dissociation is rate-limiting for subunit exchange [Citation49,Citation51]. In order to assess the concentration dependence of tafamidis-mediated kinetic stabilisation of TTR in CSF, the subunit exchange method that was previously used to quantify TTR kinetic stability in human plasma [Citation44,Citation51] was adapted for use in human CSF [Citation43]. The concentration of native tetrameric WT TTR present in pooled human CSF from healthy donors was first measured in order to determine the concentration of tagged TTR to be added to CSF in order to detect the process of subunit exchange. Tetrameric WT TTR was quantified in the CSF through its selective reaction with the fluorogenic small molecule A2, which affords a fluorescent tetrameric TTR conjugate that can be quantified chromatographically, as previously described [Citation43,Citation50,Citation51]. The concentration of V30M and/or WT TTR tetramers (hereafter referred to as the V30M/WT TTR tetramer) present in CSF of heterozygous polyneuropathy patients was also quantified by A2 conjugate formation [Citation43]. In each case the TTR-(A2 substructure)2 covalent conjugate is isolated from the ≈2610 proteins in human CSF [Citation53] by ion exchange chromatography and the TTR-(A2 substructure)2 conjugate is quantified by fluorescent detection. We generated a standard curve by reacting A2 with different concentrations of recombinant WT TTR. Three different lot numbers of purchased CSF from healthy humans were combined and three technical replicates yielded an average native WT TTR CSF concentration of 203 ± 22 nM. This value was in accordance with previously published WT TTR CSF concentrations quantified by antibody-based assays [Citation52] and the V30M/WT TTR tetramer values that we previously published (≈ 271.5 nM) [Citation43].
We next utilised subunit exchange to quantify the concentration dependence of tafamidis-mediated WT TTR kinetic stabilisation in the CSF [Citation43,Citation44]. Using the pooled healthy human CSF samples, recombinant (His)6-FT2-C10A TTR was added at a final concentration of 200 nM to be able to measure the exchange of WT TTR subunits between the untagged endogenous tetramers and tagged exogenously added tetramers. The Cys10Ala mutation in the reporter tetramer prevents oxidative modifications of the cysteine residue that can occur on the timescale of subunit exchange, which can complicate chromatographic analysis. Immediately after initiating subunit exchange, tafamidis was added at increasing final concentrations ranging from 0 to 1000 nM (final DMSO concentration in each case = 2%).
The decrease in the rate of WT TTR subunit exchange as a function of increasing tafamidis concentration in the CSF (50, 125, 250, 500, 750 and 1000 nM; the definition of kinetic stabilisation) was calculated by measuring the rate at which the (WT TTR)2((His)6-FT2-C10A TTR)2 tetramer appeared, which is peak 3 in the ion exchange chromatogram () [Citation44]. This subunit exchange process is well approximated as a monoexponential process [Citation49,Citation51,Citation54,Citation55]. The 125 nM tafamidis concentration was evaluated because it is the average tafamidis concentration achieved in the CSF after repeated once daily oral dosing of V30M/WT TTR polyneuropathy patients taking 20 mg of Vyndaqel® [Citation43]. This concentration reduced the rate of WT TTR subunit exchange in CSF by 35% before correction for added (His)6-FT2-C10A TTR (; ). We show below using methodology described previously [Citation44] that this corresponds to a decrease in the effective WT TTR dissociation rate of 42% after correcting for competition for tafamidis binding by the flag-tagged TTR added to measure subunit exchange (see below). We assumed that quadrupling the oral Vyndaqel® (tafamidis) daily dose from 20 mg to 80 mg would also increase the tafamidis CSF concentration by a factor of four to 500 nM, assuming a linear relationship [Citation43]. The oral 61 mg Vyndamax® dose is a different formulation that affords the same plasma concentration as the 80 mg Vyndaqel® dose [Citation42]. Thus, we also tested a tafamidis concentration of 500 nM and found a 77% decrease in the subunit exchange rate before correction, corresponding to an 87% reduction in the effective WT TTR tetramer dissociation rate, after correcting for the added (His)6-FT2-C10A TTR [Citation44].
Table 1. Average kinetic rate constants of TTR subunit exchange in CSF.
We next assessed the tafamidis-mediated kinetic stabilisation of V30M/WT TTR tetramer individually in the CSF from four symptomatic Val30Met/WT polyneuropathy patients using a tafamidis concentration of 500 nM. Unfortunately, we did not have enough CSF to do a full dose response curve as we did for WT TTR healthy control subjects. However, at an average CSF tafamidis concentration of 125 nM (derived from 20 mg daily Vyndaqel® treatment), the kinetic stability of V30M/WT TTR tetramer in the CSF of polyneuropathy patients was previously reported to be an approximately 50% reduction in the rate of tetramer dissociation (kex = 0.002 h−1 versus different untreated patients kex = 0.004 h−1) [Citation43]. Tafamidis-CSF concentrations were measured in three of the patients who were undergoing chronic treatment with 20 mg Vyndaqel® QD at the time of lumbar puncture (D002, D004, D008) [Citation38]. In this study, additional tafamidis was added ex vivo to increase the final tafamidis-CSF concentration of each sample to 500 nM. (His)6-FT2-C10A-V30M TTR was then added to each sample at a final concentration of 200 nM to monitor subunit exchange. The subunit exchange reaction was allowed to proceed for nine days, and the rate of subunit exchange was quantified by the appearance of peak 3 in the chromatogram (i.e. tetramers with the composition (V30M and/or WT TTR)2((His)6-FT2-C10A-V30M TTR)2 [Citation44]). Notably, a 500 nM tafamidis-CSF concentration added to the V30M/WT tetramer reduced the subunit exchange rate constant to be 6.5-fold slower (kex, average V30M/WT = 0.0005 h−1; four coloured traces ) than WT-TTR subunit exchange in the absence of tafamidis (kex, average WT DMSO vehicle control = 0.0034 h−1; black trace in ). uses bar graphs to depict the subunit exchange rate constants of the V30M/WT tetramer CSF samples with 500 nM tafamidis added (coloured bars) versus WT TTR in CSF without tafamidis added (black bar). Within our small cohort (n = 4), the 500 nM tafamidis CSF concentration slows the tetramer dissociation rate in V30M/WT heterozygotes by ≈ 50%, which is similar to the tafamidis-associated slowing of WT TTR tetramer dissociation in human CSF (). There was no statistically significant difference in the rate of tetramer dissociation between WT TTR and the V30M/WT tetramer at a tafamidis concentration of 500 nM (comparison using an unpaired t-test).
Figure 2. Tafamidis is an effective kinetic stabiliser of the TTR tetramers comprising human CSF from four heterozygous V30M/WT TTR familial amyloid polyneuropathy (FAP) patients. (A) Four subunit exchange time courses between endogenous V30M/WT TTR and (His)6-FT2-C10A-V30M TTR reporter added to human CSF derived from the four heterozygous V30M/WT CSF patients whose samples are identified as D002, D004, D006 and D008 (coloured traces; ≈ 266 nM tetrameric TTR) in the presence of 500 nM tafamidis. The black time course at the top depicts the subunit exchange kinetics of WT TTR (without added tafamidis), whereas the blue time course partially obscured by the coloured traces shows the subunit exchange kinetics of WT TTR with 500 nM tafamidis added. Both of these subunit exchange-based TTR kinetic stability measurements were performed using (His)6-FT2-C10A TTR as the reporter. (B) Calculated subunit exchange rate constants (C) Comparison of the subunit exchange rates extracted from the time courses shows no significant difference between the TTR kinetic stability afforded by 500 nM tafamidis in CSF comprising WT TTR and 500 nM tafamidis in CSF harbouring V30M/WT tetramers from polyneuropathy patient CSF. The colours in all panels correspond to the key shown in panel A.

The rates of V30M/WT TTR tetramer dissociation were previously reported in CSF after long-term oral dosing of polyneuropathy patients with 20 mg Vyndaqel® QD, where the average tafamidis-CSF concentration achieved in these patients was 125 nM [Citation43]. The Vyndaqel®-treated (20 mg QD) V30M/WT TTR polyneuropathy patients exhibited a statistically significant 1.7-fold slowing in tetramer dissociation rate when compared to healthy control CSF harbouring WT TTR. In addition, the Vyndaqel®-treated (20 mg QD) V30M/WT TTR polyneuropathy patients exhibited a slowed tetramer dissociation rate trend versus V30M/WT TTR polyneuropathy patients not treated with tafamidis; however, the difference was not statistically significant within this small cohort [Citation43].
In blood plasma, the stabilisation of TTR by tafamidis binding is strongly impacted by competition for tafamidis by albumin. To understand this effect in CSF, we fitted the TTR stabilisation versus tafamidis concentration subunit exchange data in healthy donor CSF to a model for TTR subunit exchange in the presence of albumin. Subunit exchange is well approximated as a monoexponential process with an apparent rate constant kex = funbound kdiss, where funbound is the fraction of TTR with no ligands bound and kdiss is the intrinsic TTR tetramer dissociation rate constant. The parameter funbound is a function of the affinity of tafamidis for TTR and albumin, as discussed in the Supplemental Methods section of Nelson et al. [Citation44]. We assumed that the binding constants of tafamidis for TTR’s two binding sites are the same in CSF as they are in plasma (Kd1 = 3.1 nM, Kd2 = 238 nM), and globally fit the data by varying kdiss and Kd,alb to optimise the fit as described previously [Citation44] using a concentration of 3.7 μM for albumin in CSF [Citation52]. The resulting best-fit value of kdiss was 0.0041 ± 0.0006 h−1, roughly a 3.6-fold reduction in the dissociation rate for WT TTR in CSF compared to plasma (in which kdiss = 0.0149 h−1). This slower subunit exchange in CSF is consistent with previous observations [Citation43]. The best fit value of Kd,alb in CSF was 220
30 nM, compared to 1.8
0.1 µM reported in human plasma, indicating tighter tafamidis binding to albumin in CSF compared to plasma. One plausible, if not likely, explanation for this result is that plasma has a much higher concentration of non-tafamidis metabolites than CSF, which could compete with tafamidis for albumin binding, effectively increasing Kd,Alb in plasma. Despite the apparently tighter binding of tafamidis to albumin in CSF, tafamidis is still highly effective for stabilising TTR in CSF because the concentration of albumin in CSF is over 200-fold lower than in plasma [Citation52]. Thus, the expected funbound for TTR in CSF at 125 nM and 500 nM tafamidis would be 0.58 and 0.13, respectively, after correcting for the added FLAG-tagged TTR that is present during subunit exchange experiments and assuming a TTR concentration of 200 nM. For comparison, these values of funbound would be achieved at 2.1 and 9.7 μM of tafamidis in plasma, well within the range of tafamidis plasma concentrations that have been found to be clinically effective [Citation44].
It was not possible to fit the subunit exchange data from the heterozygous Val30Met polyneuropathy patients in the way described above for CSF harbouring WT TTR because of the dearth of data. Three of four patients were being treated with tafamidis, so only one of these samples was tafamidis-free, and in any case there was only enough CSF to collect time dependent data at one concentration of tafamidis. We elected to perform subunit exchange at a tafamidis concentration of 500 nM to enable comparison with the subunit exchange experiments of the healthy controls. Thus, it was impossible to estimate kdiss in the absence of tafamidis. However, subunit exchange is expected to be slowed in the presence of 125 nM tafamidis and is observed to be strongly slowed with 500 nM tafamidis, relative to previous measurements for Val30Met TTR heterozygotes [Citation43], and it is therefore reasonable to expect a CNS clinical benefit from tafamidis treatment [Citation48].
Discussion
Transthyretin amyloidosis patients are now living longer thanks to the availability of regulatory agency approved drugs (tafamidis, patisiran, inotersen) that slow the progression of peripheral TTR aggregation-associated pathologies [Citation3–5,Citation39,Citation40]. For those ATTRv therapies lacking pharmacologically meaningful CNS exposure, the risk of developing CNS manifestations is expected to increase substantially after about a decade of treatment [Citation13,Citation16,Citation18,Citation19]. The 200 nM TTR tetramer concentration measured in the CSF of WT TTR controls or the
270 nM TTR tetramer concentration in V30M/WT polyneuropathy patients [Citation43] is much lower than the TTR concentration in blood (
3.4–5 µM), which likely explains the slower development of TTR pathology in the CNS, as aggregation is concentration-dependent [Citation43,Citation46]. While the CNS pathology is poorly understood in the >100,000 WT cardiomyopathy patients present worldwide, the risk of cerebrovascular bleeds in these patients has been noted, and could become more problematic as these patients live longer [Citation23], especially in the subset of cardiomyopathy patients treated with disease modifying therapies that do not enter the brain [Citation56–58]. By using ATTR drugs with pharmacologically useful CNS penetration, we have an opportunity to slow, if not prevent, the onset of CNS ATTR pathology to avoid transforming a peripheral amyloid disease into a CNS amyloid disease.
Generally speaking, antisense oligonucleotides and siRNA for CNS targets are administered by intrathecal injection since they don’t enter the CNS at pharmacologically relevant concentrations when administered subcutaneously or intravenously [Citation59,Citation60]. Thus, it is unlikely that the TTR mRNA-lowering drugs patisiran and inotersen will penetrate the CNS and ameliorate CNS pathology [Citation39,Citation40], although this merits experimental scrutiny. Small molecule drugs can enter the brain through the blood-brain barrier (BBB) membrane if their MW is low, the degree of branching is minimal, the number of rotatable bonds is low, the number of hydrogen bond acceptors is few, the aromatic density is high, and the log P (octanol water partition constant) is around 2 [Citation61]. Tafamidis meets most of these criteria, but its structure includes a carboxylate, which opposes BBB permeability. Nonetheless, tafamidis enters the CSF, albeit modestly, likely by passive transport through the blood-brain barrier, the membrane that surrounds the capillaries of the circulatory system in the brain. Tafamidis probably does not gain brain access by active transport [Citation43]. The 20 mg Vyndaqel® daily dose affords an average CSF tafamidis concentration of 125 nM in heterozygote V30M polyneuropathy patients. The half-life of tafamidis in the CSF is unknown, but the half-life exceeds 24 h in blood. The Parkinson’s drug tolcapone (200 mg, oral administration), which is being considered for repurposing as a ATTR therapy [Citation62], affords a mean CSF concentration of 56.4 nM 1–4 h after oral dosing in Parkinsonian patients (n = 12). Based on our previous results in blood plasma, we expect that this concentration of tolcapone would result in about half of the reduction in the TTR tetramer dissociation rate that the 20 mg of dose of Vyndaqel® achieves in the CSF [Citation63]. It may be challenging to increase the typical 200 mg oral dose of tolcapone, given its black box warning.
Currently, it is unclear what level of TTR kinetic stabilisation is needed to achieve a substantial clinical benefit in the CNS for ATTR. In the case of V30M/WT polyneuropathy patient CSF, there is about a 50% reduction in tetramer dissociation rate in the CSF imposed on the V30M/WT TTR tetramers by the 125 nM tafamidis CSF concentration, but rigorously quantifying the extent of kinetic stabilisation corrected for the added tagged TTR tetramer will require more data than is currently available (n = 9) [Citation43]. The 125 nM tafamidis CSF concentration achieved by 20 mg Vyndaqel® daily dosing seems to at least delay the onset of CNS symptoms in V30M/WT polyneuropathy patients being followed for more than a decade at the Unidade Corino de Andrade, in the Neurology Department of the Hospital de Santo Antonio, Porto, Portugal. Increasing the Vyndaqel® dose from 20 mg to 80 mg (bioequivalent to the 61 mg Vyndamax® dose) is envisioned to increase the tafamidis CSF concentration by about a factor of four to 500 nM, leading to an approximate 50–70% reduction in the V30M/WT tetramers dissociation rate (≈ 77% reduction in WT TTR tetramer dissociation rate is achieved by a 125 nM tafamidis CSF concentration; ). Currently, the majority of hereditary polyneuropathy patients are taking the 20 mg Vyndaqel® daily dose; however, the 61 mg Vyndamax® dose of tafamidis would likely be more protective against the development of CNS pathology. Testing this hypothesis merits a clinical trial, given the excellent safety profile of tafamidis [Citation4]. These hypotheses can also be scrutinised by following CNS pathology by comparing tafamidis treatment (low and high doses) to patisiran or inotersen treatment in the commercial setting in both polyneuropathy and cardiomyopathy patients.
Regarding the extent of TTR kinetic stabilisation that is needed to ameliorate peripheral pathology in ATTR, collectively our data to date suggests that a 40% or greater slowing in the tetramer dissociation rate in human plasma leads to a clinical response [Citation46,Citation48]. Seventy percent of V30M polyneuropathy patients respond to 20 mg Vyndaqel® QD (progression of neurodegeneration is slowed), which yields an average tafamidis plasma concentration of ≈ 9 µM [Citation46], corresponding to a slowing of the TTR tetramer dissocation rate by ≈ 40% (correcting for the tagged TTR added to plasma to perform subunit exchange) [Citation48]. Given that 20 mg Vyndaqel® daily dosing ameliorates WT and hereditary TTR cardiomyopathy [Citation4] and affords a ≈ 9 µM plasma concentration in WT TTR cardiomyopathy patients, the WT TTR tetramer dissociation rate in plasma would be expected to be slowed by ≈ 86% based on published data [Citation44]. The 80 mg Vyndaqel® daily dosing regimen yields a mean peak plasma concentration of ≈ 28 µM which lowers the rate of WT TTR tetramer dissocation by 97% [Citation44]. Biomarker data suggest that the 80 mg Vyndaqel® daily dose may ameliorate WT and hereditary TTR cardiomyopathy more than the 20 mg dose, although this data has limitations [Citation47]. Thus, we expect that providing patients with 80 mg Vyndaqel® QD will enable greater TTR stabilisation in the CSF, resulting in further protection against CNS pathology.
The inheritance of highly destabilising TTR mutations causes heterozygous patients to initially present with CNS symptoms in a disease termed Hereditary (Familial) TTR Leptomeningeal Amyloidosis [Citation7,Citation25,Citation28]. This CNS tropism (there is little peripheral ATTRv pathology) results from the liver cells being able to direct highly destabilised TTR to endoplasmic reticulum-associated degradation instead of secretion into the blood, thus minimising peripheral TTR aggregation. In contrast, the choroid plexus secretes these highly destabilised heterotetrameric TTR tetramers into the CSF at WT-like levels. This may be due to thyroxine (T4) pharmacological chaperoning within the endoplasmic reticulum [Citation26], i.e. T4 binds to and stabilises the TTR heterotetramers in the choroid plexus, preventing misfolded TTR from being degraded by endoplasmic reticulum-associated degradation prior to secretion. However, this T4 kinetic stabilisation may be temporary, as T4 is likely to dissociate in the CSF and allow tetramer dissociation, monomer misfolding and aggregation there. The average TTR tetramer concentration in the CSF of patients treated with the 20 mg Vyndaqel dose of tafamidis (266 nM, n = 5) is similar to that in the CSF of untreated V30M/WT TTR patients (274 nM, n = 4), indicating that tafamidis does not pharmacologically chaperone V30M/WT TTR in the CNS at this dose. This result is strikingly different from the significant increase in TTR concentration found in the blood plasma of V30M/WT TTR patients treated with the 20 mg Vyndaqel dose of tafamidis, which indicates substantial pharmacological chaperoning in the periphery [Citation48]. This result suggests that destabilised TTR variants are already being chaperoned from the choroid plexus as much as is possible by T4. Thus, tafamidis in the CSF will probably not result in higher levels of secretion of the highly aggregation prone variant tetramers associated with familial leptomeningeal TTR amyloidosis, which is desirable because increased TTR concentrations would increase the risk of aggregation. If the tafamidis concentration achievable in the CSF can be shown to slow the dissociation rate of a given TTR heterotetramer by greater than about 40% (which could be measured in CSF by ex vivo subunit exchange experiments analogous to those conducted herein), and if the lack of tafamidis pharmacological chaperoning (or modest pharmacological chaperoning) can be verified in the CNS by a clinical study, then tafamidis could be useful for treating Familial TTR Leptomeningeal Amyloidosis. It could be argued CNS penetrance of tafamidis is even more important in hereditary TTR Leptomeningeal Amyloidosis since the CNS phenotypes are acute. Thus, the response of the CNS symptoms of these patients to treatment with tafamidis might be measurable on a similar timescale to the responses observed with tafamidis treatment of the peripheral symptoms of TTR amyloidosis (18–30 months). However, some highly destabilised TTR variants may not bind tafamidis very well, hence the importance of enrolling patient genotypes that are likely to respond to the high dose formulations of tafamidis and performing ex vivo subunit exchange experiments for each of those genotypes. We still do not know whether slowing of the TTR heterotetramer dissociation rate by less than 40% would be expected to exhibit clinical benefit, but this could be evaluated by enrolling a subgroup exhibiting less slowing of the TTR heterotetramer dissociation rate based on ex vivo subunit exchange studies in CSF.
One of the limitations of this study relates to the small number of CSF samples studied. Another limitation of our study is the mathematical modeling, the accuracy of which is limited by the inherent noise in the data. Thus, the fraction tetramer occupied by tafamidis and the associated tetramer dissociation slowing may have significant errors if one of the numbers employed has to be revised based on new data. While we have biophysical evidence that WT TTR tetramers are kinetically more stable than V30M/WT heterotetramers in a buffer system, WT TTR tetramer stability and V30M/WT tetramer stability seems to be closer than what would be expected in CSF, which we do not yet understand.
What is unarguable from our TTR subunit exchange data in CSF is that the extent of slowing of TTR tetramer dissociation achieved as a function of increasing tafamidis concentration in the CSF is substantial, cf. . Our data suggest that the 80 mg Vyndaqel® daily oral dosing regimen or the 61 mg Vyndamax® daily oral dosing regimen will afford ≈ a 500 nM CSF concentration. We hypothesise that this tafamidis concentration would be near maximally protective against TTR aggregation-associated CNS pathology for the majority of TTR genotypes, but this can only be assessed quantitatively by a clinical study or trial.
Abbreviations | ||
ATTRv | = | variant transthyretin amyloid protein |
ATTRwt | = | wild type TTR amyloid protein |
CNS | = | central nervous system |
CSF | = | cerebrospinal fluid |
FAP | = | familial amyloid polyneuropathy |
QD | = | once-daily dose |
TTR | = | transthyretin |
UPLC | = | ultra-performance liquid chromatography |
V | = | variant |
WT | = | wild type |
Acknowledgments
We would like to acknowledge the team of neurologists, cardiologists, nephrologists and ophthalmologists who have carefully observed and followed the patients at the Unidade Corino de Andrade. In particular, we thank the physicians and nurses at Unidade Corino de Andrade who collected the CSF samples. Finally, we thank all the patients and their families for their willingness to participate and support this study.
Disclosure statement
J.W.K. and E.T.P. discovered tafamdis and receive sales royalties from Pfizer. J.W.K. was a paid consultant for and has received support for travel and accommodations from Pfizer, which sells tafamidis. T. C. has participated as an investigator in clinical trials for FoldRx, Pfizer, Alnylam, Ionis, and Prothena that were paid per protocol to Centro Hospitilar do Porto. T. C. is a consultant for Pfizer, Alnylam, Ionis, and Prothena. T. C. has been supported for travel, accommodation, and registration for scientific meetings by Pfizer, Alnylam, Ionis and Biogen.
Additional information
Funding
References
- Kelly JW. Does protein aggregation drive postmitotic tissue degeneration? Science Trans Med. 2021;13:eaax0914.
- Hammarström P, Schneider F, Kelly JW. Trans-suppression of misfolding in an amyloid disease. Science. 2001;293(5539):2459–2462.
- Coelho T, Maia LF, Martins da Silva A, et al. Tafamidis for transthyretin familial amyloid polyneuropathy a randomized, controlled trial. Neurology. 2012;79(8):785–792.
- Maurer MS, Schwartz JH, Gundapaneni B, et al. Tafamidis treatment for patients with transthyretin amyloid cardiomyopathy. N Engl J Med. 2018;379(11):1007–1016.
- Berk JL, Suhr OB, Obici L, et al. Repurposing diflunisal for familial amyloid polyneuropathy a randomized clinical trial. JAMA. 2013;310(24):2658–2667.
- Andrade C. A peculiar form of peripheral neuropathy; familiar atypical generalized amyloidosis with special involvement of the peripheral nerves. Brain. 1952;75(3):408–427.
- Uitti R, Donat J, Rozdilsky B, et al. Familial oculoleptomeningeal amyloidosis. Report of a new family with unusual features. Arch Neurol. 1988;45(10):1118–1122.
- Coelho T, Ines M, Conceicao I, et al. Natural history and survival in stage 1 Val30Met transthyretin familial amyloid polyneuropathy. Neurology. 2018;91(21):E1999–E2009.
- Sekijima Y, Ueda M, Koike H, et al. Diagnosis and management of transthyretin familial amyloid polyneuropathy in Japan: red-flag symptom clusters and treatment algorithm (vol 13, pg 6, 2018) [correction]. Orphanet J Rare Dis. 2019;14(1):1.
- Ikeda S. Amyloid neuropathy and autonomic dysfunction. Neurol Clin Neurosc. 2022;10(3):137–146.
- Ruberg FL, Grogan M, Hanna M, et al. Transthyretin amyloid cardiomyopathy JACC state-of-the-Art review. J Am Coll Cardiol. 2019;73(22):2872–2891.
- Kleefeld F, Scherret E, Knebel F, et al. Same same, but different? The neurological presentation of wildtype transthyretin (ATTRwt) amyloidosis. Amyloid. 2022;29(2):92–101.
- Ushiyama M, Ikeda S, Yanagisawa N. Transthyretin-type cerebral amyloid angiopathy in type-i familial amyloid polyneuropathy. Acta Neuropathol. 1991;81(5):524–528.
- Mitsuhashi S, Yazaki M, Tokuda T, et al. MRI analysis on a patient with the V30M mutation is characteristic of leptomeningeal amyloid. Amyloid. 2004;11(4):265–267.
- Yoshinaga T, Takei YI, Katayanagi K, et al. Postmortem findings in a familial amyloid polyneuropathy patient with homozygosity of the mutant Val30Met transthyretin gene. Amyloid. 2004;11(1):56–60.
- Maia LF, Magalhaes R, Freitas J, et al. CNS involvement in V30M transthyretin amyloidosis: clinical, neuropathological and biochemical findings. J Neurol Neurosurg Psychiatry. 2015;86(2):159–167.
- Salvi F, Pastorelli F, Plasmati R, et al. Brain microbleeds 12 years after orthotopic liver transplantation in Val30Met amyloidosis. JStroke Cerebrovasc Dis. 2015;24(6):E149–E151.
- Sekijima Y, Yazaki M, Oguchi K, et al. Cerebral amyloid angiopathy in posttransplant patients with hereditary ATTR amyloidosis. Neurology. 2016;87(8):773–781.
- da Silva AM, Cavaco S, Fernandes J, et al. Age-dependent cognitive dysfunction in untreated hereditary transthyretin amyloidosis. J Neurol. 2018;265(2):299–307.
- Sousa L, Coelho T, Taipa R. CNS involvement in hereditary transthyretin amyloidosis. Neurology. 2021;97(24):1111–1119.
- Westermark P, Sletten K, Johansson B, et al. Fibril in senile systemic amyloidosis is derived from normal transthyretin. Proc Natl Acad Sci USA. 1990;87(7):2843–2845.
- Rosenblum H, Castano A, Alvarez J, et al. TTR (transthyretin) stabilizers are associated with improved survival in patients with TTR cardiac amyloidosis. Circulation Heart Fail. 2018;11(4):e004769.
- Hornstrup LS, Frikke-Schmidt R, Nordestgaard BG, et al. Genetic stabilization of transthyretin, cerebrovascular disease, and life expectancy. Arterioscler Thromb Vasc Biol. 2013;33(6):1441–1447.
- Hammarstrom P, Sekijima Y, White JT, et al. D18G transthyretin is monomeric, aggregation prone, and not detectable in plasma and cerebrospinal fluid: a prescription for Central nervous system amyloidosis? Biochemistry. 2003;42(22):6656–6663.
- Sekijima Y, Hammarstrom P, Matsumura M, et al. Energetic characteristics of the new transthyretin variant A25T may explain its atypical Central nervous system pathology. Lab Invest. 2003;83(3):409–417.
- Sekijima Y, Wiseman RL, Matteson J, et al. The biological and chemical basis for tissue-selective amyloid disease. Cell. 2005;121(1):73–85.
- Herbert J, Wilcox JN, Pham KT, et al. Transthyretin: a choroid plexus-specific transport protein in human brain. The 1986 S. Weir Mitchell award. Neurology. 1986;36(7):900–911.
- Petersen RB, Goren H, Cohen M, et al. Transthyretin amyloidosis: a new mutation associated with dementia. Ann Neurol. 1997;41(3):307–313.
- Qin Q, Wei CB, Piao YS, et al. Current review of leptomeningeal amyloidosis associated with transthyretin mutations. Neurologist. 2021;26(5):189–195.
- Kametani F, Ikeda S, Yanagisawa N, et al. Characterization of a transthyretin-related amyloid fibril protein from cerebral amyloid angiopathy in type-1 familial amyloid polyneuropathy. J Neurol Scie. 1992;108(2):178–183.
- Blake CC, Geisow MJ, Oatley SJ, et al. Structure of prealbumin: secondary, tertiary and quaternary interactions determined by Fourier refinement at 1.8 A. J Mol Biol. 1978;121(3):339–356.
- Monaco HL, Rizzi M, Coda A. Structure of a complex of two plasma proteins: transthyretin and retinol-binding protein. Science. 1995;268(5213):1039–1041.
- White JT, Kelly JW. Support for the multigenic hypothesis of amyloidosis: the binding stoichiometry of retinol-binding protein, vitamin A, and thyroid hormone influences transthyretin amyloidogenicity in vitro. Proc Natl Acad Sci USA. 2001;98(23):13019–13024.
- Arvanitis M, Koch CM, Chan GG, et al. Identification of transthyretin cardiac amyloidosis using serum retinol-binding protein 4 and a clinical prediction model. JAMA Cardiol. 2017;2(3):305–313.
- Eisele YS, Monteiro C, Fearns C, et al. Targeting protein aggregation for the treatment of degenerative diseases. Nat Rev Drug Discov. 2015;14(11):759–780.
- Holmgren G, Ericzon BG, Groth CG, et al. Clinical improvement and amyloid regression after liver transplantation in hereditary transthyretin amyloidosis. Lancet. 1993;341(8853):1113–1116.
- Ericzon BG, Wilczek HE, Larsson M, et al. Liver transplantation for hereditary transthyretin amyloidosis: after 20 years still the best therapeutic alternative? Transplantation. 2015;99(9):1847–1854.
- Holmgren G, Steen L, Ekstedt J, et al. Biochemical effect of liver transplantation in two swedish patients with familial amyloidotic polyneuropathy (FAP-Met30). Clin Genet. 1991;40(3):242–246.
- Benson MD, Waddington-Cruz M, Berk JL, et al. Inotersen treatment for patients with hereditary transthyretin amyloidosis. N Engl J Med. 2018;379(1):22–31.
- Adams D, Gonzalez-Duarte A, O'Riordan WD, et al. Patisiran, an RNAi therapeutic, for hereditary transthyretin amyloidosis. N Engl J Med. 2018;379(1):11–21.
- Wange N, Anan I, Ericzon BG, et al. Atrial fibrillation and Central nervous complications in liver transplanted hereditary transthyretin amyloidosis patients. Transplantation. 2018;102(2):e59–e66.
- Lockwood PA, Le VH, O’Gorman MT, et al. The bioequivalence of tafamidis 61-mg free acid capsules and tafamidis meglumine 4 x 20-mg capsules in healthy volunteers. Clin Pharmacol Drug Devel. 2020;9(7):849–854.
- Monteiro C, da Silva AM, Ferreira N, et al. Cerebrospinal fluid and vitreous body exposure to orally administered tafamidis in hereditary ATTRV30M (p.TTRV50M) amyloidosis patients. Amyloid. 2018;25(2):120–128.
- Nelson LT, Paxman RJ, Xu J, et al. Blinded potency comparison of transthyretin kinetic stabilisers by subunit exchange in human plasma. Amyloid. 2021;28(1):24–29.
- Bulawa CE, Connelly S, Devit M, et al. Tafamidis, a potent and selective transthyretin kinetic stabilizer that inhibits the amyloid Cascade. Proc Natl Acad Sci USA. 2012;109(24):9629–9634.
- Monteiro C, Mesgazardeh JS, Anselmo J, et al. Predictive model of response to tafamidis in hereditary ATTR polyneuropathy. JCI Insight. 2019;4(12):e126526.
- Elliott P, Drachman BM, Gottlieb SS, et al. Long-term survival with tafamidis in patients with transthyretin amyloid cardiomyopathy. Circulation Heart Fail. 2022;15(1):120.008193.
- Monteiro C, Mesgarzadeh JS, Anselmo J, et al. Tafamidis polyneuropathy amelioration requires modest increases in transthyretin stability even though increases in plasma native TTR and decreases in non-native TTR do not predict response. Amyloid. 2022;2022:1–15.
- Wiseman RL, Green NS, Kelly JW. Kinetic stabilization of an oligomeric protein under physiological conditions demonstrated by a lack of subunit exchange: implications for transthyretin amyloidosis. Biochemistry. 2005;44(25):9265–9274.
- Choi S, Ong DS, Kelly JW. A stilbene that binds selectively to transthyretin in cells and remains dark until it undergoes a chemoselective reaction to create a bright blue fluorescent conjugate. J Am Chem Soc. 2010;132(45):16043–16051.
- Rappley I, Monteiro C, Novais M, et al. Quantification of transthyretin kinetic stability in human plasma using subunit exchange. Biochemistry. 2014;53(12):1993–2006.
- Reiber H. Dynamics of brain-derived proteins in cerebrospinal fluid. Clin Chim Acta. 2001;310(2):173–186.
- Begcevic I, Brinc D, Drabovich AP, et al. Identification of brain-enriched proteins in the cerebrospinal fluid proteome by LC-MS/MS profiling and mining of the human protein atlas. Clin Proteom. 2016;13(1):13.
- Schneider F, Hammarstrom P, Kelly JW. Transthyretin slowly exchanges subunits under physiological conditions: a convenient chromatographic method to study subunit exchange in oligomeric proteins. Protein Sci. 2001;10(8):1606–1613.
- Nelson GA, Edward DP, Wilensky JT. Ocular amyloidosis and secondary glaucoma. Ophthalmology. 1999;106(7):1363–1366.
- Jacobson DR, Pastore RD, Yaghoubian R, et al. Variant-sequence transthyretin (isoleucine 122) in late-onset cardiac amyloidosis in black Americans. N Engl J Med. 1997;336(7):466–473.
- Tanskanen M, Peuralinna T, Polvikoski T, et al. Senile systemic amyloidosis affects 25% of the very aged and associates with genetic variation in alpha2-macroglobulin and tau: a population-based autopsy study. Annals Med. 2008;40(3):232–239.
- Ueda M, Horibata Y, Shono M, et al. Clinicopathological features of senile systemic amyloidosis: an ante- and post-mortem study. Mod Pathol. 2011;24(12):1533–1544.
- Mazur C, Powers B, Zasadny K, et al. Brain pharmacology of intrathecal antisense oligonucleotides revealed through multimodal imaging. JCI Insight. 2019;4(20):129240.
- Brazil R. Silencing the genes that harm the brain. The Pharmaceutical Journal. 2022;309(7964). DOI:10.1211/PJ.2022.1.151588
- Silverman RB, Holladay MW. The organic chemistry of drug design and drug action. 3rd ed. Amsterdam: Elsevier; 2014. March 29, 2014.
- Sant’Anna R, Gallego P, Robinson LZ, et al. Repositioning tolcapone as a potent inhibitor of transthyretin amyloidogenesis and associated cellular toxicity. Nat Commun. 2016;7:10787.
- Russ H, Müller T, Woitalla D, et al. Detection of tolcapone in the cerebrospinal fluid of parkinsonian subjects. Naunyn-Schmiedeberg’s Arch Pharmacol. 1999;360(6):719–720. 1999/12/01