Abstract
We have evaluated the potential of plasma albumin to provide a sensitive biomarker of exposure to commonly used organophosphorus pesticides in order to complement the widely used measure of acetylcholinesterase (AChE) inhibition. Rat or human plasma albumin binding by tritiated-diisopropylfluorophosphate (3H-DFP) was quantified by retention of albumin on glass microfibre filters. Preincubation with unlabelled pesticide in vitro or dosing of F344 rats with pesticide in vivo resulted in a reduction in subsequent albumin radiolabelling with 3H-DFP, the decrease in which was used to quantify pesticide binding. At pesticide exposures producing approximately 30% inhibition of AChE, rat plasma albumin binding in vitro by azamethiphos (oxon), chlorfenvinphos (oxon), chlorpyrifos-oxon, diazinon-oxon and malaoxon was reduced from controls by 9±1%, 67±2%, 56±2%, 54±2% and 8±1%, respectively. After 1 h of incubation with 19 µM 3H-DFP alone, the level of binding to rat or human plasma albumins reached 0.011 or 0.039 moles of DFP per mole of albumin, respectively. This level of binding could be further increased by raising the concentration of 3H-DFP, increasing the 3H-DFP incubation time, or by substitution of commercial albumins for native albumin. Pesticide binding to albumin was presumed covalent since it survived 24 h dialysis. After dosing rats with pirimiphos-methyl (dimethoxy) or chlorfenvinphos (oxon) (diethoxy) pesticides, the resultant albumin binding were still significant 7 days after dosing. As in vitro, dosing of rats with malathion did not result in significant albumin binding in vivo. Our results suggest albumin may be a useful additional biomonitor for moderately low-level exposures to several widely used pesticides, and that this binding differs markedly between pesticides.
Introduction
Exposure to organophosphorus (OP) pesticides can be estimated indirectly by inhibition of erythrocyte acetyl- or plasma butyryl-cholinesterase, or by measurement of urinary metabolites. In addition, a number of immune assays have been developed that are sufficiently sensitive to measure OP pesticides directly (Zhang et al. Citation2007). However, none of these approaches are ideal. Most OP pesticides are rapidly metabolised and eliminated from the body post-exposure. Cholinesterase inhibition, although longer lasting, does not discriminate between different inhibitors. Similarly, OP pesticides with very different toxic potential can share the same urinary metabolites, and these are also relatively rapidly eliminated post-exposure. It can, however, be important to be able to discriminate between exposures to different specific OP pesticides, because their action on targets such as neuropathy target esterase (Lotti Citation2002, Lotti & Moretto Citation2005), acylpeptide hydrolase (Richards et al. Citation2000) or other serine hydrolases (Ray & Richards Citation2001, Casida & Quistad Citation2004) does not parallel their actions on acetylcholinesterase (AChE). This diversity of action limits the value of AChE inhibition when used as the sole index of exposure. In addition, there are other forms of ill-health that have been associated with low-level exposure to OPs for which a molecular target has not yet been identified (Pope Citation1999, Ray & Richards Citation2001, Lotti Citation2002, Abou-Donia Citation2003, Kamel et al. Citation2005, Costa Citation2006), and for which it cannot be assumed that there is a parallel structure–activity relationship to that seen for AChE inhibition. Hence there is value in developing other additional biomarkers that have the potential to identify specific OP exposures. For example, acylpeptide hydrolase has a similar sensitivity to diisopropylfluorophosphate (DFP) exposure in vitro as butyrylcholinesterase (BuChE), but a markedly lower rate of spontaneous reactivation in vivo, indicative of its potential as a monitor of previous DFP or OP exposures (Quistad et al. Citation2005). This diversity of biological targets has potential to be exploited by use of a spectrum of biomarkers to ‘fingerprint’ different OP exposures.
Another potential biomarker for discriminating OP pesticide exposures is serum albumin. Albumin is adducted by DFP, and has been shown to bind a large number of OP pesticides in vitro – at least when incubated with relatively high pesticide to albumin molar ratios well beyond those likely to be encountered in vivo (Peeples et al. Citation2005). However, binding of pesticides to albumin may not always be covalent, notably for phosphorothioates (Mourik & Jong Citation1978, Maliwal & Guthrie Citation1981, Sultatos et al. Citation1984). We have shown that albumin does display a sensitive and compound specific OP pesticide binding at relatively low-exposure levels producing no more than 30% inhibition of AChE activity (Carter et al. Citation2007) – a level commonly considered to represent a just sub-symptomatic exposure for pesticide operators (Gallo & Lawryk Citation1991). An additional aspect of the binding of albumin by OPs is that albumin could act as a significant decoy target, reducing toxicity by limiting the access of OPs to functional targets in brain and muscle (Qiao et al. Citation2001).
Human serum albumin is the major blood protein, constituting 47% and 56% of rat and human plasma protein, respectively (Davies & Morris Citation1993, Peters Citation1996). Albumin functions to maintain colloidal osmotic pressure and pH, and is able to bind and transport both endogenous compounds, such as fatty acids and bilirubin, and a wide variety of exogenous compounds and drugs (Peters Citation1996). With its high concentration in blood, and a half-life of approximately 20 days in humans and 3 days in rats (Katz et al. Citation1961, Peters Citation1996), albumin displays suitable traits for a biomarker. However, little is known about the potential for albumin binding by pesticides at toxicologically relevant exposures, or of the susceptibility of OP-bound albumin to elimination and spontaneous reactivation in vivo. We have addressed these issues by evaluating the binding of rat and human plasma albumins in vitro by commonly used OP pesticides when present as their biologically active (oxon) forms: azamethiphos (oxon), chlorfenvinphos (oxon), chlorpyrifos-oxon, diazinon-oxon and malaoxon. In addition, we have dosed rats with chlorfenvinphos (oxon), and the thion forms of two pesticides – pirimiphos-methyl and malathion – for bioactivation in vivo to their active oxon counterparts. Our results demonstrate differential pesticide binding of albumin both in vitro and in vivo at relatively low-level exposures. We further establish that rat plasma albumin binding by bioactivated pirimiphos-methyl (dimethoxy-) or chlorfenvinphos (oxon) (diethoxy-) in vivo results in OP-albumin binding that is still significant 7 days post-dosing, with a half-life similar to that for native rat albumin in either case. Our demonstration of significant albumin binding at sub-symptomatic exposure levels suggests that OP-albumin binding may indeed be suitable for exploitation as a reasonably long-lived biomarker of exposure to several OPs.
Materials and methods
The organophosphorus pesticides azamethiphos (oxon) (S-6-chloro-2,3-dihydro-2-oxo-1,3-oxazolo[4,5-b]pyridin-3-ylmethyl O,O-dimethyl phosphorothioate), chlorfenvinphos (oxon) (2-chloro-1-(2,4-dichlorophenyl)vinyl diethyl phosphate) and malathion (diethyl (dimethoxyphosphinothioylthio)succinate) and its corresponding oxon (malaoxon) were bought from QMX Laboratories Ltd., Thaxted, UK. All compounds were at 95–99.5% purity. Chlorpyrifos (O,O-diethyl-O-(3,5,6-trichloro-2-pyridyl)phosphorothioate) and diazinon (O,O-diethyl O-2-isopropyl-6-methylpyrimidin-4-yl phosphorothioate) as their corresponding oxons, and pirimiphos-methyl (O-2-diethylamino-6-methylpyrimidin-4-yl O,O-dimethyl phosphorothioate) were purchased from Greyhound Laboratories, Birkenhead, UK. All compounds were at 97.2–99.4% purity. For in vitro assays, pesticides were prepared as 100 mM stock solutions in ethanol (Sigma, HPLC grade, <0.10% water), except azamethiphos (oxon) which was at 50 mM, and were stored at 4°C for up to 2 weeks. Pesticides were diluted in phosphate-buffered saline to required concentrations just prior to use. Tritiated-diisopropylfluorophosphate (3H-DFP) at a specific activity of 150 GBq mmol−1 was purchased from Perkin Elmer, Boston, USA. The commercially purified albumins used for in vitro assays; rat albumin (A6272, purity >96%) and human albumin (A1653, purity 96–99%) were purchased from Sigma as were dithiothreitol (DTT), polyethylenimine solution and recombinant human AChE (C1682).
Blood and tissue preparations
Fifty-two male F344 strain rats weighing between 200 and 230 g were used for experiments. Rats were maintained in cages (four per cage) under controlled temperature (21±1°C) and light (16 h light/8 h dark cycle) with ad libitum access to food intake and water. All animal procedures were approved by the University of Nottingham Local Ethical Review Committee and were carried out in accordance with the Animals Scientific Procedures Act (UK) 1986.
Rats were dosed orally by gavage with OPs in arachis oil at 25% of their respective LD50 values. Thus 15 rats were given 354 mg kg−1 pirimiphos-methyl; 10 rats 2.5 mg kg−1 chlorfenvinphos (oxon); and 5 rats 450 mg kg−1 malathion. Control animals were given 1 ml kg−1 arachis oil only (12 rats). In an additional experiment to investigate different OP pesticide doses, 5 rats were given 1.25 mg kg−1 chlorfenvinphos (oxon) (12.5% of the LD50), and 5 others 8.0 mg kg−1 chlorfenvinphos (oxon) (80% of the LD50). One day after dosing rats were anaesthetised with isoflurane and 100 − 500 µl of blood removed from a tail vein. Alternatively, 1, 3 or 7 days after rat dosing approximately 5 ml of blood was removed by terminal intracardiac puncture. Heparinised blood was retained on ice before centrifugation at 2000g for 10 min at 4°C to pellet the red blood cells. The supernatant plasma was removed and stored at −80°C, and likewise the erythrocytes were decanted and stored at −80°C until required. Control human blood (taken with University of Nottingham Ethical Review Committee approval) from one of the authors (male, 39 years of age) was collected into heparin and similarly centrifuged to prepare erythrocytes and plasma.
Thymus and brain tissues were removed from control or dosed rats after saline perfusion, as described in Carter et al. (Citation2007).
Protein concentrations
Protein concentrations were measured using the DC Protein assay (Biorad) using bovine serum albumin as a protein standard.
Acetylcholinesterase measurements
Inhibition of erythrocyte, thymus, or brain tissue AChE activity was measured based upon the spectrophotometric method described by Ellman et al. (Citation1961). Spectrophotometric measurements were conducted at 412 nm in a Perkin Elmer Lambda 2S spectrophotometer operated using UV KinLab software. Replicate analyses for each assay sample point were conducted for 5 min at 37°C, with the rate of production of 5-thio-2-nitro-benzoic acid calculated, and averaged for the replicate readings. Erythrocytes were diluted 1:100 in 10 mM Tris/HCl pH 8.0 buffer for AChE measurements, brain was diluted 1:10 with the same buffer, and thymus tissue was used without dilution.
Vacuum filtration of radiolabelled albumin and quantitation of radiolabelling
Plasma radiolabelled with 3H-DFP was separated by filtration on GF/B (25 mm) glass microfibre filters (Whatman) using a vacuum manifold. Filters were initially charged by soaking in 0.3% (w/v) polyethylenimine for 30 min. Charged filters were then applied to a vacuum manifold and washed with 10 mM Tris/HCl pH 8.0. Typically 10 µg of plasma proteins was loaded onto a single filter, washed with 50 ml of 10 mM Tris/HCl pH 8.0, air dried, and then counted for radioactivity within inserts containing 3 ml of scintillant. All assay points were performed in duplicate. Tritiated-DFP which was retained on the membranes in the absence of plasma proteins; approximately 1000 dpm, constituting 15±0.3% of that for control plasma radiolabelling, was used as a blank and subtracted from all values.
One-dimensional SDS-PAGE and albumin autoradiography
Typically 20 µg of plasma was incubated with pesticide or phosphate-buffered saline as solvent for 20 min at room temperature. Proteins were then radiolabelled by incubation in a final volume of 130 µl with 19 µM 3H-DFP (final concentration) for 1 h at 37°C typically at a molar ratio of 3H-DFP:albumin of 8:1, or as described in individual figure legends. Proteins were heated for 10 min at 70°C in sample buffer (Novex) containing 100 mM DTT. Proteins were separated on 4–12% Bis-Tris (Novex) gels run with 3-(N-morpholino)propanesulfonic acid (MOPS) buffer, then electroblotted onto a polyvinylidene difluoride membrane, cross-linked, and 14C markers applied to the blot to identify the positions of the molecular weight standards. Blots were subjected to 7–24 h of autoradiography within a highly sensitive microchannel plate detector as detailed in Carter et al. (Citation2007). Protein radiolabelled bands from autoradiographic images were quantified using Quant scan software (Beta autoradiographic image acquisition software), with band intensities (in pixels) plotted using excel to determine relative radiolabel incorporations.
Statistical analysis
For comparison of the levels of radioactivity incorporated into albumin, a one-way analysis of variance (ANOVA) with post-hoc test was performed (Bonferroni's multiple comparison test).
Results
Albumin is differentially bound by organophosphorus pesticide-oxons in vitro at toxicologically relevant doses
Rat plasma was diluted to 320 µg ml−1 and preincubated with pesticide-oxon or solvent for 20 min in vitro at concentration/times confirmed to produce approximately 30% inhibition of thymus tissue AChE activity (Carter et al. Citation2007). The actual concentrations used were: azamethiphos (oxon) 0.12 µM, chlorfenvinphos (oxon) 1.5 µM, chlorpyrifos-oxon 0.03 µM, diazinon-oxon 0.04 µM and malaoxon 0.1 µM. Residual active hydrolase groups were then adducted by incubation with 19 µM 3H-DFP for 1 h at 37°C. Radiolabelled proteins (typically 10 µg per data point) were loaded onto glass microfibre filters and washed extensively to remove extraneous unincorporated 3H-DFP. After drying, filters were counted for radioactivity and both the maximal level of 3H-DFP incorporation and the level of pesticide binding (fall in radioactivity) quantified (A). Alternatively, radiolabelled plasma proteins were subjected to one-dimensional SDS-PAGE and autoradiography. After radiolabelling of 20 µg of rat plasma only a single radiolabelled protein was present after protein separation by denaturing SDS-PAGE. This radiolabelled albumin migrated as a discrete band of approximate molecular weight 66 kDa at the leading edge of the main Coomassie-stainable albumin (B). This band was confirmed as serum albumin by its removal by immunoprecipitation; localisation by Western blotting using an antialbumin antibody (Autogen bioclear, ABN 192); and also by peptide mass fingerprinting of the excised protein by matrix-assisted laser-desorption time-of-flight mass spectrometry (results not included).
Figure 1. 3H-DFP incorporation into rat plasma albumin after preincubation with pesticide-oxons. Rat plasma was incubated with pesticide-oxons or solvent at 20 min thymus tissue AChE IC30 concentrations. Reactive hydrolase groups were then adducted by incubation with 3H-DFP for 1 h at 37°C. (A) Ten micrograms of radiolabelled plasma was loaded onto glass microfibre filters, washed, and the incorporation of 3H-DFP determined. Results are presented as the mean±standard error from at least 16 independent experiments with each pesticide. Results were significantly different from controls with chlorfenvinphos (oxon), chlorpyrifos-oxon, and diazinon-oxon (*** p <0.001). (B) Twenty micrograms of radiolabelled plasma was resolved by SDS-PAGE, and then transferred to a polyvinylidene difluoride membrane. Proteins were stained with Coomassie brilliant blue (left panel) or autoradiographed (right panel). The positions of protein molecular weight markers are shown in the first two lanes of the gel and blot. A single radiolabelled protein band was evident (marked with an arrowhead) which superimposed with the leading edge of the albumin protein band detected from protein staining. Incubation with the pesticide-oxons of chlorfenvinphos, chlorpyrifos, and diazinon significantly reduced 3H-DFP incorporation in to this radiolabelled protein.
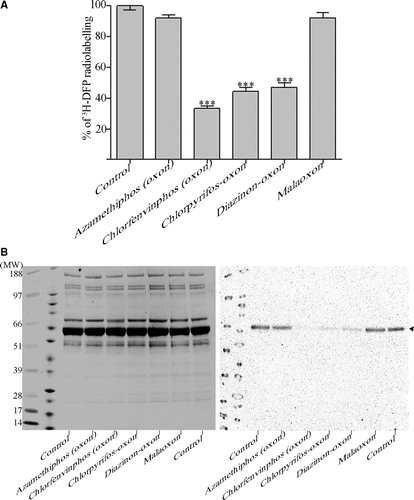
From either quantitation of 3H-DFP radiolabelled albumin retained on microfibre filters (A), or from autoradiography of gel-resolved protein (B), plasma albumin was shown to be bound in vitro by the oxons of chlorfenvinphos (67±2%), chlorpyrifos (56±2%) and diazinon (54±2%), but not azamethiphos (9±1%) or malathion (8±1%) relative to controls. Comparison of the proportion of albumin radiolabelled (by autoradiography) with the total protein present (visualised by Coomassie blue protein staining) showed that only a small proportion of albumin molecules were bound by 3H-DFP under the conditions employed. The level of 3H-DFP binding was quantified as approximately 1% of albumin molecules using the microfibre binding and quantitation method. This calculation assumes that protein recovery by this method was 100%, and so may have somewhat underestimated the true stoichiometry, but these filters are known to achieve high protein recovery (Bruns et al. Citation1983).
To validate this stoichiometry calculation by an independent means, we also radiolabelled rat plasma albumin with 3H-DFP alongside 3H-DFP radiolabelled recombinant AChE. Radiolabelled proteins were then resolved by gel electrophoresis and subjected to autoradiography using a microchannel plate detector, a device with known autoradiographic signal linearity over six orders of magnitude (Richards & Lees Citation2002). Both proteins have a similar molecular weight but AChE can incorporate organophosphates stochiometrically, i.e. one mole of organophosphate bound per mole of AChE (Raveh et al. Citation1989). A signal of 3640 arbitrary pixel intensity units was generated from 24 h autoradiography of 0.2 µg of 3H-DFP radiolabelled AChE, whereas 3000 intensity units arose from 20 µg of 3H-DFP radiolabelled albumin (images not included). Assuming 100% binding of 3H-DFP to AChE, 3H-DFP binding to albumin was therefore quantified as 0.82% of available albumin molecules, a value close to the 1% binding evidenced from retention of 3H-DFP radiolabelled albumin on microfibre filters.
Since 3H-DFP binding to albumin survived denaturing SDS-PAGE, it was presumed covalent in nature and not transient or readily reversible. That this was also true of the unlabelled test pesticides was evidenced by the reduction in 3H-DFP labelling of albumin produced by preincubation with pesticide being unchanged after extensive (24 h) dialysis to remove free OP prior to 3H-DFP radiolabelling (results not included).
The absolute level of in vitro albumin binding by 3H-DFP was related to the 3H-DFP:albumin molar ratio, time of incubation, and albumin source and purification
We sought an explanation of this relatively low 3H-DFP albumin binding stoichiometry, since other literature reports have suggested higher reactivity potential (Murachi Citation1963, Means & Wu Citation1979). If the reaction with 3H-DFP was dependent upon both the molar ratio of 3H-DFP to albumin, and also the time of incubation with 3H-DFP, then increasing either should increase the radiolabelling of albumin. We incubated diluted rat plasma (320 µg ml−1 final concentration) with 3H-DFP at a final concentration range of 5.5 µM to 19 µM. At this rat plasma dilution, albumin is at a concentration of approximately 2.3 µM giving a molar ratio of approximately 2:1 to 8:1 moles of 3H-DFP:moles of albumin, respectively. Increasing the molar ratio of 3H-DFP to plasma albumin did indeed increase the level of 3H-DFP binding () (control row). Across this range of 3H-DFP:albumin molar ratios, preincubation with pesticides in vitro (under conditions producing approximately 30% thymus tissue AChE inhibition) produced a similar differential binding pattern as that seen with 19 µM 3H-DFP (, and A, B). The absolute magnitude of the pesticide binding increased with 3H-DFP concentration, although not proportionately. This suggested that not all of the additional pesticide binding sites disclosed by the higher 3H-DFP concentrations were targeted by the pesticides (see ).
Table I. Stiochiometry of 3H-DFP incorporation into rat plasma albumin. Rat plasma albumin (2.3 µM) was incubated with solvent or pesticide-oxons at 20 min AChE IC30 concentrations, and then radiolabelled with 5.5, 10 or 19 µM 3H-DFP. Both the increase in albumin radiolabelling from increasing 3H-DFP concentration, and the level of pesticide binding relative to controls was determined by quantifying the level of radiolabelled albumin retained on glass microfibre filters.
To examine the effect of increasing the time of incubation of 3H-DFP with plasma albumin at a given 3H-DFP:albumin molar ratio (8:1), 3H-DFP was incubated with plasma albumin at 37°C for a time course of up to 4 days. Plasma samples were removed at time points during the incubation period, and the level of 3H-DFP incorporation into albumin quantified by counting 3H-DFP-radiolabelled albumin retained on microfibre filters. As 3H-DFP is unstable after 24 h in aqueous solutions (Hobbiger Citation1951) additional 3H-DFP was added to the reaction mixture at intervals of 24 h. The level of 3H-DFP incorporation into rat plasma albumin increased in a roughly linear fashion over the first 4 h but without a further increase at 24 h, indicative of 3H-DFP hydrolysis. However, after supplementation with additional 3H-DFP after the 24 h time point, and daily thereafter, an approximately linear 3H-DFP incorporation was seen (). This confirmed that albumin could be radiolabelled at a higher stiochiometry if incubation times with 3H-DFP were extended. This increase in 3H-DFP radiolabelling was not a result of denaturation of albumin over the time course, since preincubation of plasma albumin alone for 4 days prior to radiolabelling had no influence on the level of subsequent 3H-DFP incorporation, suggesting that the binding site was still intact and that the relatively low binding stoichiometry was indeed a result of slow 3H-DFP binding (results not included).
Figure 2. Time course of 3H-DFP incorporation into rat plasma albumin. Rat plasma (300 µg) was reacted at 37°C with 19 µM 3H-DFP (19 nmoles) in a buffer of 10 mM Tris/HCl pH 8.0 containing 1 mM EDTA, 5 mM DTT, 5% glycerol (1000 µl final volume). At intervals of 1, 2, 4, 24, 48, 72, and 96 h, replicate 50 µl samples were removed and the level of 3H-DFP incorporated into albumin quantified by counting the radiolabelled albumin retained on glass microfibre filters. To counter 3H-DFP hydrolysis during the time course, the reaction mixture was supplemented with 7.2 nmoles of 3H-DFP after 24 h, 4.8 nmoles of 3H-DFP after 48 h, and 2.4 nmoles of 3H-DFP after 72 h. Data points are mean±standard deviation from four independent experiments.
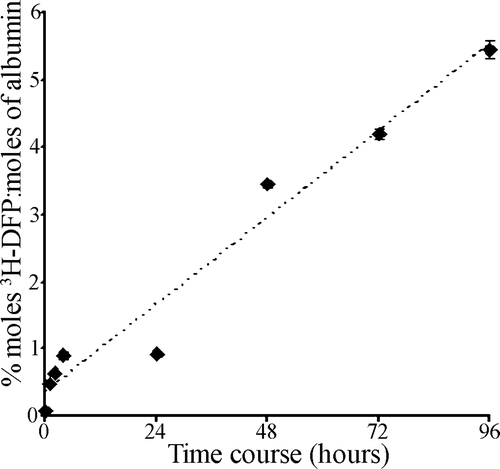
Other groups have analysed the binding of DFP and/or pesticides in vitro using commercially purified bovine or human albumin which are essentially fatty acid free (Means & Wu Citation1979, Hagag et al. Citation1983, Peeples et al. Citation2005, Li et al. Citation2007). Commercially available albumins (Fraction V) undergo purification conditions that include a series of alcohol fractionations (Peters Citation1996), conditions that would be expected to induce albumin conformational changes which may result in increased susceptibility of the active site tyrosine to 3H-DFP binding. To test this hypothesis we radiolabelled human plasma, and commercially purified rat and human albumins with 19 µM 3H-DFP for 1 h at 37°C, and compared the level of radioactivity incorporated with that from our rat plasma studies (). Interestingly, human plasma albumin was radiolabelled by 3H-DFP to a higher stiochiometry than rat plasma approaching 4% of albumin molecules, but the absolute reduction in labelling seen after OP preincubations was not significantly greater, suggesting that these additional 3H-DFP binding sites were not sensitive to the pesticides tested. For both rat and human commercial albumins, the level of 3H-DFP binding stoichiometry increased by approximately 5-fold over the native forms, suggesting that commercial albumin purification may result in albumin molecules with increased accessibility of their active site to binding by 3H-DFP.
Table II. Stiochiometry of 3H-DFP incorporation into rat and human albumins. Rat or human albumin (1–2 µM) was incubated with solvent or pesticide-oxons at 20 min AChE IC30 concentrations, and then radiolabelled with 19 µM 3H-DFP. The level of albumin radiolabelling was quantified by counting radiolabelled albumin retained on glass microfibre filters. For rat plasma albumin, n=16, and for all other data points n=4–6 experiments.
Albumin binding by pesticide-oxons in vitro was proportional to pesticide concentration, and was of a comparable sensitivity to pesticide binding of AChE
The effect of varying concentrations of the albumin-binding pesticides chlorfenvinphos (oxon), chlorpyrifos-oxon and diazinon-oxon on the 3H-DFP radiolabelling of rat plasma albumin and the inhibition of AChE activity were examined in parallel incubations. Rat plasma (at a protein concentration of 203 µg ml−1), or recombinant AChE, were preincubated with pesticides for 20 min over a concentration range of 1 nM – 200 µM for chlorfenvinphos (oxon), 1nM – 100 µM for chlorpyrifos-oxon, and 1nM – 100 µM for diazinon-oxon. The level of AChE inhibition was quantified by the Ellman assay (Ellman et al. Citation1961). After pesticide preincubations rat plasma proteins were radiolabelled with 17 µM 3H-DFP for 1 h at 37°C, and the incorporation of radioactivity into albumin quantified by counting 3H-DFP-radiolabelled albumin retained on microfibre filters, and also visualised after autoradiography of SDS-PAGE separated proteins (A–C).
Figure 3. Comparison of pesticide binding of albumin with inhibition of AChE. Rat plasma (203 µg ml−1) was incubated with pesticide or solvent for 20 min at room temperature over the pesticide concentration ranges shown. Proteins were then radiolabelled with 17 µM 3H-DFP for 1 h at 37°C. The radiolabel incorporated into albumin was quantified by counting radiolabelled albumin retained on glass microfibre filters. The corresponding inhibition of AChE at each concentration was quantified using the Ellman assay, with the results presented graphically. Radiolabelling of albumin was also visualised after SDS-PAGE and autoradiography and is shown in the lower panels. (A) Chlorfenvinphos (oxon), (B) chlorpyrifos-oxon, (C) diazinon-oxon.
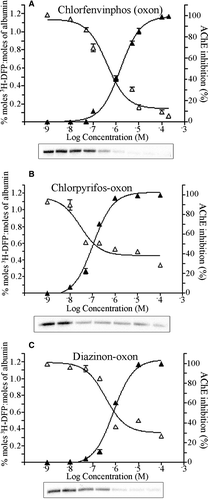
Both albumin binding and AChE inhibition were proportional to pesticide concentrations. For chlorfenvinphos (oxon), the IC50 for AChE and albumin binding were approximately 1.5 and 0.4 µM; for chlorpyrifos-oxon, 0.04 and 0.1 µM; and for diazinon-oxon, 0.5 and 2 µM, respectively. Thus albumin exhibited an in vitro sensitivity to binding by these pesticide-oxons that was similar to (and for chlorfenvinphos (oxon) greater than) that of AChE inhibition.
Albumin is bound by pirimiphos-methyl and chlorfenvinphos (oxon) in vivo with half-lives comparable to that of native rat albumin
Having established that albumin binding by certain pesticides is seen in vitro at toxicologically relevant pesticide exposures, we investigated albumin binding in vivo, and also whether pesticide binding to albumin is sufficiently stable to provide a biomonitor of pesticide exposure.
Rats were dosed with solvent (controls), or with pesticides at 25% of their LD50: 354 mg kg−1 pirimiphos-methyl (a thion for bioactivation to its oxon counterpart in vivo), and 2.5 mg kg−1 chlorfenvinphos (oxon). Rats remained asymptomatic at these dose levels. Blood was removed at 1, 3, or 7 days after dosing, and plasma albumin radiolabelled with 19 µM 3H-DFP for 1 h at 37°C. The incorporation of radioactivity into albumin was quantified by counting 3H-DFP-radiolabelled albumin retained on microfibre filters (A, B). In addition, radiolabelled albumin was resolved by SDS-PAGE and visualised by autoradiography. An example of the level of radiolabel incorporated into albumin in one rat from each group is also included in A and B (lower panels). Erythrocytes from the rats were used to determine the corresponding level of AChE inhibition arising from each dose by the Ellman assay.
Figure 4. Quantitation of dimethoxy- and diethoxy-pesticide binding of albumin in vivo. Rats were treated with solvent (controls) or dosed with 25% of the LD50 of pirimiphos-methyl (A), or 25% of the LD50 of chlorfenvinphos (oxon) (B). After radiolabelling with 3H-DFP the incorporation of radioactivity into albumin was quantified by counting the radiolabelled albumin retained on glass microfibre filters. Results are presented as the mean±standard error from at least four independent experiments from each rat. Results were significantly different from controls at 1, 3, and 7 days after dosing (***p <0.001) for both pesticides. Radiolabelled albumin was also resolved by SDS-PAGE, and an example of the level of radioactivity incorporated into albumin for each dose condition displayed in the lower panels. The average level of erythrocyte AChE inhibition for each dosing condition is also included.
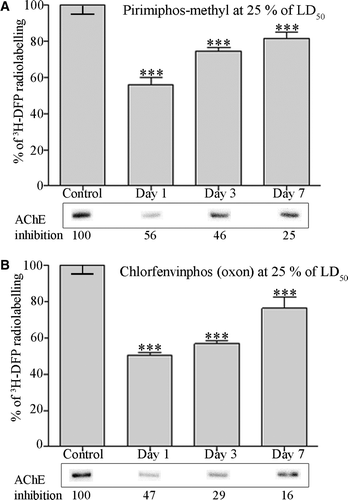
When plasma albumin was analysed 1 day after dosing, both pirimiphos-methyl and chlorfenvinphos (oxon) had inhibited approximately 50% of the erythrocyte AChE activity, with a corresponding inhibition of binding of approximately 50% of the 3H-DFP-radiolabelled albumin molecules. This level was comparable to the ‘maximal’ pesticide binding achieved from our in vitro studies. Analysis of the elimination of pesticide binding to albumin over a 1 week post-dosing time course, revealed that both pirimiphos-methyl (dimethoxy-) and chlorfenvinphos (oxon) (diethoxy-) binding steadily returned toward control levels, but was still significant 3 and 7 days after dosing.
A natural logarithmic plot of the elimination of the pesticide binding to albumin for pirimiphos-methyl or chlorfenvinphos (oxon) indicated half-lives of at least 3 days (graph not included), suggesting that pesticide-bound albumin was not actively degraded or unstable, but followed a similar rate of turnover to that described for native rat albumin (Peters Citation1996, Troester et al. Citation2002).
Albumin is not bound by malathion in vivo but is maximally bound by chlorfenvinphos (oxon) even at low AChE inhibitions
To establish whether the differential pesticide binding of albumin that we have documented in vitro was operable in vivo, rats were dosed with malathion (at approximately 25% of the LD50), a thion for bioactivation in vivo to its oxon counterpart, malaoxon – a pesticide that did not significantly bind albumin in vitro. In addition, to determine if albumin binding by chlorfenvinphos (oxon) was increased at a higher pesticide dose, or decreased with a lower dose, rats were given either 80% or 12.5% of the LD50. The higher dose, but not the lower dose, produced salivation and muscle fasciculation in all rats. Twenty-four hours after dosing rat blood was removed, and the level of albumin binding quantified by counting the 3H-DFP-radiolabelled albumin retained on microfibre filters, and the corresponding levels of erythrocyte AChE inhibition determined by the Ellman assay (). For each of the chlorfenvinphos (oxon) doses analysed, approximately 50% of the 3H-DFP radiolabelled albumin molecules were bound, suggesting that this level of binding was also ‘maximal’ for the in vivo exposure duration. Moreover, even at the lowest AChE inhibition (22%) achieved with the lowest chlorfenvinphos (oxon) dose, albumin binding was still maximal, supporting our in vitro studies that chlorfenvinphos (oxon) binding to albumin was at least, if not more, sensitive than binding to AChE. By contrast, no significant binding of albumin by malathion was evident (despite 57% AChE inhibition), also in agreement with our in vitro studies, that malathion, or its bioactivated oxon (malaoxon), were not capable of significantly binding albumin.
Figure 5. Albumin is maximally bound across a range of chlorfenvinphos (oxon) doses in vivo, but is not significantly bound by malathion in vivo. Rats were treated with solvent (controls) or dosed with 12.5%, 25%, and 80% of the LD50 of chlorfenvinphos (oxon), or approximately 25% of the LD50 of malathion. Twenty-four hours after dosing plasma albumin was radiolabelled with 3H-DFP, and the incorporation of radioactivity into albumin quantified by counting the radiolabelled albumin retained on glass microfibre filters. Results are presented as the mean±standard error from at least four independent experiments from each rat. Results significantly different from controls are marked (***p <0.001). Radiolabelled albumin was also visualised by autoradiography after SDS-PAGE, and an example of the level of radioactivity incorporated into albumin shown in the lower panels. The average level of erythrocyte AChE inhibition for each dosing condition is also included.
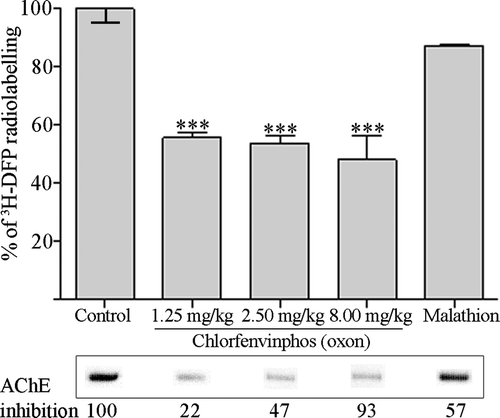
Discussion
In order to assess the potential for albumin to act as a biomarker for a range of pesticides in common use within the UK or USA, we used DFP labelling to quantify the levels of pesticide binding to rat albumin primarily at sub-symptomatic OP exposure levels. After pesticide preincubations in vitro sufficient to produce 30% thymus tissue AChE inhibition, marked albumin binding was demonstrated with the oxons of chlorfenvinphos, chlorpyrifos and diazinon, but not with those of azamethiphos or malathion. This binding was confirmed as covalent by extensive dialysis prior to 3H-DFP incubation. The other major characterised 3H-DFP target proteins in plasma, AChE and BuChE, are at very low concentrations relative to albumin so, although they will also have been reacted with these pesticides, their contribution to the total level of binding calculated would be insignificant. For comparison, albumin is at an approximate plasma protein concentration of 32 mg ml−1 in rats and 42 mg ml−1 in humans (0.5–0.6 mM) (Davies & Morris Citation1993), whereas the human concentration of plasma BuChE has been estimated at 3.3 µg ml−1, or approximately 50–80 nM (Brimijoin & Hammond Citation1988, van der Schans et al. Citation2004). For AChE, the human plasma concentration has been estimated as 8 ng ml−1 (Brimijoin & Hammond Citation1988). That the binding of pesticides to these two cholinesterases was not significant under our assay conditions was confirmed experimentally by preincubation of plasma with the AChE inhibitor eserine (Sigma E8375), or the BuChE inhibitor ethopropazine (Sigma L308765), which in neither case lowered radioactivity incorporated into plasma albumin from that of control levels (results not included).
In order to evaluate the functional significance of this albumin binding, it is necessary to estimate the absolute stoichiometry; however, as radiolabelled pesticides were not available, we achieved this indirectly via 3H-DFP binding. Clearly DFP is not an ideal ligand for this purpose, since after 1 h incubation with 19 µM 3H-DFP only approximately 1% of rat and 4% of human albumin molecules were radiolabelled via calculation of the retention of 3H-DFP-radiolabelled albumin on microfibre filters.
However, increasing the molar ratio of 3H-DFP to albumin, extending the time course with 3H-DFP or replacement of native with commercially purified albumin, all increased the level of 3H-DFP radiolabelling of albumin. This concurs with the results described by other groups detailing higher stoichiometries of binding after either prolonged and/or high concentrations of inhibitor used under assay conditions of above pH 8.0 which facilitate binding-site accessibility, and/or from incubations with commercially prepared albumins from which fatty acids have been stripped (Murachi Citation1963, Means & Wu Citation1979, Hagag et al. Citation1983, Li et al. Citation2007). However, this higher level of albumin binding would not be expected to be seen with native albumin in vivo.
The low stiochiometry of radiolabelling of albumin that we report may reflect an inaccessibility of the active site to adduction by 3H-DFP. In contrast to the more usual adduction of active-site serine hydrolases, DFP and OPs bind an active-site tyrosine in serum albumin (tyrosine 411 of human or rat albumin) (Sanger Citation1963, Means & Wu Citation1979, Black et al. Citation1999, Schopfer et al. Citation2005, Li et al. Citation2007). Since this active-site tyrosine is also a point of ionic contact for associated molecules including both monounsaturated oleic acid and polyunsaturated arachidonic acid, two fatty acids that normally associate with albumin in serum (Petitpas et al. Citation2001), DFP binding may certainly be conformationally restricted by the presence of these or similar fatty acids (Means & Wu Citation1979, Petitpas et al. Citation2001), a restriction which may be lost in commercial albumin preparations. We did not investigate this further, concentrating our studies on interactions with the native, fatty acid bound albumin which is present in vivo.
Marked differences between the levels of binding of the different OPs to albumin were evident from both our in vitro and in vivo results, in contrast to the uniform binding shown by the majority of agents tested at the very high exposure levels used by others (Peeples et al. Citation2005). The structures of the OPs used in this study are shown in , and divide into relatively low and high albumin-binding structures. Although these OPs were selected on the basis of pesticide usage rather than structure, it is apparent that those that possess an ester linkage to the leaving group readily bind albumin, whereas those with a thioester are virtually unreactive. Hence we might predict that other similarly structured dimethoxy triesters in which the leaving group is thioester linked, such as azinphos-methyl and possibly its diethoxy triester counterpart azinphos-ethyl, would be unreactive with albumin under conditions in which similarly structured esters were reactive. This prediction, like the mechanistic basis for albumin's differential pesticide reactivity, will need to be tested via further experimentation.
The functional significance of albumin binding by OPs is unclear at present. There are a number of enzymatic activities intrinsic to serum albumin that have the potential to counter certain xenobiotics, of which the OP-binding or fatty acid binding site, tyrosine 411, may also be an enzymatically active site residue (Means & Wu Citation1979, Hagag et al. Citation1983, Sultatos et al. Citation1984, Sogorb et al. Citation1998, Watanabe et al. Citation2000, Kragh-Hansen et al. Citation2002). However, the majority of these enzymatic activities are relatively weak, or only operate in the absence of fatty acids and/or at pHs above the normal physiological range (Ortigoza-Ferado et al. Citation1984). Nonetheless, irrespective of its relative enzymatic inabilities, albumin does constitute the major plasma protein; hence, if only 1% of albumin molecules in adults are physiologically active to sequester OPs or catalytically hydrolyse OPs (act as an A-esterase) or other xenobiotics, albumin will still exert considerable influence on the free circulatory OP/xenobiotic concentration, and therefore its tissue distribution, liberation, elimination, and thus detoxification. This has recently been substantiated, at least in vitro, by a demonstration of the detoxification of the carbamate pesticide carbaryl by bovine serum albumin at toxicologically relevant concentrations (Sogorb et al. Citation2007). It is noteworthy that this protection from neurotoxicity that binding of OPs to albumin affords, may well be compromised in the fetus and newborn since they possess lower concentrations of albumin and other serum proteins (Qiao et al. Citation2001).
The binding of OP pesticides to albumin will also have the potential to compete and/or displace endogenous and exogenous albumin-bound compounds, including an array of drugs that also bind albumin at the same region of the molecule, hence influencing drug metabolism and pharmacokinetics (Watanabe et al. Citation2000, Kragh-Hansen et al. Citation2002). Furthermore, since human albumin has the second highest number of allelic mutants after haemoglobin including residues adjacent to the OP-binding site (referenced at the Expasy website: http://au.expasy.org/uniprot/P02768, or at http://www.albumin.org/), with some mutants influencing fatty acid (or drug) binding (Nielsen et al. Citation1997), then presumably the binding of OPs to albumin may also be variable within the human population, and thereby influence an individual's ability to detoxify pesticides.
Once bound, an OP-adduct may undergo three fates: it may remain at the binding site until the protein is either turned over or actively degraded; spontaneous removal from the target by hydrolysis which results in the reformation of the native hydroxy amino acid; or ageing, whereby an alkyl side chain of the inhibitor is lost (dealkylation) and the resulting enzyme-inhibitor complex becomes resistant to enzyme regeneration by fluoride ions or oximes. The relative rates of protein turnover/degradation, spontaneous reactivation, or ageing will determine the persistence of OP binding, and hence it's usefulness as a biomarker of OP exposure.
The rates of ageing and spontaneous reactivation are specific to the bound phosphoryl group. For AChE, the stability and resistance to spontaneous reactivation of O,O’-dimethyl is less than O,O’-diethyl, which is less than O,O’-dipropyl bound counterparts, but structurally similar phosphoryl groups should all reactivate at approximately the same rate if bound to the same enzyme (Hobbiger Citation1951, Davison Citation1955). In the present study we examined dimethoxy- and diethoxy-albumin binding. The diethoxy pesticide binding was greater than that for the dimethoxy pesticide at 3 and 7 days after dosing which presumably represents enhanced resistance to spontaneous reactivation. However, for either pesticide the levels of pesticide binding were still significant 7 days after dosing, indicating good potential for both structures to provide post-exposure biomonitoring information. In the absence of sufficient data collection points it is difficult to accurately calculate the true half-lives of this pesticide to albumin binding, but since stable albumin adducts should follow a first-order rate of elimination (Granath et al. Citation1992, Troester et al. Citation2002), it is clear that pesticide albumin binding is not unstable but maintained at least as long as the approximately 3 day half-life of native rat albumin (Katz et al. Citation1961, Peters Citation1996).
By comparison, estimates of erythrocyte cholinesterase inhibited either in vitro or in vivo indicate a half-life of spontaneous reactivation of 1–2 h with dimethoxy OPs (Aldridge & Davison Citation1953, Vandekar & Heath 1956, Blaber & Creasey Citation1960, Worek et al. Citation1999), and approximately 2 days with diethoxy pesticides (Blaber & Creasey Citation1960, Mason et al. Citation2000). Other estimates of spontaneous reactivation of erythrocyte cholinesterase inhibited by dimethoxy pesticides have suggested a half-life of as much as 37 h (Mason et al. Citation2000). The levels of inhibitor used in these assays may contribute to the differences in the values attained for the dimethoxy phosphate esters due to sustained enzyme inhibition, and in practice, inhibition can be prolonged by ageing, but particularly for dimethoxy adducts plasma albumin clearly has a usefully long duration of binding relative to AChE. It will remain for future studies to evaluate the true rates of spontaneous reactivation of human albumin OP-bound in vivo.
Pesticide binding to albumin may not totally return to control levels due to ageing. However, the usefulness of albumin for retaining OP adduct information has been demonstrated from both in vitro and in vivo studies which have shown that albumin was resistant to ageing (and thereby susceptible to fluoride or oxime reactivation) after active-site occupancy by DFP, OPs or a number of nerve agents (Black et al. Citation1999, Adams et al. Citation2004, Li et al. Citation2007, Williams et al. Citation2007).
Human albumin has an estimated half-life of approximately 20 days (Peters Citation1996, Chaudhury et al. Citation2003), a life-span suitably persistent for biomarker sampling. However, at present we can only presume that the pesticide albumin binding stability that we observed for rats would also be seen in humans.
For comparison, the half-life of recovery of BuChE from the dimethoxy OP, dichlorvos, was approximately 12 days (Mason Citation2000), similar to the estimates of this protein's half-life (Munkner et al. Citation1961, Ostergaard et al. Citation1988). Erythrocyte AChE, undergoes a steady (linear) recovery from dichlorvos, returning to control levels after approximately 82 days – a little prior to the life-span of an erythrocyte (approximately 120 days), which demonstrates its value for monitoring relatively long-lasting OP inhibitions (Mason Citation2000).
In summary, the results described in this paper quantify binding of low levels of certain pesticides to rat serum albumin that persists beyond 7 days post-exposure, and suggest that the relatively high protein concentration of albumin, coupled to its relatively long half-life, make it a suitable biomonitor target for certain pesticides. Currently, use of gas chromatography to identify products from fluoride reactivation of BuChE-OP adducts provides a sensitive method for post-exposure OP detections (Polhuijs et al. Citation1997, van der Schans et al. Citation2004). However, this method is not without limitations, as OP-BuChE adducts are susceptible to ageing with associated loss of amenability to the reactivation process. Conversely, albumin adducted by OPs and some nerve agents is resistant to ageing thereby maintaining OP-adduct information which has been detected by a number of mass spectrometry-based methods (Black et al. Citation1999, Adams et al. Citation2004, Li et al. Citation2007, Williams et al. Citation2007). In future work it will be important to determine the in vivo threshold at which pesticide binding to albumin can be detected in order to further characterise albumin-binding sensitivity. However, mass spectrometric methods, or an enzyme-linked immunosorbent assay utilising antibodies specifically directed against albumin-OP adducts rather than the parent OP compound, will probably provide a more practicable means for quantifying the extent of OP-albumin binding, thereby avoiding the use of radioactive DFP.
The tendency of specific OP pesticides to differentially bind albumin and other non-AChE targets could be exploited via a panel of immune assays to gain information about the specific agents to which persons have been exposed. Thus malaoxon would adduct AChE but not albumin or acylpeptide hydrolase (Richards et al. Citation2000), whereas diazinon-oxon would bind albumin and AChE, but not acylpeptide hydrolase. This approach may alleviate the base-line problems associated with cholinesterase activity measurements that arise in the absence of pre-exposure values, and therefore help to provide a better means of correlating specific OP pesticide exposures with reported ill-health.
Acknowledgements
This work was funded by a UK Medical Research Council programme grant, and DEFRA grant to D.E.R., and by a Libyan Government grant to M.H.T. We are grateful to Dr Stephen Alexander (Institute of Neuroscience, University of Nottingham) for the use of the filtration vacuum manifold. We are thankful to the manuscript reviewers for their helpful comments to improve the manuscript content and clarity.Declaration of interest: The authors report no conflicts of interest. The authors alone are responsible for the content and writing of the paper.
References
- Abou-Donia MB. Organophosphorus ester-induced chronic neurotoxicity. Archives of Environmental Health 2003; 58: 484–497
- Adams TK, Capacio BR, Smith JR, Whalley CE, Korte WD. The application of the fluoride reactivation process to the detection of sarin and soman nerve agent exposures in biological samples. Drug & Chemical Toxicology 2004; 27: 77–91
- Aldridge WN, Davison AN. The mechanism of inhibition of cholinesterases by organophosphorus compounds. Biochemical Journal 1953; 55: 763–766
- Blaber LC, Creasey NH. Mode of recovery of cholinesterase activity in vivo after organophosphorus poisoning. 1. Erythrocyte cholinesterase. Biochemical Journal 1960; 77: 591–596
- Black RM, Harrison JM, Read RW. The interaction of sarin and soman with plasma proteins: the identification of a novel phosphonylation site. Archives of Toxicology 1999; 73: 123–126
- Brimijoin S, Hammond P. Butyrylcholinesterase in human brain and acetylcholinesterase in human plasma: trace enzymes measured by two-site immunoassay. Journal of Neurochemistry 1988; 51: 1227–1231
- Bruns RF, Lawsonwendling K, Pugsley TA. A rapid filtration assay for soluble receptors using polyethylenimine-treated filters. Annals of Biochemistry 1983; 132: 74–81
- Carter WG, Tarhoni M, Rathbone AJ, Ray DE. Differential protein adduction by seven organophosphorus pesticides in both brain and thymus. Human & Experimental Toxicology 2007; 26: 347–353
- Casida JE, Quistad GB. Organophosphate toxicology: safety aspects of nonacetylcholinesterase secondary targets. Chemical Research in Toxicology 2004; 17: 983–998
- Chaudhury C, Mehnaz S, Robinson JM, Hayton WL, Pearl DK, Roopenian DC, Anderson CL. The major histocompatibility complex-related Fc receptor for IgG (FcRn) binds albumin and prolongs its lifespan. Journal of Experimental Medicine 2003; 197: 315–322
- Costa LG. Current issues in organophosphate toxicology. Clinica Chimica Acta 2006; 366: 1–13
- Davies B, Morris T. Physiological parameters in laboratory animals and humans. Pharmaceutical Research 1993; 10: 1093–1095
- Davison AN. Return of cholinesterase activity in the rat after inhibition by organophosphorus compounds. 2. Comparative study of true and pseudo cholinesterase. Biochemical Journal 1955; 60: 339–346
- Ellman GL, Courtney KD, Andres V, Featherstone RM. A new and rapid colorimetric determination of acetylcholinesterase activity. Biochemical Pharmacology 1961; 7: 88–95
- Gallo MA, Lawryk NJ. Organic phosphorus pesticides. Handbook of pesticide toxicology, WJ Hayes, Jr, ER Laws, Jr. Academic Press Inc, San Diego, CA 1991; 2: 917–1123
- Granath F, Ehrenberg L, Tornqvist M. Degree of alkylation of macromolecules in vivo from variable exposure. Mutatation Research 1992; 284: 297–306
- Hagag N, Birnbaum ER, Darnall DW. Resonance energy transfer between cysteine-34, tryptophan-214, and tyrosine-411 of human serum albumin. Biochemistry 1983; 22: 2420–2427
- Hobbiger F. Inhibition of cholinesterases by irreversible inhibitors in vitro and in vivo. British Journal of Pharmacology & Chemotherapy 1951; 6: 21–30
- Kamel F, Engel LS, Gladen BC, Hoppin JA, Alavanja MCR, Sandler DP. Neurologic symptoms in licensed private pesticide applicators in the agricultural health study. Environmental Health Perspectives 2005; 113: 877–882
- Katz J, Rosenfeld S, Sellers AL. Sites of plasma albumin catabolism in rat. American Journal of Physiology 1961; 200: 1301–1306
- Kragh-Hansen U, Chuang VTG, Otagiri M. Practical aspects of the ligand-binding and enzymatic properties of human serum albumin. Biological & Pharmaceutical Bulletin 2002; 25: 695–704
- Li B, Schopfer LM, Hinrichs SH, Masson P, Lockridge O. Matrix-assisted laser desorption/ionization time-of-flight mass spectrometry assay for organophosphorus toxicants bound to human albumin at Tyr411. Annals of Biochemistry 2007; 361: 263–272
- Lotti M. Low-level exposures to organophosphorus esters and peripheral nerve function. Muscle Nerve 2002; 25: 492–504
- Lotti M, Moretto A. Organophosphate-induced delayed polyneuropathy. Toxicology Review 2005; 24: 37–49
- Maliwal BP, Guthrie FE. Interaction of insecticides with human serum albumin. Molecular Pharmacology 1981; 20: 138–144
- Mason HJ. The recovery of plasma cholinesterase and erythrocyte acetylcholinesterase activity in workers after over-exposure to dichlorvos. Occupational Medicine 2000; 50: 343–347
- Mason HJ, Sams C, Stevenson AJ, Rawbone R. Rates of spontaneous reactivation and aging of acetylcholinesterase in human erythrocytes after inhibition by organophosphorus pesticides. Human & Experimental Toxicology 2000; 19: 511–516
- Means GE, Wu HL. Reactive tyrosine residue of human serum albumin: characterization of its reaction with diisopropylfluorophosphate. Archives of Biochemistry Biophysics 1979; 194: 526–530
- Mourik J, Jong LPAD. Binding of organophosphates parathion and paraoxon to bovine and human serum albumin. Archives of Toxicology 1978; 41: 43–48
- Munkner T, Videbaek A, Matzke J. Cholinesterase activity of human plasma after intramuscular diisopropylfluorophosphonate (DFP). Acta Pharmacologica et Toxicologica 1961; 18: 170–174
- Murachi T. A general reaction of diisopropylphosphorofluoridate with proteins without direct effect on enzymic activities. Biochimica et Biophysica Acta 1963; 71: 239–240
- Nielsen H, KraghHansen U, Minchiotti L, Galliano M, Brennan SO, Tarnoky AL, Franco MHLP, Salzano FM, Sugita O. Effect of genetic variation on the fatty acid-binding properties of human serum albumin and proalbumin. Biochimica et Biophysica Acta 1997; 1342: 191–204
- Ortigozaferado J, Richter RJ, Hornung SK, Motulsky AG, Furlong CE. Paraoxon hydrolysis in human serum mediated by a genetically variable arylesterase and albumin. American Journal of Human Genetics 1984; 36: 295–305
- Ostergaard D, VibyMogensen J, Hanel HK, Skovgaard LT. Half-life of plasma cholinesterase. Acta Anaesthesiologica Scandinavica 1988; 32: 266–269
- Peeples ES, Schopfer LM, Duysen EG, Spaulding R, Voelker T, Thompson CM, Lockridge O. Albumin, a new biomarker of organophosphorus toxicant exposure, identified by mass spectrometry. Toxicological Sciences 2005; 83: 303–312
- Peters T, Jr. All about albumin: biochemistry, genetics, and medical applications. Academic Press, London 1996
- Petitpas I, Grune T, Bhattacharya AA, Curry S. Crystal structures of human serum albumin complexed with monounsaturated and polyunsaturated fatty acids. Journal of Molecular Biology 2001; 314: 955–960
- Polhuijs M, Langenberg JP, Benschop HP. New method for retrospective detection of exposure to organophosphorus anticholinesterases: application to alleged sarin victims of Japanese terrorists. Toxicology & Applied Pharmacology 1997; 146: 156–161
- Pope CN. Organophosphorus pesticides: do they all have the same mechanism of toxicity?. Journal of Toxicology & Environmental Health B Critical Review 1999; 2: 161–181
- Qiao D, Seidler FJ, Slotkin TA. Developmental neurotoxicity of chlorpyrifos modeled in vitro: comparative effects of metabolites and other cholinesterase inhibitors on DNA synthesis in PC12 and C6 cells. Environmental Health Perspectives 2001; 109: 909–913
- Quistad GB, Klintenberg R, Casida JE. Blood acylpeptide hydrolase activity is a sensitive marker for exposure to some organophosphate toxicants. Toxicological Sciences 2005; 86: 291–299
- Raveh L, Ashani Y, Levy D, Delahoz D, Wolfe AD, Doctor BP. Acetylcholinesterase prophylaxis against organophosphate poisoning – quantitative correlation between protection and blood-enzyme level in mice. Biochemical Pharmacology 1989; 38: 529–534
- Ray DE, Richards PG. The potential for toxic effects of chronic, low-dose exposure to organophosphates. Toxicology Letters 2001; 120: 343–351
- Richards PG, Johnson MK, Ray DE. Identification of acylpeptide hydrolase as a sensitive site for reaction with organophosphorus compounds and a potential target for cognitive enhancing drugs. Molecular Pharmacology 2000; 58: 577–583
- Richards P, Lees J. Functional proteomics using microchannel plate detectors. Proteomics 2002; 2: 256–261
- Sanger F. Amino acid sequences in active centres of certain enzymes. Proceedings of the Chemistry Society London 1963; 5: 76–83
- Schopfer LM, Champion MM, Tamblyn N, Thompson CM, Lockridge O. Characteristic mass spectral fragments of the organophosphorus agent FP-biotin and FP-biotinylated peptides from trypsin and bovine albumin (Tyr410). Annals of Biochemistry 2005; 345: 122–132
- Sogorb MA, Diaz-Alejo N, Escudero MA, Vilanova E. Phosphotriesterase activity identified in purified serum albumins. Archives of Toxicology 1998; 72: 219–226
- Sogorb MA, Alvarez-Escalante C, Carrera V, Vilanova E. An in vitro approach for demonstrating the critical role of serum albumin in the detoxication of the carbamate carbaryl at in vivo toxicologically relevant concentrations. Archives of Toxicology 2007; 81: 113–119
- Sultatos LG, Basker KM, Shao M, Murphy SD. The interaction of the phosphorothioate insecticides chlorpyrifos and parathion and their oxygen analogs with bovine serum albumin. Molecular Pharmacology 1984; 26: 99–104
- Troester MA, Lindstrom AB, Waidyanatha S, Kupper LL, Rappaport SM. Stability of hemoglobin and albumin adducts of naphthalene oxide, 1,2-naphthoquinone, and 1,4-naphthoquinone. Toxicological Sciences 2002; 68: 314–321
- Vandekar M, Heath DF. Reactivation of cholinesterase after inhibition in vivo by some dimethyl phosphate esters. Biochemical Journal 1957; 67: 202–208
- van der Schans MJ, Polhuijs M, van Dijk C, Degenhardt CEAM, Pleijsier K, Langenberg JP, Benschop HP. Retrospective detection of exposure to nerve agents: analysis of phosphofluoridates originating from fluoride-induced reactivation of phosphylated BuChE. Archives of Toxicology 2004; 78: 508–524
- Watanabe H, Tanase S, Nakajou K, Maruyama T, Kragh-Hansen U, Otagiri M. Role of Arg-410 and Tyr-411 in human serum albumin for ligand binding and esterase-like activity. Biochemical Journal 2000; 349: 813–819
- Williams NH, Harrison JM, Read RW, Black RM. Phosphylated tyrosine in albumin as a biomarker of exposure to organophosphorus nerve agents. Archives of Toxicology 2007; 81: 627–639
- Worek F, Diepold C, Eyer P. Dimethylphosphoryl-inhibited human cholinesterases: inhibition, reactivation, and aging kinetics. Archives of Toxicology 1999; 73: 7–14
- Zhang Q, Wang L, Ahn KC, Sun Q, Hu B, Wang J, Liu F. Hapten heterology for a specific and sensitive indirect enzyme-linked immunosorbent assay for organophosphorus insecticide fenthion. Analytica Chimica Acta 2007; 596: 303–311