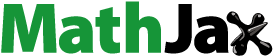
Abstract
Context
Bryophyllum pinnatum (Lam.) Oken (Crassulaceae) is used traditionally to treat many ailments.
Objectives
This study characterizes the constituents of B. pinnatum flavonoid-rich fraction (BPFRF) and investigates their antioxidant and anticholinesterase activity using in vitro and in silico approaches.
Materials and methods
Methanol extract of B. pinnatum leaves was partitioned to yield the ethyl acetate fraction. BPFRF was isolated from the ethyl acetate fraction and purified. The constituent flavonoids were structurally characterized using UPLC-PDA-MS2. Antioxidant activity (DPPH), Fe2+-induced lipid peroxidation (LP) and anticholinesterase activity (Ellman’s method) of the BPFRF and standards (ascorbic acid and rivastigmine) across a concentration range of 3.125–100 μg/mL were evaluated in vitro for 4 months. Molecular docking was performed to give insight into the binding potentials of BPFRF constituents against acetylcholinesterase (AChE) and butyrylcholinesterase (BuChE).
Results
UPLC-PDA-MS2 analysis of BPFRF identified carlinoside, quercetin (most dominant), luteolin, isorhamnetin, luteolin-7-glucoside. Carlinoside was first reported in this plant. BPFRF significantly inhibited DPPH radical (IC50 = 7.382 ± 0.79 µg/mL) and LP (IC50 = 7.182 ± 0.60 µg/mL) better than quercetin and ascorbic acid. Also, BPFRF exhibited potent inhibition against AChE and BuChE with IC50 values of 22.283 ± 0.27 µg/mL and 33.437 ± 1.46 µg/mL, respectively compared to quercetin and rivastigmine. Docking studies revealed that luteolin-7-glucoside, carlinoside and quercetin interact effectively with crucial amino acid residues of AChE and BuChE through hydrogen bonds.
Discussion and conclusions
BPFRF possesses an excellent natural source of cholinesterase inhibitor and antioxidant. The material could be further explored for the potential treatment of oxidative damage and cholinergic dysfunction in Alzheimer’s disease.
Introduction
Alzheimer’s disease (AD) is a chronic, progressive age-related neurodegenerative disorder characterized by the manifestation of multiple cognitive impairments, gradual loss of memory and behavioural abilities (Vijayan and Reddy Citation2016). The pathophysiology of AD is complex and multiple aetiological factors such as the formation of senile plaques composed of amyloid-β (Aβ) protein (Swerdlow Citation2007; Qiu and Fratiglioni Citation2018), the formation of neurofibrillary tangles (NFT) (Wischik et al. Citation2014), oxidative stress and substantial loss of cholinergic function (Asaduzzaman et al. Citation2014) have been postulated to explain the progression of AD.
The enzyme cholinesterase is considered a major therapeutic target for the treatment of AD (Vinutha et al. Citation2007; Nwidu et al. Citation2017; Ali Reza et al. Citation2018; Moodie et al. Citation2019). Studies have reported a positive correlation between memory impairment in AD patients and a loss of cholinergic function as well as diminished levels of the neurotransmitter acetylcholine (ACh) in the brain (Asaduzzaman et al. Citation2014; Chlebek et al. Citation2019). Inhibition of acetylcholinesterase (AChE) and butyrylcholinesterase (BuChE) has been shown to increase neural acetylcholine levels and increase communication between nerve endings, which make them first-line therapy for alleviating symptomatic mild to moderate AD (Moodie et al. Citation2019). Several synthetic drugs currently used for symptomatic relief of AD such as donepezil, galantamine, and rivastigmine are found to slow the breakdown of acetylcholine and accordingly, reinforces cholinergic neurotransmission (Jiménez-González et al. Citation2018). However, these drugs have been marked with significant side effects and only show short-term relief from symptoms (Sun et al. Citation2012).
In addition to the role of cholinesterase enzyme in AD pathology, mounting experimental evidence has implicated oxidative damage in AD pathology resulting from β-amyloid deposition (Fischer and Maier Citation2015). Aβ has been found to initiate neuron death by several cellular reactions, such as the formation of reactive oxygen species (ROS) and reactive nitrogen species (RNS), oxidation of lipids, and protein (Chauhan and Chauhan Citation2006; Wu et al. Citation2016). Oxidative stress increased in an aging brain is caused by a disproportion of the redox state, involving the generation of excess ROS and resulting in the oxidation of biomolecules leading to cellular damage (Muller et al. Citation2007; Huang et al. Citation2016). Furthermore, the high utilization of oxygen by the central nervous system makes the brain tissues more susceptible to oxidative damage (Ademosun et al. Citation2016). Strong experimental evidence has shown elevated levels of lipid peroxidation products such as malondialdehyde (MDA) and hydroxynonenal in the brains of patients with AD (Huang et al. Citation2016). Antioxidant systems act to neutralize the possible destructive effects of ROS by the production of antioxidants to scavenge excess oxidants (Luca et al. Citation2015; Ali Reza et al. Citation2018).
Bryophyllum pinnatum (Lam.) Oken (Crassulaceae) is a succulent perennial herb commonly known as ‘miracle plant’ and in Nigeria locally known as ‘Oda opue’ in Igbo and ‘Abamoda’ in Yoruba. It is widely distributed in tropical Africa, India, China, Australia, and South America (Afzal et al. Citation2013). Bryophyllum pinnatum flourishes throughout parts of Southern Nigeria and is commonly used in folk medicine for the treatment of many ailments (Afzal et al. Citation2013; Chibli et al. Citation2014). Several bioactive compounds including, phenolics, alkaloids, flavonoids, triterpenes, glycosides, steroids, bufadienolides, cardenolides, and organic acids, have been identified and documented in B. pinnatum (Fernandes et al. Citation2019). Bryophyllum pinnatum plant extracts are reported to possess several pharmacological activities including antimicrobial (Abubakar et al. Citation2014), neuropharmacological (Salahdeen and Yemitan Citation2006), anti-inflammatory and analgesic (Fürer et al. Citation2013), muscle relaxant and sedative (Plangger et al. Citation2006), gastroprotective (Afzal et al. Citation2013) and antioxidant (Sharma et al. Citation2014).
Polyphenolic compounds such as flavonoids have gained increasing attention for their development as phytotherapeutics, owing to their roles in biological function such as antioxidant, antibacterial, anti-inflammatory, anticancer properties, their ability to delay age-related functional and physiological deficits in the brain, and their capacity to mediate important cellular enzyme functions (Panche et al. Citation2016; Bensalem et al. Citation2018). To date, there is still an untapped reservoir of substrates present in plant secondary metabolites that could serve as safer alternatives for the management of AD and other neurodegenerative diseases (Huang et al. Citation2016; Ali Reza et al. Citation2018; Khan, Ali, et al. Citation2018). A recent report by Ojo et al. (Citation2018) and Elufioye et al. (Citation2019) showed that B. pinnatum exhibits strong antioxidant and anticholinesterase activity. However, insights into the possible bioactive compound and mode of interaction are yet to be established. Hence, this study provides a detailed identification and characterization of the purified flavonoid enriched fraction of B. pinnatum leaves. For this purpose, a high-resolution ultra-performance liquid chromatography (UPLC) was applied for short-run times coupled with a photodiode array detector (PDA) and a quadrupole/time-of-flight mass spectrometer (Q/TOF-MS) and the antioxidant capacity and cholinesterase inhibitory activity were evaluated. Molecular docking studies were further employed to examine the possible molecular interactions of B. pinnatum flavonoid constituents against cholinesterase.
Materials and methods
Plant material procurement, identification and authentication
Fresh leaves of B. pinnatum () were collected around the Nsukka area of Enugu State, Nigeria, in June 2018. They were identified and authenticated by botanist Dr. Grace Ugbabe at the herbarium of the National Institute of Pharmaceutical Research Abuja, Nigeria. A voucher specimen was deposited, and a reference No: NIPRID/H/6855 was obtained.
Plant preparation
The fresh leaves of B. pinnatum were carefully separated from the stem, washed with clean water to remove sand and debris, and then chopped into smaller pieces. The chopped leaves were spread under shade to drain completely. Subsequently, the leaves were air-dried to a constant weight. The dried plant leaves were pulverized into powdered form and stored in an air-tight container under room temperature (23–25 °C) devoid of light for two days.
Chemicals and reagents
UHPLC-MS solvents, LC-MS formic acid reagent, acetonitrile were purchased from Merck (Santiago, Chile). Ultrapure water was obtained from a Millipore purification system (Milli-Q Merck Millipore, Santiago, Chile). UHPLC standards with a purity higher than 95% for HPLC) were acquired from Sigma Aldrich (St. Louis, MO, USA). Quercetin, ascorbic acid, gallic acid, sodium trioxocarbonate (VI), sodium phosphate, 1,1-diphenyl-2-picrylhydrazyl radical (DPPH), trichloroethanoic acid (TCA), thiobarbituric acid (TBA), ferrous sulphate, ethyl acetate, methanol, ethanol, Dowex® 50WX8 hydrogen form, acetylcholine iodide (AChI), butyrylcholine iodide (BCEI) were purchased from Sigma Aldrich (St. Louis, MO, USA). Other reagents such as Folin-Ciocalteau, potassium bromide, hydrochloric acid, used for this study were of analytical grade and products procured from Qualikems Laboratories (India) and BDH Chemicals Ltd. (Poole, England). The water used for all analysis was glass distilled.
Enzyme sources
Whole-brain tissue from adult male Wistar rat was used as a source of AChE enzyme and a lipid-rich media for lipid peroxidation assay while rat blood tissue was used as a source of BuChE enzyme. Animals were procured from the Department of Zoology, Faculty of Biological Sciences, University of Nigeria Nsukka. The animals used were kept in plastic cages and maintained in controlled conditions with a 12 h light/dark cycle at a temperature 23 ± 2 °C with access to food and water ad libitium. The protocols for animal handling were performed according to the National and International Ethical Recommendations for Care and Use of Laboratory Animals (National Academy of Science Citation2011) with the guidelines strictly observed. Ethical approval was obtained from the Ethics and Biosafety Committee, Faculty of Biological Sciences, UNN with protocol number UNN/FBS/EC/1009.
Extraction of bioactive constituents
The preparation of the crude sample of B. pinnatum was carried out according to Kumar et al. (Citation2014). A known weight of 1600 g of dried and grounded plant material was macerated with methanol (80%) in distilled deionized water and macerated for 72 h. The extract was filtered, and the filtrate was centrifuged to separate the fine suspended particles. Next, the filtrate was concentrated using a rotary evaporator to obtain crude (methanol) extract. The extract was suspended in water and partitioned in ethyl acetate to obtain ethyl acetate fraction which was used for ion-exchange chromatography.
Resin activation and sample loading and elution
Activation of resin, isolation, and purification of flavonoid from the ethyl acetate fraction of B. pinnatum leaves as described previously by Omotuyi et al. (Citation2018). Dowex 50WX8 (5 g) cation resin was activated by treatment with 2-bed volumes (BV) of ethanol overnight followed by rinsing with distilled water (5 BV) under room temperature (25 ± 1 °C). The activated resin was then loaded on a column and 200 mL of the fraction was loaded onto 50 mL resin volume in every cycle and allowed to settle for 1 h. The packed column was thoroughly washed with deionized water to elute unbound impurities and sugars. Bound flavonoids, also known as B. pinnatum flavonoid-rich fraction (BPFRF), were eluted using 5% HCL: 95% ethanol (1% v/v) until A280 nm was close to 0.000 and the eluted samples were collected and used for the assay.
Phytochemical analysis
Estimation of total phenolic content (TPC)
The total phenolic content was measured following a described colorimetric method by Dohou et al. (Citation2003). Gallic was used as standard and expressed as milligrams of gallic equivalent per gram of sample (mg GAE/g fraction). The total phenolic content was determined using the standard curve (y = 0.0066x + 0.0032; R2 = 0.9889) obtained with gallic acid.
Estimation of total flavonoid content (TFC)
The total flavonoid content was measured following a described colorimetric method (Zhishen et al. Citation1999). Quercetin was used as standard and expressed as milligrams of quercetin equivalent per gram of sample (mg QE/g sample). The flavonoid content was determined using the standard curve (y = 0.0052x + 0.0574; R2 = 0.9823) obtained with quercetin.
In vitro bioactivity assay of BPFRF
In vitro antioxidant assay
2,2-Diphenyl-1-picrylhydrazyl (DPPH) radical scavenging assay
DPPH free radical scavenging activity of BPFRF was determined using the DPPH spectrophotometric method of Brand-Williams et al. (Citation1995) with slight modifications. Based on the principle, when DPPH reacts with an antioxidant compound that can donate hydrogen, it is reduced. The change in colour from deep violet to golden/light yellow can be measured at 518 nm. Briefly, 1 mL of 0.3 mM of DPPH solution was added to 1 mL of each of the test solutions. A BPFRF, quercetin or ascorbic acid (1 mL) at different concentrations (3.12–100 µg/mL) in 80% methanol was mixed with 0.5 mL of 0.076 mM DPPH in methanol. The mixtures were vortexed thoroughly and allowed to stand at room temperature in the dark for 30 min, and were incubated in the dark at room temperature for 30 min. The absorbance values were recorded at 518 nm, and converted into percentage antioxidant activity, using the formula below: Results were expressed as a percentage of inhibitions (100%). The concentration required to cause a 50% decrease in the absorbance (IC50) was, calculated from the standard plot. Each test was carried out in triplicates.
The % DPPH scavenging effect of the antioxidant was calculated as follows:
(1)
(1)
Fe2+-induced lipid peroxidation assay
Thiobarbituric acid reactive species (TBARS) assay was used to measure the lipid peroxide formed using brain tissue homogenates as lipid-rich media, as described by Ruberto et al. (Citation2000) with slight modifications. Brain homogenate (10% v/v) (0.5 mL) was added to 0.1 mL of the BPFRF, quercetin or standard (ascorbic acid) (3.125–100 µg/mL) and the volume was then made up to 1.0 mL with distilled water. Thereafter, 0.05 mL of FeSO4 was added and the mixture was incubated at 37 °C for 30 min. Then, 1.5 mL of acetic acid was added, followed by 1.5 mL of TBA in SDS. The resulting mixture was mixed and heated at 95 °C for 60 min. After cooling, 5 mL of butanol was added and the mixture was centrifuged at 3000 rpm for 10 min. The absorbance of the organic upper layer was measured at 532 nm and the percentage inhibition was calculated with the formula:
(2)
(2)
Acetylcholinesterase (AChE) and butyrylcholinesterase (BuChE) inhibitory activity
Ellman’s colorimetric method was applied to investigate the cholinesterase inhibitory activity of the BPFRF (Ellman et al. Citation1961). For the enzyme source, the rat brains were homogenized in a homogenizer with 10 volumes of a homogenization buffer (10 mM Tris-HCl, pH 7.2) containing 1 M NaCl, 50 mM MgCl2 and1% Triton X-100 and centrifuged at 10,000 rpm for 30 min. The resulting supernatants were collected, and the solution of these supernatants was used as an enzyme source. The temperature was maintained at 4 °C throughout the excretion procedure. The rate of AChE hydrolysis was monitored spectrophotometrically. Each BPFRF, quercetin or standard (rivastigmine) at varying concentrations (3.125–100 µg/mL) was mixed with an enzyme solution (500 μL) and incubated at 37 °C for 30 min. Absorbance at 405 nm was recorded immediately after adding Ellman’s reaction mixture (3.5 mL; 0.5 mM acetylthiocholine, 1 mM DTNB) in a 50 mM sodium phosphate buffer (pH 8.0) to the reaction mixture. Readings were repeated for 30 min to verify the reaction. Rivastigmine was used as a positive control. The percentage of AChE inhibition was calculated using the following formula:
(3)
(3)
Assessment of BuChE inhibition was performed as described in EquationEquation (3)(3)
(3) except that the enzyme solution was (100 μL) acetylthiocholine iodide was replaced with butyrylthiocholine iodide. All other reagents and conditions remained the same. The experiment was conducted in triplicate, and the concentrations of the test extract that inhibit the hydrolysis of the substrate (butyrylthiocholine) by 50% (IC50) were determined by linear regression analysis between the inhibition percentages against BPFRF concentration using Graph pad prism 5.0 program.
Identification and characterization of the flavonoid constituents in BPFRF
UPLC-PDA-ESI-QTOF-MS/MS instrumentation and chromatographic conditions
Qualitative and semi-quantitative UPLC-PDA-quadrupole time-of-flight mass spectrometer (MS) analysis of the BPFRF was carried out using the method of Stander et al. (Citation2017). High-resolution UPLC-MS2 analysis was carried out using a Waters Synapt G2 quadrupole time-of-flight (QTOF) mass spectrometer (MS) coupled to an Acquity ultra-performance liquid chromatography (UPLC) (Waters, Milford, MA, USA). A Waters HSS T3, 2.1 × 100 mm, 1.7 μm column was used for successful separation and an injection volume of 2 μL was used. The mobile phase was made up of 0.1% formic acid (solvent A) and acetonitrile containing 0.1% formic acid as solvent B. The gradient started at 100% solvent A for 1 min and changed to 28% B over 22 min in a linear way. It then moved up to 40% B over 50 s and a wash step of 1.5 min at 100% B, followed by re-equilibration to initial conditions for 4 min. The flow rate was 0.3 mL/min and the column was maintained at a temperature of 55 °C. Ion mobility data were obtained using the same UPLC gradient and column as above and IMS Wave velocity was set at 332 m/s and wave height at 20.2 V. For the MS conditions, electrospray ionization was used in negative mode with a cone voltage of 15 V, desolvation temperature of 275 °C, desolvation gas at 650 L/h, and the rest of the MS settings optimized for best resolution and sensitivity. Data were obtained by scanning across a range of m/z 50 to 1500 in resolution mode as well as in MSE mode. In MSE mode, two channels of MS data were used, one at low collision energy (4 V) and the second using a collision energy ramp (40–100 V) to obtain fragmentation data as well. Leucine enkephalin was used as lock mass (reference mass) for accurate mass determination and the instrument was calibrated with sodium formate (Stander et al. Citation2017). Semi quantification of major flavonoids present in the BPFRF sample was evaluated from the peak areas in the UPLC profile using external calibration curves.
Molecular docking studies
The X-ray crystallographic structure of the cholinergic target proteins, that is, the human AChE and BuChE were downloaded from RCSB Protein Data Bank (PDB). 3D structures of AChE and BuChE with PDB ID: 4EY7 (resolution 2.3 Å) (Cheung et al. Citation2012) and 1POM (resolution 2.38 Å) (Nicolet et al. Citation2003), respectively, were used. Before molecular docking simulations, the two PDB protein structures were prepared via the ‘Protein Preparation Wizard’ workflow in the Schrodinger suit. Briefly, hydrogen atoms were added to the protein, bond orders were assigned, and unnecessary water molecules were deleted retaining only water molecules interacting with residues at the active site of the enzyme. Furthermore, side chains and missing residues were added, and the partial charges were assigned. Minimization of energy was carried out using OPLS_2005 (Optimised potentials for liquid simulations) force field. As all the downloaded proteins were co-crystallized structures, the ligand-binding site was used to define the active site of the protein. Receptor grid generation workflow was used to define a grid (box) around the ligand, to keep all the functional residues in the grid (Sastry et al. Citation2013; Kumar et al. Citation2017). 2D structures of the identified ligands obtained from UPLC-PDA-ESI-MS/MS data were retrieved from the PubChem database. Ligprep was used to prepare and optimize the ligands prior to docking (Friesner et al. Citation2006); extra precision (XP) docking was employed to dock the prepared protein and the ligands. Structures of ligands were set as flexible to generate several conformations. Calculations were performed using the OPLS_2005 force field (Kumar et al. Citation2017). All the results were analyzed in XP visualizer.
Statistical analysis
The data obtained from the study were analyzed using Graph pad prism version 5.0. The results were expressed as mean ± standard deviation of triplicate measurements.
Results and discussion
Percentage yield of BPFRF
The extraction of 1.6 kg of the powdered leaves of B. pinnatum with methanol gave a yield of 4.77% of the powdered plant material soaked. Partitioning of 77 g of the MEBP partitioned in ethyl acetate resulted in 7.69 g of the ethyl acetate partition (EAF). Subsequent purification of the EAF by ion-exchange chromatography led to the formation of a purified flavonoid-rich fraction (BPFRF) with a percentage yield of 9.97%. The high BPFRF yield observed could be attributed to the purity of the fraction and method of purification (Omotuyi et al. Citation2018). Extraction solvent, nature, and conditions of the leaves, acidification percentage, drying temperature and storage conditions are important factors to be considered for the optimal yield of flavonoids from plants (John et al. Citation2005).
Total phenolic and flavonoid concentrations of the BPFRF
Bryophyllum pinnatum flavonoid-rich fraction (BPFRF) was examined for its total flavonoid and phenolic contents. The results indicated that the total flavonoid content of BPFRF was 132.87 ± 0.10 mg QE/g while the total phenolic contents were 83.98 ± 0.37 mg GAE/g. This result is incongruent with previously reported literature carried out on the methanol extract and ethyl acetate fraction of B. pinnatum leaves (Ojo et al. Citation2018). The enriched flavonoid content observed in this study could be attributed to the purification process; this is in agreement with the previous study by Liang et al. (Citation2019). Polyphenols have been found to possess high antioxidant activity as well as a plethora of favourable benefits to human health (Queen and Tollefsbol Citation2010; Ben Haj Yahia et al. Citation2019).
Identification and characterization of BPFRF by UPLC-ESI-QTOF-MS2
Several analytical techniques such as UV spectrometry, HPLC-DAD, GC-MS, HPLC-MS/MS, have been applied for the identification of polyphenolic compounds (Yang et al. Citation2020). UPLC-PDA-ESI-QTOF-MS2 has been reported to be most effective and sensitive for accurately identifying and characterizing the structures of known and unknown compounds (Yang et al. Citation2020). In this study, UPLC-ESI-QTOF-MS2 technique was employed in profiling and characterizing the flavonoid enriched fraction of B. pinnatum (BPFRF). Structures of BPFRF chemical compounds were detected and characterized by comparing their chromatographic and spectrometric data with scientific literature, open-access mass-spectra databases and authentic standards were available. The UV chromatogram and PDA spectra showed several peaks of UV-absorption of BPFRF compounds. Also, a cluster of large peaks was observed between 16 and 27 min. The UPLC-PDA-QTOF-ESI-MS/MS patterns of BPFRF are summarised in and , respectively. Peak 1a (compound 1a) was eluted at (Rt) 18.56 min ( and ) and exhibited UV spectra detected at (λ max of 255 and 370 nm) (). Compound 1a exhibited a molecular ion [M–H]– at m/z 579.1341 and daughter fragments at m/z 447, 415, 300, 301, 255, 243, 217, 178 (). The resulting elemental composition of compound 1a was (C26H27O15). So, compound 1a was tentatively identified as carlinoside, a flavone derivative of luteolin (8-arabinopyranosyl-6-glucopyranosyl luteolin). This compound’s mass spectra data is consistent with a similar compound present in Rooibos tea and reported by Stander et al. (Citation2017). Peak (2) eluted at retention time 22.51 min ( and ) and indicated a characteristic UV spectrum at 254 nm and 370 nm (). The [M–H]− ion parent fragment was m/z 301.0327 with molecular formula C15H9O7. The daughter fragmentation patterns generated were m/z 299, 273, 245, 178, 152, 121, 107 of different compounds fragmented from the parent flavonoid identified (). The identification of compound 2 was confirmed by comparing its UPLC retention time, UV and mass spectra which was similar to those reported in previous literature (Brito et al. Citation2014; Oufir et al. Citation2015; Garayev et al. Citation2018) quercetin (compound 2) was found to be the most abundant flavonoid in BPFRF sample with an estimated peak area of about 80%. Peak (3) eluted at (Rt) 25.50 min ( and ) and showed UV maxima at 263 nm and 367 nm () which is characteristic of flavones showing parent ions of m/z 285.0374 [M–H]− and assigned molecular formula of C15H9O6. The fragmentation patterns of m/z 301, 257, 229, 187, 178, 151, 121, 107 (), showing that compound 3 is an aglycone flavone identified as luteolin. Similar fragmentation patterns were observed from the parent compound previously reported by Li et al. (Citation2018) and Stander et al. (Citation2017). Peak 4 was eluted at the retention time of 26.03 min ( and ) with UV maxima of 254 nm and 370 nm () which is characteristic of flavonols showing parent ions of m/z 315.0477 [M–H]− and presents a molecular formula of C16H11O7. The mass fragmentation patterns with intensity normalized to 80 for the highest fragment resulted in a quercetin fragment at 300 (80), and other daughter fragment ions of m/z 300, 271, 255, 227, 178, 151, 148, 107 (), indicating that compound 4 is an aglycone flavonol identified as isorhamnetin (quercetin-3-methyl ether) (Garayev et al. Citation2018). Peak 7 was eluted at the retention time of 28.32 min ( and ) with UV maxima of 222.41 nm and 367 nm (), which is characteristic of flavones showing parent ions of m/z 447.2006 [M–H]− and presents a molecular formula of C21H20O11. The mass fragmentation patterns with intensity normalized to 100 for the highest fragment resulted in a luteolin fragment at 285.14 (100), and other daughter fragment ions of m/z 351, 311, 301, 286, 241, 216, 202,151 (), showing that compound 7 is an aglycone flavone luteolin-7-glycoside (cynaroside) (Li et al. Citation2018). To the best of our knowledge, carlinoside (8-arabinopyranosyl-6-glucopyranosyl luteolin) had not been previously reported as a constituent of B. pinnatum, while compounds 2–4 were already described (Fürer et al. Citation2013). It is noteworthy that of the 15 compounds detected and separated by the UPLC only the 5 flavonoid compounds were tentatively identified which mainly belong to two principal metabolite types: flavone and flavonol class of flavonoids. These flavonoids have been reported to possess potent anticholinesterase activity (Panche et al. Citation2016; Khan, Marya, et al. Citation2018) and neuroprotective effects (Khan, Ali, et al. Citation2018).
Figure 2. Overlay of UPLC chromatogram at 280 nm top and base peak intensity (BPI) chromatograms (bottom) of BPFRF.

Figure 3. Photodiode array detector (PDA) spectra and mass spectra (MS/MS) fragmentation pattern present in B. pinnatum flavonoid-rich fraction (BPFRF). Luteolin C-glucoside-C-arabinoside (carlinoside) (A). Quercetin (B). Luteolin (C). Quercetin-3-methyl ether (isorhamnetin) (D). Luteolin-7-glucoside (E).

Table 1. Constituents of BPFRF identified and characterized by UPLC-PDA-Q/TOF-MS2 analysis.
In vitro bioactivity assay of BPFRF
DPPH radical scavenging activity
Evaluation of the DPPH radical scavenging property of the BPFRF is presented in and . From our result, BPFRF, quercetin, and ascorbic acid caused a concentration-dependent increase in the inhibition of the DPPH radical . DPPH is a stable nitrogen-based free radical that functions as a substrate of radical-trapping reactions in this method (Assefa et al. Citation2016; Ben Haj Yahia et al. Citation2019). The BPFRF caused a fast conversion of the purple-colored DPPH radical to pale yellow. Reduction in absorbance shows the antioxidant activity of the fraction (Assefa et al. Citation2016). As shown in , BPFRF was found to be a better DPPH radical scavenger with an estimated IC50 value of 7.382 ± 0.79 µg/mL and R2 = 0.731 than quercetin (IC50 = 13.803 ± 0.34 µg/mL and R2 = 0.741) and the reference standard ascorbic (IC50 = 10.186 ± 0.14 µg/mL and R2 = 0.76). The IC50 values in µM were also presented for comparison, See Supplementary Table 1. This result agrees with previous studies that showed the radical scavenging activity of B. pinnatium crude extract against DPPH radical (Ojo et al. Citation2018; Gupta et al. Citation2016). Moreover, UPLC-PDA-QTOF-MS/MS identification of BPFRF showed the presence of some flavonoids () (i.e., carlinoside, quercetin (most abundant), luteolin, isorhamnetin and luteolin-7-glucoside) with evident antioxidant ability. These flavonoids may be mostly responsible for the potent antioxidant abilities exhibited by BPFRF. Quercetin, luteolin, isorhamnetin are potent radical scavengers owing to their ability to reduce the stable DPPH radical either by their capacity to donate a hydrogen atom or electron; thus, further converting the radicals into non-toxic species (Rahman et al. Citation2015).
Figure 4. 2,2-Diphenyl-1-picrylhydrazyl free radical scavenging effect of BPFRF in comparison with ascorbic acid (3.125–100 μg/mL).

Table 2. DPPH radical scavenging potential, lipid peroxidation inhibitory activity and cholinesterase inhibitory activity of BPFRF, quercetin and standards.
Lipid peroxidation inhibition activity
This study evaluated the inhibitory effect of BPFRF against non-enzymatic lipid peroxidation in rat brain homogenate. The inhibition of lipid peroxidation is considered the most important marker of oxidative stress (Badmus et al. Citation2011). In vitro lipid peroxidation was initiated by incubating rat brain tissue in the presence of FeSO4. Our results revealed that BPFRF, quercetin and the standard (ascorbic acid) inhibited Fe2+ induced lipid peroxidation in a dose-dependent manner . The IC50 (fraction concentration causing 50% inhibition of LP) values are presented in , BPFRF (IC50 = 7.182 ± 0.60 µg/mL and R2 = 0.86 had a higher inhibitory effect on Fe2+ induced lipid peroxidation in the brain homogenate compared to standard ascorbic acid (IC50 = 14.393 ± 0.54 µg/mL and R2 = 0.880) and quercetin (IC50 = 25.242 ± 1.97 µg/mL and R2 = 0.92). The IC50 values in µM were also presented for comparison, See Supplementary Table 1. These results are in agreement with the previous study carried out by Gupta et al. (Citation2016). These results showed that BPFRF can prevent cellular aberrations caused by ROS by breaking down the chain reactions responsible for lipid peroxidation (Ali Reza et al. Citation2018). The result obtained from the present study suggests the peroxide trapping property of BPFRF constituents. Brain tissues are made up of lipid-rich medium normally susceptible to per-oxidative attack (Chauhan and Chauhan Citation2006). The process of lipid oxidation is preceded by hydrogen atom removal from an unsaturated fatty acid, culminating in lipid peroxyl radical formation (Tönnies and Trushina Citation2017). Thus, the ability of BPFRF to inhibit Fe2+ mediated lipid peroxidation may largely be attributed to their distinct chemical structures, such as the number and position of hydroxyls and presence of double bonds on aromatic rings A and B as well as the heterocyclic ring C of the flavonoids identified in BPFRF () which donate H atom to fatty acyl chains in the membranes and scavenge OH radicals generated by Fenton’s reaction and Fe2+ chelating ability (Panche et al. Citation2016).
Evaluation of cholinesterase inhibitory activity
Evaluation of acetylcholinesterase (AChE) and butyrylcholinesterase (BuChE) inhibitory activity
One of the main pathological features of AD is the decline in cholinergic acetylcholine (ACh) neurotransmitters by cholinesterases. Studies suggest that an aberrant decrease in ACh leads to cognitive dysfunction and eventually death (Sun et al. Citation2012). Thus, inhibition of cholinesterases is one of the central focuses on developing natural therapeutics for AD (Feitosa et al. Citation2011; Suganthy and Pandima Citation2016). The inhibitory activity of BPFRF against rat brain AChE and serum BuChE was measured. BPFRF, quercetin and the standard drug Rivastigmine exhibited a concentration-dependent increase in the inhibitory activity of AChE and BuChE, respectively . shows the maximum inhibitory effects of BPFRF, quercetin, and standard against AChE and BuChE. BPFRF exhibited a strong inhibitory activity, with IC50 values of 22.283 ± 0.27 µg/mL and 33.437 ± 1.46 µg/mL against AChE and BuChE respectively compared to quercetin (15.528 ± 0.08 µg/mL and 24.326 ± 0.40 μg/mL) and the standard Rivastigmine (7.994 ± 0.12 µg/mL and 20.120 ± 0.72 µg/mL). The IC50 values in µM were also presented for comparison, See Supplementary Table 1. The results obtained from this study show appreciable IC50 values when compared to previously reported data on different crude extracts of B. pinnatum (Ojo et al. Citation2018). This indicates that the purified BPFRF conveys a potent inhibitory effect against AChE and BuChE. Khan, Marya, et al. (Citation2018) reported that the maximum AChE inhibitory activity of most flavonoids is due to the presence and position of the hydroxyl (OH) group at ring A and ring B and the unsaturation of ring C. The potent inhibitory activity of the partially purified BPFRF, maybe as a result of the presence of –OH groups on the side phenyl ring and interactions with important amino acid residues at the active site of the enzyme to form hydrogen bonds of the free OH groups in BPFRF flavonoids (). Thus, the anticholinesterase activity observed in this study suggests that the effect of BPFRF may not be due to only a particular flavonoid but could be due to the combined effect of the flavonoid constituents with different characteristics present in BPFRF.
Molecular docking
A molecular docking study was performed to evaluate the binding interaction of BPFRF constituents at the active site of AChE and BuChE to gain better insight into the in vitro experimental results. Molecular docking studies revealed that the BPFRF constituents exhibited favourable binding energies against AChE and BuChE activity. As shown in favourable overall binding energies were observed in ranges of −6.9 to −14.9 kcal/mol for AChE (4EY7) −8.9 to −14.9 kcal/mol and −12.7 to −6.9 kcal/mol for BuChE (1POM).
Table 3. Binding energies of BPFRF ligands and known inhibitors against AChE and BuChE drug targets.
The higher (negative) binding free energy, the more potent the interaction (Kumar et al. Citation2017). Luteolin-7-glucoside carlinoside and quercetin were found to have the highest binding affinities across the two targets compared to the synthetic inhibitors based on their binding energies (). Molecular docking provides a rough approximation of the binding affinity of a ligand for a given binding site (Ogidigo et al. Citation2018). The orientations of these ligands play a significant role in the interaction between active site residues (Raeisi Citation2013).
In the case of AChE, the active site of the human AChE is enclosed in a 20 Å deep pocket consisting of the catalytic site (Ser 203, His447 and Glu334), acyl-binding pocket (Phe295and Phe297) at the base of the gorge, quaternary ammonium binding locus (Trp86), oxyanion hole (Gly120, Gly121, and Ala204), and lastly, PAS (Tyr72, Asp74, Tyr124, Trp286 and Tyr341) (Johnson and Moore Citation2006; Kumar et al. Citation2017). Ligands interacting with these residues have been found to have a crucial function in the inhibition of the substrate. shows a 3D and 2D visual observation of the docked conformation of luteolin-7-glucoside at the AChE active site indicating interactions with the main residues at the active site. Luteolin-7-glucoside assumed a binding pose close to the catalytic anionic site (CAS) of AChE near the catalytic triad residues and formed crucial interactions. The hydroxyl group of the β-d-glucopyranosyl moiety of luteolin-7-glucoside was engaged in a hydrogen bond interaction with Tyr341, Phe295 and forming contact with an oxygen atom from the pyran ring (C-ring) . Also, pi–pi stacking with Trp286 (PAS residue) and Tyr341 (PAS residue) was established. Polar interactions with catalytic residue His 447 was observed. The docking simulation strongly correlates well with X-ray crystallographic structures of known AChE complexes (Cheung et al. Citation2012; Kumar et al. Citation2017). Furthermore, quercetin and Rivastigmine were observed to interact with the active site residues of AChE (Supplementary Table 1 and ).
Figure 7. Illustrations of molecular interactions (left: 3D and right: 2D) between the/highest binding energies of BPFRF constituents and standard against AChE target. Luteolin-7-glucoside (red) (Ai, Aii). Quercetin (orange) (Bi, Bii). Rivastigmine (purple) (Ci, Cii).

For BuChE, the catalytic site of human BuChE consist of 20 Å in its active site consisting of Ser198, His438, Glu325 catalytic amino acid residues found at the catalytic site. Other structural features of BChE include a choline-binding site or the cation-π site (Trp82), an oxyanion hole (Gly116, Gly117, Ala199), acyl binding site (hydrophobic pocket) (Leu286, Val288) and PAS (Asp70) (Kumar et al. Citation2017). Carlinoside showed the best binding energies with −12.7 kcal/mol compared to the known inhibitors Rivastigmine was −6.9 kcal/mol. Carlinoside also formed a hydrogen bond with Pro285, Ser 287, Asn 289 and noticeable polar interactions with two catalytic site residues His438 and Ser198 (Supplementary Table 2 and ). Similarly, quercetin and Rivastigmine interacted favourably with the active site residues Ser198, His438 and Glu325 (Supplementary Table 2 and ). These interactions observed may account for the possible mechanism of cholinesterase inhibition by BPFRF constituents. Thus, these findings fully support the in vitro experiment and corroborate the anticholinesterase activity of BPFRF.
Conclusions
Profiling of flavonoids from BPFRF by UPLC-PDA-QTOF-ESIMS/MS led to the identification of 15 compounds from which one flavone derivative carlinoside was reported for the first time in this plant species. BPFRF exhibited high polyphenol content and substantial antioxidant and anticholinesterase activities. The chemical constituents of BPFRF compounds exhibited favourable cholinesterase inhibitory activities evidenced by the in vitro and molecular docking simulation studies. The observed biological activities of BPFRF indicate the synergistic effect of the flavonoid constituents present in B. pinnatum leaves. Hence, B. pinnatum could emerge as an effective cholinesterase inhibitor and potent antioxidant agent against oxidative stress and free radical damage associated with AD; thus, these results provide a basis for the clinical validation of B. pinnatum as a promising source of neuroprotectant.
Author’s contribution
Authors OFCN, CAA and JOO designed the work; JOO conducted most of the in vitro assay, literature search and prepared the draft manuscript. DEE provided inputs on the antioxidant assay. Author CUI provided insight into the in silico experiment, Author JEP read the manuscript and Authors OFCN, BCN, CAA evaluated and approved the design, and supervised the work. All authors read and approved the final manuscript.
Supplementary_Data_PHB.doc
Download ()Acknowledgements
The authors hereby acknowledge Dr. Chukwuka Ogbonna for assisting with the sourcing of the plant material. Dr. Malcom J. Taylor of the mass spectrometry unit of the Central Analytical Facility (CAF), Stellenbosch University, South Africa, for assisting with the UPLC-PDA-QTOF-ESI-MS/MS analysis and data interpretation, as well as the members of the Centre for Bio-computing and Drug Design (CBDD), Adekunle Ajasin University, Nigeria, for the inputs on the isolation and purification of the BPFRF.
Disclosure statement
No potential conflict of interest was reported by the author(s).
Data availability statement
The data that support the findings of this study are available from the authors upon request.
References
- Abubakar AA, Oladele HA, Adejumoke A, Kayode FJ. 2014. Synergistic effect of combined extract of Bryophyllum pinnatum and Aloe barbadensis enhances anti-microbial activity in vitro. Glob Adv Res J Med Med Sci. 3:26–32.
- Ademosun AO, Oboh G, Bello F, Ayeni PO. 2016. Antioxidative properties and effect of quercetin and its glycosylated form (rutin) on acetylcholinesterase and butyrylcholinesterase activities. J Evid Based Complementary Altern Med. 21(4):NP11–NP17.
- Afzal M, Kazmi I, Anwar F. 2013. Antineoplastic potential of Bryophyllum pinnatum (Lam.) on chemically induced hepato carcinogenesis in rats. Phcog Res. 5(4):247–253.
- Ali Reza ASM, Hossain MS, Akhter S, Rahman MR, Nasrin MS, Uddin MJ, Sadik G, Khurshid AHM. 2018. In vitro antioxidant and cholinesterase inhibitory activities of Elatostema papillosum leaves and correlation with their phytochemical profiles: a study relevant to the treatment of Alzheimer’s disease. BMC Complement Altern Med. 18(1):123–131.
- Asaduzzaman M, Uddin M, Kader M, Alam A, Rahman AA, Rashid MK, Kato K, Tanaka T, Takeda M, Sadik G. 2014. In vitro acetylcholinesterase inhibitory activity and the antioxidant properties of Aegle marmelos leaf extract: implications for the treatment of Alzheimer’s disease. Psychogeriatrics. 14(1):1–10.
- Assefa AD, Ko EY, Moon SH, Keum YS. 2016. Antioxidant and antiplatelet activities of flavonoid-rich fractions of three citrus fruits from Korea. 3 Biotech. 6(1):1–10.
- Badmus JA, Adedosu TO, Fatoki JO, Adegbite V, Adaramoye OA, Odunola OA. 2011. Lipid peroxidation inhibition and antiradical activities of some leaf fractions of Mangifera indica. Acta Pol Pharm. 68(1):23–29.
- Ben Haj Yahia I, Zaouali Y, Ciavatta ML, Ligresti A, Jaouadi R, Boussaid M, Cutignano A. 2019. Polyphenolic profiling, quantitative assessment and biological activities of Tunisian native Mentha rotundifolia (L.) Huds. Molecules. 24(13):2351–2319.
- Bensalem J, Dudonné S, Gaudout D, Servant L, Calon F, Desjardins Y, Layé S, Lafenetre P, Pallet V. 2018. Polyphenol-rich extract from grape and blueberry attenuates cognitive decline and improves neuronal function in aged mice. J Nutr Sci. 7(e19):e19–e10.
- Brand-Williams W, Cuvelier ME, Berset C. 1995. Use of a free radical method to evaluate antioxidant activity. Lebensm-Wiss U-Technol. 28(1):25–30.
- Brito A, Ramirez JE, Areche C, Sepúlveda B, Simirgiotis MJ. 2014. HPLC-UV-MS profiles of phenolic compounds and antioxidant activity of fruits from three Citrus species consumed in northern Chile. Molecules. 19(11):17400–17421.
- Chauhan V, Chauhan A. 2006. Oxidative stress in Alzheimer’s disease. Pathophysiology. 13(3):195–208.
- Cheung J, Rudolph MJ, Burshteyn F, Cassidy MS, Gary EN, Love J, Franklin MC, Height JJ. 2012. Structures of human acetylcholinesterase in complex with pharmacologically important ligands. J Med Chem. 55(22):10282–10286.
- Chibli LA, Rodrigues KCM, Gasparetto CM, Pinto NCC, Fabri RL, Scio E, Alves MS, Del-Vechio-Vieira G, Sousa OV. 2014. Anti-inflammatory effects of Bryophyllum pinnatum (Lam.) Oken ethanol extract in acute and chronic cutaneous inflammation. J Ethnopharmacol. 154(2):330–338.
- Chlebek J, Korábečný J, Doležal R, Štěpánková Š, Pérez D, Hošťálková A, Opletal L, Cahlíková L, Macáková K, Kučera T, et al. 2019. In vitro and in silico acetylcholinesterase inhibitory activity of thalictricavine and canadine and their predicted penetration across the blood-brain barrier. Molecules. 24(7):1311–1340.
- Dohou N, Yamni K, Tahrouch S, Idrissi-Hassani L, Badoc A, Gmira N. 2003. Screening phytochimique d'une endémiqueibéro-marocaine, Thymelaea lythroides. Bull Soc Pharmacolo Bord. 142:61–78.
- Ellman GL, Courtney KD, Andres V, Feather-Stone RM. 1961. A new and rapid colorimetric determination of acetylcholinesterase activity. Biochem Pharmacol. 7:88–95.
- Elufioye TO, Olusola DM, Oyedeji AO. 2019. Correlation of total phenolic, flavonoid and tannin content of Bryophyllum pinnatum (Lam.) (Crassulaceae) extract with the antioxidant and anticholinesterase activities. Pharmacology J. 11:1003–1009.
- Feitosa CM, Freitas RM, Luz NNN, Bezerra MZB, Trevisan MTS. 2011. Acetylcholinesterase inhibition by somes promising Brazilian medicinal plants. Braz J Biol. 71(3):783–789.
- Fernandes JM, Cunha LM, Azevedo EP, Lourenço EMG, Pedrosa MF, Zucolotto SM. 2019. Kalanchoe laciniata and Bryophyllum pinnatum: an updated review about ethnopharmacology, phytochemistry, pharmacology and toxicology. Rev Bras Farmacogn. 29(4):529–553.
- Fischer R, Maier O. 2015. Interrelation of oxidative stress and inflammation in neurodegenerative disease: role of TNF. Oxid Med Cell Longev. 2015:610813–610818.
- Friesner RA, Murphy RB, Repasky MP, Frye LL, Greenwood JR, Halgren TA, Sanschagrin PC, Mainz DT. 2006. Extra precision glide: docking and scoring incorporating a model of hydrophobic enclosure for protein-ligand complexes. J Med Chem. 49(21):6177–6196.
- Fürer K, Raith M, Brenneisen R, Mennet M, Simões-Wüst A, Von Man-Dach U, Hamburger M, Potterat O. 2013. Two new flavonol glycosides and a metabolite profile of Bryophyllum pinnatum, a phytotherapeutic used in obstetrics and gynaecology. Planta Med. 79:1565–1571.
- Garayev E, Di Giorgio C, Herbette G, Mabrouki F, Chiffolleau P, Roux D, Sallanon H, Ollivier E, Elias R, Baghdikian B. 2018. Bioassay-guided isolation and UHPLC-DAD-ESI-MS/MS quantification of potential anti-inflammatory phenolic compounds from flowers of Inula montana L. J Ethnopharmacol. 226:176–184.
- Gupta S, Adak S, Rajak RC, Banerjee R. 2016. In vitro efficacy of Bryophyllum pinnatum leaf extracts as potent therapeutics. Prep Biochem Biotechnol. 46(5):489–494.
- Huang WJ, Zhang X, Chen WW. 2016. Role of oxidative stress in Alzheimer’s disease. Biomed Rep. 4(5):519–522.
- Jiménez-González A, Quispe C, Bórquez J, Sepúlveda B, Riveros F, Areche C, Nagles E, García-Beltrán O, Simirgiotis MJ. 2018. UHPLC-ESI-ORBITRAP-MS analysis of the native Mapuche medicinal plant palo negro (Leptocarpha rivularis DC. - Asteraceae) and evaluation of its antioxidant and cholinesterase inhibitory properties. J Enzyme Inhib Med Chem. 33(1):936–944.
- John KMM, Vijayan D, Kumar RR, Premkumar R. 2005. Factors influencing the efficiency of extraction of polyphenols from young tea leaves. Asian J of Plant Sciences. 5(1):123–126.
- Johnson G, Moore SW. 2006. The peripheral anionic site of acetylcholinesterase: structure, functions and potential role in rational drug design. Curr Pharm Des. 12(2):217–225.
- Khan A, Ali T, Rehman SU, Khan MS, Alam SI, Ikram M, Muhammad T, Saeed K, Badshah H, Kim MO. 2018. Neuroprotective effect of quercetin against the detrimental effects of LPS in the adult mouse brain. Front Pharmacol. 9:1–16.
- Khan H, Marya AS, Kamal MA, Patel S. 2018. Flavonoids as acetylcholinesterase inhibitors: current therapeutic standing and future prospects. Biomed Pharmacother. 101:860–870.
- Kumar P, Mir S, Semalty A. 2014. Isolation and characterization of novel flavonoid from methanolic extract of Pongamia pinnata pods. Research J of Phytochemistry. 8(1):21–24.
- Kumar S, Chowdhury S, Kumar S. 2017. In silico repurposing of antipsychotic drugs for Alzheimer’s disease. BMC Neurosci. 18(1):1–9.
- Li K, Fan H, Yin P, Yang L, Xue Q, Li X, Sun L, Liu Y. 2018. Structure-activity relationship of eight high content flavonoids analyzed with a preliminary assign-score method and their contribution to antioxidant ability of flavonoids-rich extract from Scutellaria baicalensis shoots. Arab J Chem. 11(2):159–170.
- Liang Z, Zhang J, Huang Y, Zhou C, Wang Y, Zhou CH, Xing SP, Shun QS, Xu YX, Wei G. 2019. Identification of flavonoids in Dendrobium huoshanense and comparison with those in allied species of Dendrobium by TLC, HPLC and HPLC coupled with electrospray ionization multi-stage tandem MS analyses. J Sep Sci. 42(5):1088–1104.
- Luca M, Luca A, Calandra C. 2015. The role of oxidative damage in the pathogenesis and progression of Alzheimer’s disease and vascular dementia. Oxid Med Cell Longev. 2015:1–8.
- Moodie LWK, Sepčić K, Turk T, Frangež R, Svenson J. 2019. Natural cholinesterase inhibitors from marine organisms. Nat Prod Rep. 36(8):1053–1092.
- Muller FL, Lustgarten MS, Jang Y, Richardson A, Van Remmen H. 2007. Trends in oxidative aging theories. Free Radic Biol Med. 43(4):477–503.
- National Academy of Science. 2011. Guide for the care and use of laboratory animals. Institute for laboratory animal research, national academy of sciences. 8th ed. Washington (DC): National Academies Press; p. 220.
- Nicolet Y, Lockridge O, Masson P, Fontecilla-Camps JC, Nachon F. 2003. Crystal structure of human butyrylcholinesterase and of its complexes with substrate and products. J Biol Chem. 278(42):41141–41147.
- Nwidu LL, Elmorsy E, Thornton J, Wijamunige B, Wijesekara A, Tarbox R, Warren A, Carter WG. 2017. Anti-acetylcholinesterase activity and antioxidant properties of extracts and fractions of Carpolobia lutea. Pharm Biol. 55(1):1875–1883.
- Ogidigo JO, Anosike C, Nwodo OFC, Omotuyi O, Nash O, Metibemu S, Eniafe G, Okpalafe O, Sani M. 2018. In silico molecular docking and pharmacokinetic studies of some selected phyto-constituents of Byrophyllum pinnatum as a potential selective inhibitor of MAO-B. Pharmacologyonline 3(45):14–49.
- Ojo OA, Ojo AB, Ajiboye BO, Olaiya O, Akawa A, Olaoye O, Anifowose OO, Idowu O, Olasehinde O, Obafemi T, et al. 2018. Inhibitory effect of Bryophyllum pinnatum (Lam.) Oken leaf extract and their fractions on α-amylase, α-glucosidase and cholinesterase enzyme. Pharmacology J. 10:497–506.
- Omotuyi OI, Nash O, Inyang OK, Ogidigo J, Enejoh O, Okpalefe O, Hamada T. 2018. Flavonoid-rich extract of Chromolaena odorata modulate circulating GLP-1 in Wistar rats: computational evaluation of TGR5 involvement. 3 Biotech. 8(2):124–132.
- Oufir M, Seiler C, Gerodetti M, Gerber J, Fürer K, Mennet-von Eiff M, Elsas SM, Brenneisen R, von Mandach U, Hamburger M, et al. 2015. Quantification of bufadienolides in Bryophyllum pinnatum leaves and manufactured products by UHPLC-ESIMS/MS. Planta Med. 81(12–13):1190–1197.
- Panche AN, Diwan AD, Chandra SR. 2016. Flavonoids: an overview. J Nutr Sci. 5(e47): 1–15.
- Plangger N, Rist L, Zimmermann R, von Mandach U. 2006. Intravenous tocolysis with Bryophyllum pinnatum is better tolerated than beta-agonist application. Eur J Obstet Gynecol Reprod Bio. 124(2):168–172.
- Qiu C, Fratiglioni L. 2018. Aging without dementia is achievable: current evidence from epidemiological research. J Alzheimers Dis. 62(3):933–942.
- Queen BL, Tollefsbol TO. 2010. Polyphenols and aging. Curr Aging Sci. 3(1):34–42.
- Raeisi S. 2013. Molecular docking approach of monoamine oxidase B inhibitors for identifying new potential drugs: Insights into drug-protein interaction discovery. J Cell Mol Res. 5 (1):24–33.
- Rahman MM, Islam MB, Biswas M, Khurshid Alam AH. 2015. In vitro antioxidant and free radical scavenging activity of different parts of Tabebuia pallida growing in Bangladesh. BMC Res Notes. 8(1):621–630.
- Ruberto G, Baratta MT, Deans SG, Dorman HJ. 2000. Antioxidant and antimicrobial activity of Foeniculum vulgare and Crithmum maritimum essential oils. Planta Med. 66(8):687–693.
- Salahdeen HM, Yemitan OK. 2006. Neuropharmacological effects of aqueous leaf extract of Bryophyllum pinnatum in mice. Afr J Med Health Sci. 9:101–107.
- Sastry GM, Adzhigirey M, Day T, Annabhimoju R, Sherman W. 2013. Protein and ligand preparation: parameters, protocols, and influence on virtual screening enrichments. J Comput Aided Mol Des. 27(3):221–234.
- Sharma A, Bhot M, Chandra N. 2014. In vitro antibacterial and antioxidant activity of Bryophyllum pinnatum (Lam.) Kurz. Int J Pharm Pharm Sci. 6:558–560.
- Stander MA, Van Wyk B, Taylor MJC, Long HSJ. 2017. Analysis of phenolic compounds in rooibos tea (Aspalathus linearis) with a comparison of flavonoid-based compounds in natural populations of plants from different regions. J Agric Food Chem. 65(47):10270–11028.
- Suganthy N, Pandima DK. 2016. In vitro antioxidant and anti-cholinesterase activities of Rhizophora mucronata. Pharm Biol. 54(1):118–129.
- Sun X, Jin L, Ling P. 2012. Review of drugs for Alzheimer’s disease. Drug Discov Ther. 6(6):285–290.
- Swerdlow RH. 2007. Pathogenesis of Alzheimer’s disease. Clin Interv Aging. 2:347–359.
- Tönnies E, Trushina E. 2017. Oxidative stress, synaptic dysfunction, and Alzheimer’s disease. JAD. 57(4):1105–1121.
- Vijayan M, Reddy PH. 2016. Stroke, vascular dementia, and Alzheimer’s disease: molecular links. J Alzheimers Dis. 54(2):427–443.
- Vinutha B, Prashanth D, Salma K, Sreeja SL, Pratiti D, Padmaja R, Radhika S, Amit A, Venkateshwarlu K, Deepak M. 2007. Screening of selected Indian medicinal plants for acetylcholinesterase inhibitory activity. J Ethnopharmacol. 109:359–363.
- Wischik CM, Harrington CR, Storey JMD. 2014. Tau-aggregation inhibitor therapy for Alzheimer’s disease. Biochem Pharmacol. 88(4):529–539.
- Wu L, Tong T, Wan S, Yan T, Ren F, Bi K, Jia Y. 2016. Protective effects of puerarin against Aβ 1-42-induced learning and memory impairments in mice. Planta Med. 83(03–04):224–231.
- Yang X, Bai ZF, Zhang DW, Zhang Y, Cui H, Zhou HL. 2020. Enrichment of flavonoid-rich extract from Bidens bipinnata L. by macroporous resin using response surface methodology, UHPLC-Q-TOF MS/MS-assisted characterization and comprehensive evaluation of its bioactivities by analytical hierarchy process. Biomed Chromatogr. 34(11):e4933.
- Zhishen J, Mengcheng T, Jianming W. 1999. The determination of flavonoid contents in mulberry and their scavenging effects on superoxide radicals. Food Chem. 64(4):555–559.