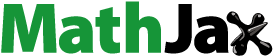
Abstract
Context: Various parts of Ziziphus abyssinica Hochst ex. A. Rich (Rhamnaceae) have been used in Ghanaian and African traditional medicine as an analgesic. However, there are little scientific data to support the anti-nociceptive effects of the hydro-ethanolic leaf extract of Ziziphus abyssinica (EthE) as well as the possible mechanisms involved in its anti-nociceptive effects.
Purpose: To predict possible nociceptive pathways involved in the anti-nociceptive effects of EthE.
Materials and methods: The effect of EthE (30, 100 and 300 mg/kg) on intraplantar injection of pain mediators such as interleukin-1β, tumour necrosis factor-α, prostaglandin E2 and bradykinin was evaluated in male Sprague Dawley rats using Randall–Selitto test for 5 h. The effect of specific antagonists to the opioidergic, adenosinergic, ATP-sensitive K+ channels, nitric oxide, serotonergic, muscarinic, adrenergic and voltage-gated calcium channel on the anti-nociceptive effect of EthE (100 mg/kg) was evaluated using the formalin test in male imprinting control region (ICR) mice for 1 h.
Results: Pretreatment of the rats with EthE significantly reversed the hypernociception induced by intraplantar injection of TNF-α (F4,120 = 10.86, p < 0.0001), IL-1β (F4,120 = 14.71, p < 0.0001), bradykinin (F4,80 = 12.52, p < 0.0001) and prostaglandin E2 (F5,144 = 6.165, p = 0.0001). The anti-nociceptive effect exhibited by EthE in the formalin test was reversed by systemic administration of NG-l-nitro-arginine methyl ester, naloxone, theophylline and glibenclamide.
Conclusions: EthE inhibits hypernociception induced by TNF-α, IL-1β, bradykinin and prostaglandin E2. EthE exhibited anti-nociceptive effects possibly mediated through opioidergic, adenosinergic, ATP-sensitive potassium channels and nitric oxide cyclic GMP pathways.
Introduction
Pain is a personal and subjective experience that involves emotional, behavioural and sensory elements associated with actual or potential tissue damage (Merskey and Bugduk 1994). It is a direct or indirect consequence of several diseases and is the commonest reason for hospital visits (American Pain Society 2000). In both developing and developed countries, patients with moderate to severe pain are often under-treated because opioids, which are the mainstay of pain relief in such cases, are mostly inaccessible. This is a result of the fact that opioids are categorized as controlled substances and therefore are subjected to stringent control (Kumar Citation2007).
Inflammatory mediators such as prostaglandins and bradykinins are marked sensitizers of nociceptors. These mediators facilitate the electrical activity of the neuronal membrane by acting on neuronal receptors directly resulting in the activation of several molecular mechanisms which subsequently causes hypernociception (Linley et al. Citation2010; Schaible et al. Citation2011).
Cytokines are important mediators of peripheral sensitization. Intradermal administration of cytokines such as keratinocyte-derived chemokine (KC), TNF-α, interleukins IL-1β, IL-8, IL-12, IL-15 and IL-18 have produced intense and sustained mechanical sensitization and hypernociception in rodents (Stein et al. Citation2009). It has been proposed that TNF-α can be stimulated by irritants such as carrageenan, lipopolysaccharide or the antigen itself which, in turn, induces IL-1β and IL-6, thus activating the synthesis of cyclooxygenase products (PGE2). Again, TNF-α can induce another cytokine, IL-8, thus stimulating the local production of sympathetic amines which subsequently produces hypernociception. Also, endothelin-1 can be activated by IL-18 and IL-12 resulting in hypernociception (Ferreira et al. Citation1993).
Understanding the exact mechanism of medicinal substances and blocking or antagonizing these pathways have been the focus of researchers in recent times and have resulted in the development of novel analgesics. Compounds such as TRPV1 antagonists (Willis Citation2009), nerve growth factor (NGF) antagonists (Watson et al. Citation2008; Cattaneo Citation2010), selective Na channel blockers (Jarvis et al. Citation2007), anti-TNF-α and interleukin-1 monoclonal antibodies (Furst et al. Citation2003; Vale et al. Citation2004), and bradykinin receptor antagonists (Rodger Citation2009) have been targeted in the search for novel analgesics to augment or replace the already available analgesics.
Plants also constitute a large source of novel phytocompounds that might lead to the discovery of new pharmaceutical agents (Shah and Alagawadi 2011). About 25% of drugs prescribed worldwide are derived from plants and their use as medicines is still widespread (Wachtel-Galor and Benzie Citation2011). One such plant is Ziziphus abyssinica Hochst Ex A. Rich (Rhamnaceae), commonly called ‘catch thorn’ in English and ‘Jujubier sauvage’ in French; the Hausa’s call it ‘magariya’, whereas in Ghana it is called ‘larukluror’ (Sisaala) (Burkill 1985; Orwa et al. Citation2009). Extracts from various parts of the plant have exhibited antioxidant, antibacterial and antifungal activities (Gundidza and Sibanda 1991; Nyaberi et al. Citation2010; Wagate et al. Citation2010). Molluscicidal (Kela et al. Citation1989) and antiplasmodial (Muthaura et al. Citation2015) activities of the plant have been reported. Root extracts have been reported to possess anti-ulcerogenic (Ugwah et al. Citation2013) and antidiarrheal (Ugwah-Oguejiofor et al. Citation2013) properties. Our earlier research validated the analgesic effect of the hydro-ethanolic leaf extract of the plant in murine models (Boakye-Gyasi et al. Citation2017). Qualitative phytochemical investigations have revealed that the aqueous and methanol fruit extracts of Z. abyssinica contain saponins, tannins, sterols and steroids, alkaloids, flavonoids and reducing compounds (Nyaberi et al. Citation2010). Also the presence of carbohydrates, alkaloids, saponins, tannins, glycosides, anthraquinones and steroids were detected in Z. abyssinica aqueous root extract (Ugwah et al. Citation2013). Hydro-ethanolic leaf extract of the plant has been reported to contain tannins, phenols, alkaloids, triterpenes, flavonoids and phytosterols (Boakye-Gyasi et al. Citation2017).
Since there is no report on the possible mechanisms mediating the anti-nociceptive effects of the hydro-ethanolic leaf extract of Ziziphus abyssinica, this study seeks to determine the possible involvement of some important inflammatory mediators, cytokines, ion channels, receptors and ligands in the anti-nociceptive effect of the extract.
Method and materials
Plant collection
Fresh leaves of Ziziphus abyssinica were collected from Ejura (7°23′00.16″N, 1°22′00.00″W) in the Ejura-Sekyedumase Municipal of Ashanti Region in the month of October 2015. It was authenticated by Mr. Clifford Asare of the Department of Herbal Medicine, Faculty of Pharmacy and Pharmaceutical Sciences (FPPS), Kwame Nkrumah University of Science and Technology (KNUST). A voucher specimen (KNUST/HM/2016/L003) was deposited at the Department of Herbal Medicine’s herbarium.
Plant extraction
About 1 kg of fresh matured leaves of Ziziphus abyssinica was air-dried for 14 days in a room. With the aid of a hammer mill, it was pulverized into fine powder. The powdered leaves (600 g) were extracted with 4 L of 70% v/v ethanol for 48-h period using a Soxhlet apparatus (Aldrich® Soxhlet Extraction Apparatus, Z556203, St. Louis, MO). The extract obtained was labelled as EthE (hydro-ethanolic extract) and subsequently concentrated using a rotary evaporator (Rotavapor R-215 model, BÜCHI Labortechnik AG, Flawil, Switzerland) under reduced pressure and temperature (70 °C). This was further dried on a water bath and then preserved in a desiccator containing activated silica until it was ready for use. The yield obtained was 10.8% w/w.
Animals
Male imprinting control region (ICR) mice (20–25 g) and Sprague Dawley rats (170–250 g) were bought from Noguchi Memorial Institute for Medical Research, University of Ghana, Legon, Ghana. They were kept in stainless cages (34 cm × 47 cm × 18 cm) in groups of five at the animal house facility of Faculty of Pharmacy and Pharmaceutical Sciences (FPPS), KNUST, Kumasi. The animals were given normal commercial diet obtained from Agricare Limited, Kumasi, Ghana, and water ad libitum. They were kept under normal laboratory conditions with regard to room temperature and humidity. All the techniques and protocols used in the study were done in accordance with established public health guidelines in ‘Guide for Care and Use of Laboratory Animals’ (Garber et al. Citation2011). Also, all protocols used in the study were approved by the Department of Pharmacology, FPPS, KNUST Ethics Committee.
Drugs and chemicals
The following chemicals and drugs were used in the study: formalin, theophylline, carrageenan and acetic acid (British Drug House, Poole, England); granisetron hydrochloride (Corepharma LLC, Middlesex, NJ); glibenclamide (Daonil®, Sanofi-Aventis, Guildford, UK); yohimbine hydrochloride (Procomil®, Walter Ritter GmbH & Co. KG, Hamburg, Germany); atropine sulphate, naloxone hydrochloride, morphine sulphate (Duopharma (M) Sdn Bhd, Malaysia); nifedipine and diclofenac sodium (Denk Pharma, Munich, Germany); and l-glutamic acid, NG-l-nitro-arginine methyl ester (L-NAME), prostaglandin E2 (PGE2), bradykinin acetate salt, murine recombinant interleukin-1β (IL-1β) and tumour necrosis factor-alpha (TNF-α) (Sigma-Aldrich Inc., St. Louis, MO).
Tumour necrosis factor-alpha (TNF-α)-induced hypernociception
Assessment of mechanical hypernociception induced by TNF-α after pretreatment of rats with EthE or morphine was performed as previously described by Vale et al. (Citation2004). Five groups of rats (n = 5) received pretreatment with either vehicle (10 mL/kg, p.o.), EthE (30, 100 and 300 mg/kg p.o.) for 1 h or morphine (3 mg/kg, i.p.) for 30 min before intraplantar injection of TNF-α (2.5 pg/paw; 20 μL) into the right hind paw. Hypernociception was measured in the injected paws at 1, 2, 3, 4 and 5 h post-TNF-α injection using an analgesimeter (Model No. 15776, Ugo Basile, Comerio, Varese, Italy) as described previously (Randall and Selitto 1957; Villetti et al. Citation2003). The rats were trained at three different times before the day of testing and it involved gradually applying pressure to their right hind paws. The applied pressure (grams) able to elicit paw withdrawal was recorded as paw withdrawal threshold (PWT). A cut-off threshold of 250 g was set in order not to cause any injury to the paws. Baseline thresholds were taken for each animal before they were given TNF-α.
Percentage maximum possible effect was determined using the formula below:
This same procedure was used to measure hypernociception in the IL-1β, PGE2 and bradykinin-induced hypernociceptions below.
Interleukin-1-beta (IL-1β)-induced hypernociception
Assessment of mechanical hypernociception induced by IL-1β after pretreatment of rats with EthE or morphine was performed as previously described by Vale et al. (Citation2004). Five groups of male rats (n = 5) received pretreatment with either vehicle (10 mL/kg, p.o.), EthE (30, 100 and 300 mg/kg, p.o.) for 1 h or morphine (3 mg/kg, i.p.) for 30 min before intraplantar injection of IL-1β (1 pg/paw; 20 μL) into the right hind paws.
Prostaglandin E2 (PGE2)-induced hypernociception
The effect of pretreatment of rats with EthE on prostaglandin E2-induced hypernociception was evaluated as earlier described (Vale et al. Citation2004; Woode et al. Citation2012). Six groups of rats (n = 5) received pretreatment with either vehicle (10 mL/kg, p.o.) or EthE (30–300 mg/kg, p.o.) for 1 h and morphine (3 mg/kg, i.p.) or diclofenac (10 mg/kg, i.p.) for 30 min before intraplantar injection of PGE2 (100 ng/paw; 20 μL) into their right hind paws.
Bradykinin-induced hypernociception
To evaluate the effect of EthE pretreatment on mechanical hypernociception induced by bradykinin in rats, a previously described method was used (Vale et al. Citation2004; Woode et al. Citation2012). Five groups of rats (n = 5) were pretreated with either EthE (30, 100 and 300 mg/kg, p.o., 1 h) or morphine (3 mg/kg, i.p., 30 min) before intraplantar injection of bradykinin (500 ng/paw; 20 μL) into their right hind paws. The control group received vehicle (10 mL/kg, p.o., 1 h). Rats were pretreated with captopril (5 mg/kg, s.c.) 1 h before the experiment to avoid break down of bradykinin by angiotensin converting enzyme.
Further investigation into the possible mechanism of EthE anti-nociceptive effect
Formalin test (Tjølsen et al. Citation1992; Ellis et al. Citation2008) was used to further assess the possible involvement of the opioidergic, adenosinergic, ATP-sensitive K+ channels, nitric oxide, serotonergic, muscarinic, adrenergic and voltage-gated calcium channel pathways in the observed anti-nociceptive effect of the extract. Doses of drugs were selected based on preliminary experiments in our laboratories and previous studies (Woode et al. Citation2012).
To investigate the roles played by these nociceptive pathways, nineteen groups of mice (n = 5) were pretreated orally with naloxone (2 mg/kg, i.p., a nonselective opioid receptor antagonist), theophylline (5 mg/kg, p.o., a nonselective adenosine receptor antagonist), glibenclamide (8 mg/kg, p.o., an ATP-sensitive K+ channel inhibitor), L-NAME, (10 mg/kg, i.p., a nitric oxide synthase inhibitor), granisetron (2 mg/kg, p.o., a 5HT3 receptor antagonist), atropine (3 mg/kg, i.p., nonselective muscarinic antagonist), yohimbine (3 mg/kg, p.o., an α2 receptor antagonist) and nifedipine (10 mg/kg, p.o., L-type voltage-gated calcium channel blocker). After 60 min (p.o.) or 30 min (i.p.) pretreatment with various antagonists, mice were either given oral administration of 100 mg/kg EthE or intraperitoneal injection of 3 mg/kg morphine. Negative control group received only vehicle (10 mL/kg, p.o.), whereas EthE- and morphine-treated controls received either 100 mg/kg EthE or 3 mg/kg morphine only. After one-h EthE or 30-min morphine treatments, pain was induced with 10 μL of 5% formalin in all the groups and nociceptive score was measured for 1 h. To do this, the mice were instantly transferred into transparent testing perspex chambers (15 cm ×15 cm ×15 cm). A mirror placed at 45° to the floor level allowed complete view of the animals in the camcorder (Sony Handycam, model HDRCX675/B, Tokyo, Japan) which was used to capture the nociceptive behaviours of the mice following formalin injection. This was recorded for 60 min and later tracked using a JWatcher™ software version 1.0 developed by University of California, Los Angeles, CA, USA and Macquarie University, Sydney, Australia (http://www.jwatcher.ucla.edu/). A nociceptive score for every 5 min time bloc was obtained by measuring the duration and frequency of licking/biting of injected paws and the mean nociceptive score for each time bloc per 5 min determined as the product of the duration and frequency of licking/biting. The results obtained were considered as early/neurogenic phase (0–10 min) and late/inflammatory phase (10–60 min) from which time-course curves were plotted and the areas under the curves (AUCs) for each phase and each treatment determined and plotted.
Acute toxicity study
ICR mice (25–30 g) were randomly selected and divided into seven groups of five mice in each group. They were fasted overnight and given EthE (30, 100, 300, 1000, 3000 and 5000 mg/kg, p.o.). The control group received 10 mL/kg p.o. of normal saline. The mice were observed at time 0, 30, 60, 120 and 180 min after drug administration for any toxic signs or death. They were also observed at 24 h and daily for up to 7 days to detect any possible delayed deaths.
Statistical analysis
A sample size of five rats or mice per group was used in all in vivo tests. Mean ± standard error of mean (SEM) was used in presenting all data. All time-course curves in the study were analyzed using two-way analysis of variance (ANOVA) with Bonferroni’s post hoc test. One-way ANOVA with Newman–Keuls post hoc test was used to determine differences between treatments groups (areas under the curves). The equation below was used to calculate the percentage inhibition for each treatment:
GraphPad® Prism version 7.0 (GraphPad Software, San Diego, CA) for Windows was used to perform all statistical analysis with p < 0.05 considered statistically significant for all tests.
Results
TNF-α-induced hypernociception
The results presented in show that EthE and morphine markedly reversed hypernociception induced by intraplantar injection of TNF-α irritant. Pretreatment of the rats with EthE (30, 100 and 300 mg/kg, p.o.) and morphine (3 mg/kg, i.p.) led to a significant decrease in the paw withdrawal latencies of rats (F4,120 = 10.86, p < 0.0001, ) and total anti-nociceptive score (F4,18 = 5.811, p = 0.005, ). The highest dose of EthE completely reversed TNF-α-induced mechanical hypernociception with an average total anti-nociceptive score of 21.62 ± 2.52, whereas morphine (3 mg/kg, i.p.) similarly reversed the hypernociception with an average total anti-nociceptive score of 23.49 ± 4.59 ().
Figure 1. Effect of pretreatment of rats with EthE (30–300 mg/kg, p.o.) and morphine (3 mg/kg, i.p.) on TNF-α-induced hypernociception. Each datum represents the mean of five animals and the error bars indicate SEM. The symbols * and † indicate significance levels compared to respective control groups: (a) represent the time-course curves ***p < 0.001, **p < 0.01 (two-way ANOVA followed by Bonferroni’s post hoc test), whereas (b) represents total anti-nociceptive effects (AUC) ††p < 0.01 and †p < 0.05 (one-way ANOVA followed by Newman–Keuls post hoc test).

Interleukin-1β-induced hypernociception
The results presented in show that intraplantar injection of IL-1β prominently decreased rats’ paw withdrawal thresholds in the Randall–Selitto test compared to baseline readings and this has been described as a state of hypernociception which was maintained throughout the test in the vehicle-treated rats. Pretreatment of the animals with either EthE (30, 100 or 300 mg/kg, p.o.) or morphine (3 mg/kg, i.p.) significantly reversed the hypernociception by increasing paw withdrawal thresholds (F4,120 = 14.71, p < 0.0001, ) and total anti-nociceptive score (F4,20 = 5.96, p = 0.0025, ). From the results presented in , EthE (300 mg/kg, p.o.) completely reversed IL-1β-induced hypernociception with a mean total anti-nociceptive score of 40.99 ± 18.27 even though it was less potent than morphine (3 mg/kg, i.p.) which had a mean total anti-nociceptive score of 65.33 ± 26.91 ().
Figure 2. Effect of pretreatment of rats with EthE (30–300 mg/kg, p.o.) and morphine (3 mg/kg, i.p.) on IL-1β-induced hypernociception. Each datum represents the mean of five animals and the error bars indicate SEM. The symbols * and † indicate significance levels compared to respective control groups: (a) represents the time-course curves **p < 0.01, *p < 0.05 (two-way ANOVA followed by Bonferroni’s post hoc test), whereas (b) represents total anti-nociceptive effects (AUC) ††p < 0.01 and †p < 0.05 (one-way ANOVA followed by Newman–Keuls post hoc test).

Bradykinin-induced hypernociception
Mechanical pressure applied to the rats’ right hind paws after intraplantar injection of bradykinin resulted in an increase in paw withdrawal reflexes which can be described as a state of hypernociception in the animals. Pretreatment of the animals with either EthE (30, 100 or 300 mg/kg, p.o.) or morphine (3 mg/kg, i.p.) led to a significant increase in paw withdrawal latencies (F4,80 = 12.52, p < 0.0001, ) and total anti-nociceptive score (F4,20 = 8.353, p = 0.0004, ). The highest dose of EthE produced a mean total anti-nociceptive score of 35.45 ± 5.55, whereas morphine (3 mg/kg, i.p.) produced a total anti-nociceptive score of 29.0 ± 6.96 ().
Figure 3. Effect of pretreatment of rats with EthE (30–300 mg/kg, p.o.) and morphine (3 mg/kg, i.p.) on bradykinin-induced hypernociception. Each datum represents the mean of five animals and the error bars indicate SEM. The symbols * and † indicate significance levels compared to respective control groups: (a) represents the time-course curves ***p < 0.001, **p < 0.01, *p < 0.05 (two-way ANOVA followed by Bonferroni’s post hoc test), whereas (b) represents total anti-nociceptive effects (AUC) †††p < 0.001 and ††p < 0.01 (one-way ANOVA followed by Newman–Keuls post hoc test).

Prostaglandin E2-induced hypernociception
Intraplantar administration of prostaglandin E2 irritant (100 ng/paw, 20 μL) resulted in a decrease in paw withdrawal threshold upon the application of mechanical pressure to the right hind paws of the rats and this can be described as a state of hypernociception. Pretreatment of the rats with EthE (30, 100 and 300 mg/kg, p.o.), morphine (3 mg/kg, i.p.) and diclofenac (10 mg/kg, i.p.) resulted in a significant increase in paw withdrawal thresholds (F5,144 = 6.165, p = 0.0001, ) and total anti-nociceptive scores (F5,24 = 5.811, p = 0.0012, ). The highest dose of EthE completely reversed the hypernociception induced by PGE2 with a mean total anti-nociceptive score of 17.31 ± 5.84. Morphine (3 mg/kg, i.p.) and diclofenac (10 mg/kg, i.p.) similarly reversed the hypernociception with mean total anti-nociceptive scores of 18.98 ± 8.40 and 25.9 ± 6.66, respectively ().
Figure 4. Effect of pretreatment of rats with EthE (30–300 mg/kg, p.o.), morphine (3 mg/kg, i.p.) and diclofenac (10 mg/kg, i.p.) on PGE2-induced hypernociception. Each datum represents the mean of five animals and the error bars indicate SEM. The symbols * and † indicate significance levels compared to respective control groups: (a) represents the time-course curves **p < 0.01, *p < 0.05 (two-way ANOVA followed by Bonferroni’s post hoc test), whereas (b) represents total anti-nociceptive effects (AUC) ††p < 0.01 (one-way ANOVA followed by Newman–Keuls post hoc test).

Further assessment of possible mechanism of action of EthE
Results presented in and show the effect of pretreatment of mice with yohimbine (3 mg/kg, p.o.), nifedipine (10 mg/kg, p.o.), atropine (5 mg/kg, i.p.), naloxone (2 mg/kg, i.p.), granisetron (2 mg/kg, p.o.), L-NAME (10 mg/kg, i.p.), glibenclamide (8 mg/kg, p.o.) and theophylline (10 mg/kg, i.p.) on the anti-nociceptive effects of EthE. Both phase 1 and phase 2 anti-nociceptive effects of EthE (100 mg/kg, p.o.) were significantly reversed by pretreatment of mice with naloxone and L-NAME. Pretreatment of mice with glibenclamide and theophylline significantly reversed the anti-nociceptive effect of EthE in only the second phase of formalin test. Yohimbine, nifedipine, atropine and granisetron could not significantly reverse EthE anti-nociception in both phases of formalin test ( and ).
Figure 5. Effect of pretreatment of mice with (a) yohimbine (3 mg/kg, p.o.), (b) nifedipine (10 mg/kg, p.o.), (c) atropine (5 mg/kg, i.p.), (d) naloxone (2 mg/kg, i.p.), (e) granisetron (2 mg/kg, p.o.), (f) L-NAME (10 mg/kg, i.p.), (g) glibenclamide (8 mg/kg, p.o.) and (h) theophylline (10 mg/kg, i.p.) on the nociceptive scores of EthE (100 mg/kg, p.o.) on the time-course curves of formalin-induced nociceptive test. Each point represents the mean of five animals and the error bars indicate SEM. ***p < 0.001, **p < 0.01 and *p < 0.05 compared to the control group at same time points (two-way ANOVA followed by Bonferroni’s post hoc test).

Figure 6. Effect of pretreatment of mice with yohimbine (3 mg/kg, p.o.), nifedipine (10 mg/kg, p.o.), atropine (5 mg/kg, i.p.), naloxone (2 mg/kg i.p.), granisetron (2 mg/kg, p.o.), L-NAME (10 mg/kg, i.p), glibenclamide (8 mg/kg, p.o.) and theophylline (10 mg/kg, i.p.) on the total nociceptive score of EthE (100 mg/kg, p.o.) in phase 1 and phase 2 of formalin-induced nociception. Each column represents the mean of five animals and the error bars indicate SEM. ***p < 0.001 compared to control group and †††p < 0.001, ††p < 0.01 and †p < 0.05 compared to EthE-alone-treated group (one-way ANOVA followed by Newman–Keuls post hoc test).

Also, results presented in and show the effect of pretreatment of mice with yohimbine (3 mg/kg, p.o.), nifedipine (10 mg/kg, p.o.), atropine (5 mg/kg, i.p.), naloxone (2 mg/kg, i.p.), granisetron (2 mg/kg, p.o.), L-NAME (10 mg/kg, i.p.), glibenclamide (8 mg/kg, p.o.) and theophylline (10 mg/kg, i.p.) on the anti-nociceptive effect induced by morphine (3 mg/kg, i.p.). Intraperitoneal administration of morphine (3 mg/kg, i.p.) resulted in a significant decrease in total nociception induced by intraplantar injection of 5% formalin (p < 0.001). The anti-nociceptive effect of morphine (3 mg/kg, i.p.) was markedly reversed by pretreatment of mice with all the antagonists used in both phases except glibenclamide. Pretreatment of mice with glibenclamide (8 mg/kg, p.o.) 30 min before morphine (3 mg/kg, i.p.) significantly (p < 0.05) reversed the anti-nociceptive effect of the latter in only the inflammatory phase but not the neurogenic phase ( and ).
Figure 7. Effect of pretreatment of mice with (a) yohimbine (3 mg/kg, p.o.), (b) nifedipine (10 mg/kg, p.o.), (c) atropine (5 mg/kg, i.p.), (d) naloxone (2 mg/kg, i.p.), (e) granisetron (2 mg/kg, p.o.), (f) L-NAME (10 mg/kg, i.p.), (g) glibenclamide (8 mg/kg, p.o.) and (h) theophylline (10 mg/kg i.p.) on the total nociceptive score of morphine (3 mg/kg, i.p.) on the time-course curves of formalin-induced nociceptive test. Each point represents the mean of five animals and the error bars indicate SEM. ***p < 0.001, **p < 0.01 and *p < 0.05 compared the control group at same time points (two-way ANOVA followed by Bonferroni’s post hoc test).

Figure 8. Effect of pretreatment of mice with yohimbine (3 mg/kg, p.o.), nifedipine (10 mg/kg, p.o.), atropine (5 mg/kg, i.p.), naloxone (2 mg/kg i.p.), granisetron (2 mg/kg, p.o.), L-NAME (10 mg/kg, i.p), glibenclamide (8 mg/kg, p.o.) and theophylline (10 mg/kg, i.p.) on the total nociceptive scores of morphine (3 mg/kg, i.p.) in phase 1 and phase 2 of formalin-induced nociception. Each column represents the mean of five animals and the error bars indicate SEM. ***p < 0.001 compared to control group and †††p < 0.001, ††p < 0.01 and †p ≤ 0.05 compared to morphine-alone-treated group (one-way ANOVA followed by Newman–Keuls post hoc test).

Acute toxicity study
There was no lethality recorded during the 7-day study period at doses up to 5000 mg/kg. Treated mice did not exhibit any toxic signs in terms of their behavioural, neurological and autonomic activities as compared to control.
Discussion
Intraplantar injection of TNF-α antigen is known to stimulate IL-1β production, thus inducing the production of cyclooxygenase products such as prostaglandin E2 which subsequently causes hypernociception (Verri et al. Citation2007). A common feature of the inflammatory response produced by these pro-inflammatory cytokines (TNF-α and IL-1β) when injected intraplantarly is increased pain sensitivity which occurs as a result of their direct action on their respective targets, thus decreasing pain thresholds resulting in hypernociception (Jin and Gereau 2006; Binshtok et al. Citation2008). EthE significantly reversed hypernociception induced by intraplantar injection of TNF-α and IL-1β, suggesting a possible blockade of their targets or inhibitory effect on their release peripherally and/or centrally.
Similarly, the hypernociception induced by prostaglandin E2 and bradykinin, marked sensitizers of nociceptors, was both reversed significantly and dose dependently by the extract EthE. By binding to G protein-coupled receptors, prostaglandin E2 increases cAMP which subsequently activates PKA in cells. This pathway enhances excitability of neurons by sensitizing ion channels in membranes such as TRPV1 receptors and Na+ channels (Schaible et al. Citation2011). On the other hand, the mechanical hypernociception induced by bradykinin involves an indirect activation of PKA and a direct activation of B2 receptor-mediated phospholipase C (PLC) which in turn leads to the production of PKC resulting in the sensitization of sensory ion channels which subsequently leads to hypernociception (Ferreira et al. Citation2004; Linley et al. Citation2010). EthE might have acted possibly by inhibiting the release or interfering with some of the pathways of these mediators, thus reversing the hypernociception.
In an attempt to further identify other pathways possibly involved in the pain-relieving effect of extract, mice were treated with either EthE or morphine in the presence of the following antagonists: yohimbine, nifedipine, atropine, naloxone, granisetron, L-NAME, glibenclamide and theophylline. The ability of the antagonists to reverse the pain-relieving activity of the extract or morphine was then assessed in the formalin-induced nociception test.
The anti-nociceptive effect of EthE was markedly overturned in both phases of the formalin test following pretreatment of animals with L-NAME, a nitric oxide (NO) synthase inhibitor. The findings implicate the involvement of the NO cGMP mechanism. NO is a constantly synthesized soluble gas from L-arginine amino acid in endothelial cells by the nitric oxide synthase (NOS) enzyme (Mayer and Hemmens 1997). Nitric oxide is known to play a complex part in transmission of nociceptive signals peripherally and centrally (Cury et al. Citation2011; Galdino et al. Citation2015). Nitric oxide is known to be involved in the inhibition of neurons that are spontaneously activated in response to pain and particularly diminishing the activity of pain mediators such as substance P released in spinal cord (Garry et al. Citation1994; Schmid and Pehl 1996). The marked reversal of EthE anti-nociceptive activity by NOS antagonist, L-NAME, is an indication of a possible involvement of NO cGMP pathway in the extract’s mode of anti-nociception action.
Furthermore, pretreatment with naloxone, a nonselective opioid antagonist, also blocked the anti-nociceptive effects of EthE which might implicate the involvement of opioid receptors and/or endogenous opioids in the anti-nociceptive effects of the extract (Bovill Citation1997; Kiran and Sinha 2015).
Adenosine is an important endogenous modulator of neurotransmission and it participates in modulating several biological activities including nociception. Such effects are mediated through the stimulation of its G protein-coupled receptors A1, A2a, A2b and A3 (Fredholm et al. Citation2001). Whereas the activation of A2 and A3 facilitates nociception (Sawynok Citation1998), the trigger of A1 spinal receptors further slows responses of C fibre-mediated pain transmission in the neurons of the dorsal horn which subsequently causes anti-nociception. Again, the anti-nociceptive effect of A1 receptor agonist is also mediated through adenylate cyclase inhibition and a sealing of ATP-sensitive K+ Channels. From this study, the second phase of anti-nociceptive activity of EthE was significantly reversed by the adenosine antagonist, theophylline. This effect may be due to a surge in endogenous adenosine release by the extract and/or the extract’s ability to activate A1 receptors. The possible involvement of ATP potassium channel in the anti-nociceptive effect of EthE was further highlighted through pretreatment of mice with glibenclamide (an ATP-sensitive K+ channel antagonist) before they were given EthE. The results indicated that only the late phase was significantly reversed by the extract indicating the involvement of ATP-dependent potassium channels.
Though there is ample evidence that indicates the involvement of muscarinic, adrenergic, calcium channels and serotonergic pathways in nociception, the results obtained revealed that their respective antagonists could not significantly reverse the anti-nociceptive effect of the extract. Results from the acute toxicity study did not show any toxic effect that could be attributed to drug treatment. The LD50 of EthE after oral administration to mice could be estimated to be above 5000 mg/kg. This indicates that EthE is relatively nontoxic based on the recommendations of the Organization for Economic Co-operation and Development (OECD) for chemical labelling and classification of acute systemic toxicity based on oral LD50 values.
Conclusions
EthE inhibits hypernociception induced by TNF-α, IL-1β, bradykinin and prostaglandin E2. EthE exhibited anti-nociceptive effects possibly mediated through opioidergic, adenosinergic, ATP-sensitive potassium channels and nitric oxide cyclic GMP pathways.
Acknowledgements
Authors are grateful to Prof. Martins Ekor and the technical staff of the Department of Pharmacology, Faculty of Pharmacy and Pharmaceutical Sciences, KNUST.
Disclosure statement
The authors report no conflicts of interest.
Funding
This research did not receive any specific grant from funding agencies in the public, commercial or not-for-profit sectors.
References
- American Pain Society. 2000. Pain assessment and treatment in the managed care environment. Case Manager. 11:50–53.
- Binshtok AM, Wang H, Zimmermann K, Amaya F, Vardeh D, Shi L, Brenner GJ, Ji RR, Bean BP, Woolf CJ, et al. 2008 . Nociceptors are interleukin-1beta sensors. J Neurosci. 28:14062–14073.
- Bovill J. 1997. Mechanisms of actions of opioids and non-steroidal anti-inflammatory drugs. Eur J Anaesthesiol. 14:9–15.
- Boakye-Gyasi E, Henneh IT, Abotsi WKM, Ameyaw EO, Woode E. 2017. Hydro-ethanolic leaf extract of Ziziphus abyssinica Hochst Ex. A. Rich (Rhamnaceae) exhibits anti-nociceptive effects in murine models. BMC Complement Altern Med. 17:231–243.
- Burkill HM. 1985. The useful plants of west tropical Africa. Vol. 4. London: Kew, Royal Botanic Gardens.
- Cattaneo A. 2010. Tanezumab, a recombinant humanized mAb against nerve growth factor for the treatment of acute and chronic pain. Curr Opin Mol Ther. 12:94–106.
- Cury Y, Picolo G, Gutierrez VP, Ferreira SH. 2011. Pain and analgesia: the dual effect of nitric oxide in the nociceptive system. Nitric Oxide. 25:243–254.
- Ellis A, Benson N, Machin I, Corradini L. 2008. The rat formalin test: can it predict neuropathic pain treatments? Measuring Behav. 2008:324.
- Ferreira J, Da Silva GL, Calixto JB. 2004. Contribution of vanilloid receptors to the overt nociception induced by B2 kinin receptor activation in mice. Br J Pharmacol. 141:787–794.
- Ferreira S, Lorenzetti B, Poole S. 1993 . Bradykinin initiates cytokine-mediated inflammatory hyperalgesia . Br J Pharmacol. 110:1227–1231.
- Fredholm BB, Ap IJ, Jacobson KA, Klotz KN, Linden J. 2001. International Union of Pharmacology. XXV. Nomenclature and classification of adenosine receptors. Pharmacol Rev. 53:527–552.
- Furst DE, Schiff MH, Fleischmann RM, Strand V, Birbara CA, Compagnone D, Fischkoff SA, Chartash EK. 2003 . Adalimumab, a fully human anti tumor necrosis factor-alpha monoclonal antibody, and concomitant standard antirheumatic therapy for the treatment of rheumatoid arthritis: results of STAR (Safety Trial of Adalimumab in Rheumatoid Arthritis). J Rheumatol. 30:2563–2571.
- Galdino G, Duarte I, Perez A. 2015. Central release of nitric oxide mediates antinociception induced by aerobic exercise. Braz J Med Biol Res. 48:790–797.
- Garber JC, Barbee RW, Bielitzki JT, Clayton L, Donovan J, Hendriksen CM, Kohn DF, Lipman NS, Locke PA, Melcher J, et al. 2011. Guide for the care and use of laboratory animals. Washington DC: The National Academic Press.
- Garry MG, Richardson JD, Hargreaves K. 1994. Sodium nitroprusside evokes the release of immunoreactive calcitonin gene-related peptide and substance P from dorsal horn slices via nitric oxide-dependent and nitric oxide-independent mechanisms. J Neurosci. 14:4329–4337.
- Gundidza M, Sibanda M. 1991. Antimicrobial activities of Ziziphus abyssinica and Berchemia discolor. Cent Afr J Med. 37:80–83.
- Jarvis MF, Honore P, Shieh CC, Joshi S, Zhang XF, Kort M, Carroll W, Marron B, Atkinson R, Thomas J. 2007. A-803467, a potent and selective Nav1.8 sodium channel blocker, attenuates neuropathic and inflammatory pain in the rat. Proc Natl Acad Sci USA. 104:8520–8525.
- Jin X, Gereau RW. 2006 . Acute p38-mediated modulation of tetrodotoxin-resistant sodium channels in mouse sensory neurons by tumor necrosis factor-alpha. J Neurosci. 26:246–255.
- Kela SL, Ogunsusi RA, Ogbogu VC, Nwude N. 1989. Screening of some Nigerian plants for molluscicidal activity. Rev Elev Med Vet Pays Trop. 42:195–202.
- Kiran A, Sinha AN. 2015. Elucidation of analgesic effects of butorphanol compared with morphine: A prospective cohort study. J Biol Life Sci. 6:130–140.
- Kumar N. 2007. WHO normative guidelines on pain management. Geneva: World Health Organization.
- Linley JE, Rose K, Ooi L, Gamper N. 2010. Understanding inflammatory pain: ion channels contributing to acute and chronic nociception. Pflugers Arch. 459:657–669.
- Merskey H, Bugduk N. 1994. Classification of chronic pain: descriptions of chronic pain syndromes and definitions of pain terms. 2nd ed. Seattle (WA): IASP Press.
- Mayer B, Hemmens B. 1997. Biosynthesis and action of nitric oxide in mammalian cells. Trends Biochem Sci. 22:477–481.
- Muthaura C, Keriko J, Mutai C, Yenesew A, Gathirwa J, Irungu BN, Nyangacha R, Mungai GM, Derese S. 2015 . Antiplasmodial potential of traditional phytotherapy of some remedies used in treatment of malaria in Meru-Tharaka Nithi County of Kenya. J Ethnopharmacol. 175:315–323.
- Nyaberi MO, Onyango CA, Mathooko FM, Maina JM, Makobe M, Mwaura F. 2010. Evaluation of phytochemical, antioxidant and antibacterial activity of edible fruit extracts of Ziziphus abyssinica A. Rich. J Anim Plant Sci. 6:623–629.
- Orwa C, Mutua A, Kindt R, Jamnadass R, Simons A. 2009. Agroforestree database: a tree reference and selection guide version 4.0. [accessed 2016 Feb 17]. http://www.worldagroforestry.org/af/treedb/
- Randall LO, Selitto JJ. 1957. A method for measurement of analgesic activity on inflamed tissue. Arch Int Pharmacodyn Ther. 111:409–419.
- Rodger IW. 2009. Analgesic targets: today and tomorrow. Inflammopharmacology. 17:151–161.
- Sawynok J. 1998. Adenosine receptor activation and nociception. Eur J Pharmacol. 347:1–11.
- Schaible H-G, Ebersberger A, Natura G. 2011. Update on peripheral mechanisms of pain: beyond prostaglandins and cytokines. Arthritis Res Ther. 13:1–8.
- Schmid HA, Pehl U. 1996. Regional specific effects of nitric oxide donors and cGMP on the electrical activity of neurons in the rat spinal cord. J Chem Neuroanat. 10:197–201.
- Shah AS, Alagawadi KR. 2011. Anti-inflammatory, analgesic and antipyretic properties of Thespesia populnea Soland ex. Correa seed extracts and its fractions in animal models. J Ethnopharmacol. 137:1504–1509.
- Stein C, Clark JD, Oh U, Vasko MR, Wilcox GL, Overland AC, Vanderah TW, Spencer RH. 2009. Peripheral mechanisms of pain and analgesia. Brain Res Rev. 60:90–113.
- Tjølsen A, Berge O-G, Hunskaar S, Rosland JH, Hole K. 1992. The formalin test: an evaluation of the method. Pain. 51:5–17.
- Ugwah MO, Etuk EU, Bello SO, Aliero AA, Ugwah-Oguejiofor CJ. 2013. Comparative studies of anti-ulcerogenic activities of three Nigerian medicinal plants: a preliminary evaluation. J Med Plants Res. 7:490–495.
- Ugwah-Oguejiofor JC, Alkali IY, Ugwah MO, Abubakar K. 2013. Antidiarrhoeal potential of the aqueous root extract of Ziziphus abyssinica A. Rich. Sch Acad J Pharm. 2:419–423.
- Vale ML, Benevides VM, Sachs D, Brito GA, Rocha FA, Poole S, Ferreira SH, Cunha FQ, Ribeiro RA. 2004. Antihyperalgesic effect of pentoxifylline on experimental inflammatory pain. Br J Pharmacol. 143:833–844.
- Verri WA, Jr Cunha TM, Poole S, Ferreira SH, Cunha FQ. 2007. Cytokine inhibitors and pain control. Rev Bras Reumatol. 47:341–353.
- Villetti G, Bergamaschi M, Bassani F, Bolzoni PT, Maiorino M, Pietra C, Rondelli I, Chamiot CP, Simonato M, Barbieri M. 2003. Antinociceptive activity of the N-methyl-d-aspartate receptor antagonist N-(2-indanyl)-glycinamide hydrochloride (CHF3381) in experimental models of inflammatory and neuropathic pain. J Pharm Exp Ther. 306:804–814.
- Wachtel-Galor S, Benzie IFF. 2011. Herbal medicine: an introduction to its history, usage, regulation, current trends, and research needs. In: Benzie IFF, Wachtel-Galor S, editors. Herbal medicine: biomolecular and clinical aspects. 2nd ed. Boca Raton (FL): CRC Press, Taylor & Francis; p. 1–10.
- Wagate CG, Mbaria JM, Gakuya DW, Nanyingi MO, Kareru PG, Njuguna A, Kareru PG, Njuguna A, Gitahi N, Macharia JK, et al. 2010. Screening of some Kenyan medicinal plants for antibacterial activity. Phytother Res. 24:150–153.
- Watson JJ, Allen SJ, Dawbarn D. 2008. Targeting nerve growth factor in pain: what is the therapeutic potential? BioDrugs. 22:349–359.
- Willis WD. Jr. 2009. The role of TRPV1 receptors in pain evoked by noxious thermal and chemical stimuli. Exp Brain Res. 196:5–11.
- Woode E, Ameyaw EO, Boakye-Gyasi E, Abotsi WK. 2012. Analgesic effects of an ethanol extract of the fruits of Xylopia aethiopica (Dunal) A. Rich (Annonaceae) and the major constituent, xylopic acid in murine models. J Pharm Bioallied Sci. 4:291–301.