ABSTRACT
Introduction
There is an urgent need for specific and sensitive bioassays to augment biodosimetric assessments of unwanted and excessive radiation exposures that originate from unexpected nuclear/radiological events, including nuclear accidents, acts of terrorism, or the use of a radiological dispersal device. If sufficiently intense, such ionizing radiation exposures are likely to impact normal metabolic processes within the cells and organs of the body, thus inducing multifaceted biological responses.
Areas covered
This review covers the application of metabolomics, an emerging and promising technology based on quantitative and qualitative determinations of small molecules in biological samples for the rapid assessment of an individual’s exposure to ionizing radiation. Recent advancements in the analytics of high-resolution chromatography, mass spectrometry, and bioinformatics have led to untargeted (global) and targeted (quantitative phase) approaches to identify biomarkers of radiation injury and countermeasure efficacy. Biomarkers are deemed essential for both assessing the radiation exposure levels and for extrapolative processes involved in determining scaling factors of a given radiation countering medicinal between experimental animals and humans.
Expert Opinion
The discipline of metabolomics appears to be highly informative in assessing radiation exposure levels and for identifying biomarkers of radiation injury and countermeasure efficacy.
1. Introduction
Conventional biodosimetry techniques, such as the early monitoring of clinical prodromal symptoms (e.g. nausea, vomiting peripheral lymphocyte counts, etc.), are not all that effective or useful as specific biomarkers for select types of organ/tissue injuries within individuals exposed to less than acute doses of ionizing radiation or to radiation exposures delivered more chronically (e.g. fallout-associated exposures). A new generation of minimally invasive molecular biomarker-based assays are being developed in order to extend current biodosimetric assessments of absorbed ionizing radiation doses after total-body or partial-body exposures. An array of recently published studies provide strong support for a multi-metabolite profile obtained from a high-resolution mass spectrometry-based metabolomics platform that indicates more robust and accurate assessments of radiation toxicity of organs and tissues and thus, yield improved medical triage. There are several studies using different approaches to identify and validate biomarkers [Citation1,Citation2]. It is unarguable that exposure to sufficiently intense ionizing radiation will damage cells and tissues of the body. Further, it is axiomatic that the sensitivity of those ionizing radiation-exposed cells that reside within select, vital organs (i.e. those with high cell turnover rates) are directly related to cellular mitotic rate and indirectly proportional to the extent of cellular differentiation. Specifically, the cells of the lymphohematopoietic system, the lining of the gastrointestinal system, and gametocytes are maximally sensitive to radiation exposure. Contrary to this, central nervous system cells are significantly resistant to radiation [Citation3]. It is not possible at this time to identify universal biomarkers for radiation injury that would be valuable in a variety of scenarios such as total- and partial-body irradiation and for a wide range of radiation doses due to several limitations. There is a consensus among radiation biologists that there is no single, perfect method to assess radiation dose for all possible types and conditions of radiation exposures. Hence, there is a need to use complimentary and surrogate approaches to assess the absorbed radiation dose, and these efforts must be continued.
The development and availability of suitable radiation countermeasures for acute radiation syndrome (ARS) remains a substantial unmet medical need, and has been recognized as a high priority area by the U.S. government [Citation4]. Radiation countermeasures are urgently needed that are safe, easily administered, and effective at reducing or eliminating adverse health consequences to individuals, as well as minimizing the overall impact to the public’s health at large. Although there has been a long-standing effort to develop such countermeasures, there has been only limited success, especially in terms of getting these agents fully approved and promulgated by government authorities (e.g. United States Food and Drug Administration (US FDA)). To date, only limited radiomitigators for the hematopoietic subsyndrome of ARS (H-ARS) have been approved by the US FDA [Citation5–7], and not a single radioprotector (protective agents that can be administered prior to exposure) has been similarly approved. Further, no radioprotective or mitigative agent has been approved specifically for the gastrointestinal subsyndrome of ARS (GI-ARS). Importantly, all radiomitigators that have been approved by the FDA for H-ARS are actually in the category of repurposed drugs, which are by definition, drugs that have been in clinical use for other indications.
For the development of radiation countermeasures, critical milestones include the identification and validation of biomarkers for radiation injury and for assessing efficacy of a given countermeasure under consideration. A biomarker is an objective, qualitative biologic feature that can be quantified, and can indicate a specific biological, therapeutic, pathological entity, or process. In the context of radiation injury or the recovery of injury elicited by a given countermeasure, such biomarkers include, but are not limited to, the following: gene sequences, receptor expression, blood cell counts, miRNA, messenger RNA, long non-coding RNAs, proteins, citrulline, metabolites, lipidomes, microbiota, growth factors, cytokines, chromosomal aberrations, tooth enamel and fingernail-based markers, and other imaging-based measurements [Citation1]. Under the Animal Rule, the human dose of the countermeasure needs to be determined based on the efficacious dose for animals obtained from well-controlled animal studies. To find the doses of drug likely to show efficacy in humans, it is important to use drug-induced biomarkers as proxies for drug efficacy. However, the biomarkers for total-body or partial-body radiation exposure may be quite distinct and may respond in a manner different than that of a putative countermeasure [Citation8]. Biomarkers may associate with the mechanism by which the countermeasure decreases the detrimental effect of radiation exposure, and this may correlate with the desired clinical outcome with a decrease in morbidity and mortality. In addition, biomarkers are also used as signals to trigger the use of clinical interventions for the management and treatment of radiation injuries.
Metabolomics is an emerging discipline that provides a systems biology snapshot of the net expression of metabolites from several known pathways in response to exogenous challenges. Metabolites are products of biological cascades of proteomic and genomic processes, and their presence is associated with the individual’s cell/tissue phenotype, making them potentially ideal biomarkers for disease or injury progression, as well as for identifying the effects of medical countermeasures. Metabolite biomarkers are also quite useful due to their comparative ease of identification and validation in biological samples, such as biological fluids, that can be collected without invasive procedures. The relationship of such metabolites to manifest phenotype during both a steady state and during disease progression, is another valuable factor. Both untargeted (global) and targeted (quantitative phase) metabolomic techniques have been widely used in various settings of radiation injuries [Citation9].
2. Metabolites as biomarkers for radiation injury
Exposure to sufficiently intense ionizing radiation elicits a set of complex biological responses that involve gene expression and protein turnover; these processes may in part dysregulate metabolic processes emblematic of the ‘radiation-injured cell’ phenotype. Hence, metabolomics may be a powerful and useful tool for developing biomarkers for radiation injury assessments.
The first phase in radiation biomarker discovery using a metabolomics approach is an untargeted screening of sham-irradiated and irradiated samples or pre and post-irradiation samples that can identify a large number of candidate biomarkers. Such global metabolomics profiling yields high dimensional data sets requiring extensive bioinformatics analysis for data reduction and feature selection (). A typical workflow for the analysis of LC-MS data involves the use of a peak picking algorithm for deconvolution of raw data files to a preprocessed file that is amenable to downstream statistical and bioinformatics analyses. High-resolution mass spectrometry enables the detection of thousands of features in a single analytical run with high mass accuracy while greater sensitivity allows for the detection of low abundance metabolites missed by NMR. The MS spectral data is pre-processed using peak detection algorithms including publicly available tools like XCMS, Metalign, and MZmine. The pre-processed metabolomics data can be further analyzed using supervised and unsupervised multivariate statistical methods. Supervised methods include partial least squares discriminate analysis (PLS-DA), support vector machines (SVM), discriminant function analysis (DFA), univariate ANOVA, and median fold change (MFC). Unsupervised methods include principal component analysis (PCA), in addition to supervised and self-organized maps. These methodologies allow the researcher to reduce dimensionality of complex data and make meaningful inferences pertinent to the scientific question being addressed. Metabolites identified during global profiling need validation using reference standards [Citation10–12]. The second phase in biomarker development involves verification and validation using a targeted LC-MS approach that exploits information gathered from the untargeted approach to analyze and confirm trends of metabolite abundance. Like other platforms, quantitative measures of metabolites are performed with calibration curves and normalization procedures using internal standards [Citation10,Citation13]. Metabolomic trends may include the direction of perturbation, and expand into a time and dose-dependent response over time.
Figure 1. Flow diagram of non-targeted (global profiling) and targeted (select metabolites) metabolomics. Non-targeted approach has used NMR (Nuclear magnetic resonance), LC-MS (Liquid chromatography–mass spectrometry), and GC-MS (Gas chromatography–mass spectrometry) while targeted approach has used HPLC (High-performance liquid chromatography), UPLC (Ultra-performance liquid chromatography), LC-MS, and GC-FID (Gas chromatography – Flame-ionization detection)
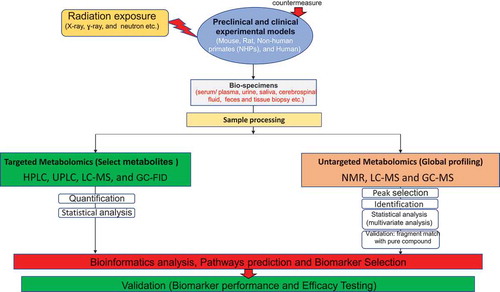
The following are the most frequently altered metabolites in response to different doses of radiation (wide dose range with varying dose rates and different qualities) exposure that have been detected and validated in various animal models and in clinical patient samples: carnitine (C7H15NO3), citric acid (C6H8O7), citrulline (C6H13N3O3), creatine (C4H9N3O2), creatinine (C4H7N3O), hypoxanthine (C5H4N4O), taurine (C2H7NO3S), xanthine (C5H4N4O2), uric acid (C5H4N4O3), and threonine (C4H9NO3). Other metabolites altered moderately include 2′deoxyuridine (C9H12N2O5), arginine (C6H14N4O2), glycine (C2H5NO2), glutamine (C5H10N2O3), hippuric acid (C9H9NO3), inositol (C6H12O6), palmitic acid (C16H32O2), uridine (C9H12N2O6), lactic acid (C3H6O3), leucine (C6H13NO2), linoleic acid (C18H32O2), methionine (C5H11NO2S), tyrosine (C9H11NO3), and sebacic acid (C10H18O4) [Citation9,Citation13–17].
Lipidomics is a subclass of metabolomics dealing with biochemical characterization of lipids in various biological samples. Plasma samples from animal models and humans contain a large number of lipids, which provide the opportunity to detect perturbations of cell and tissue lipids as a result of irradiation [Citation18]. Phospholipids (PLs)/glycerophospholipids are the most common class of lipids that are involved in important cellular processes, and are an integral component of the lipid bilayer of the cell membrane. Phosphatidylcholines (PCs) and other PL derivatives such as lysophosphatidylcholines (LPCs) are signaling molecules that participate in regulating cellular apoptosis and proliferation [Citation19]. Cholesteryl esters (CEs), diacylglycerides (DGs), LPCs, PCs, phosphatidylinositols (PIs), triacylglycerides (TGs), and sphingomyelins (SMs) have been detected in several lipid studies and their class structures were variable. The increase of sphingolipid ceramide was observed in several studies [Citation13,Citation19–22]. The most commonly affected lipid was decreased PC (36:1, 36 carbons in total with 1 double bond) levels in blood in mice, NHPs, and human studies [Citation19,Citation23–25]. Additional PCs and TGs detected in other studies were PC (34:0), PC (34:2), PC (36:2), PC (36:5), TG (58:7), TG (52:3), TG (56:6), TG (56:8), TG (58:3), and TG (58:4) [Citation19,Citation23–27].
The enrichment of extracellular vesicle fraction of metabolites indicative of stress response has also been described using quantitative ELISA [Citation28]. Plasma profiling demonstrated markers of dyslipidemia, oxidative stress, and inflammation post-irradiation. This study demonstrated enrichment of different classes of metabolites, such as N-acyl-amino acids, fatty acid ester of hydroxyl fatty acids, glycolipids, and triglycerides, as compared to the plasma metabolome composition with implications in mediation of systemic response to radiation induced stress signaling [Citation28].
Earlier results of lethally irradiated mice have demonstrated dysregulation of metabolites including lipids and branched-chain amino acids as early as 24 h after irradiation in serum [Citation29]. Analysis of murine and NHP urine and serum samples have shown decreased tricarboxylic acid cycle metabolites (citric acid, glucose and succinic acid) and tryptophan metabolism [Citation30–32]. As a result of a decrease in glycolysis and tricarboxylic acid activity, succeeding pathways were also dysregulated (decreased fatty acid oxidation and increased ketogenesis) [Citation32]. Creatinine and uric acid have been shown to decrease in response to irradiation in various animal models [Citation14]. Several studies have demonstrated decreased circulating citrulline levels in murine and NHP models following irradiation [Citation10,Citation33,Citation34]. Citrulline is an example of an organ-specific metabolite for irradiation. A reduced level of citrulline after irradiation has been shown to be associated with GI damage [Citation34]. Damage to mouse bone marrow after a short period of time (4–12 d) and a loss of mucosal surface in the GI after irradiation with high doses (4 to 8 Gy) have been reported [Citation34]. Furthermore, mouse jejunum have been shown to have decreased lipid and protein synthesis after irradiation [Citation35,Citation36]. Long-term damage (2 months) has been shown at lower doses (~2 Gy) which result in reduced nucleotide metabolism and inflammation in intestine [Citation37]. Sphingolipid, a ceramide, has been shown to be increased after irradiation in the lung in addition to several cell types in mice [Citation14]. Anti-ceramide antibody has been shown to protect mice from supralethal doses of radiation [Citation38].
Metabolites play an important role in human health and wellbeing. The intestinal microbiota converts ingested nutrients into metabolites that target the intestinal microbiota and host cells. Gut microbiota-derived metabolites play critical roles in maintaining health. Metabolites act as messengers of information between the intestinal microbiota and host cells [Citation15]. The GI microbiota composition and resulting metabolites impact host development, health, and pathogenesis [Citation39]. Studies have demonstrated modulation of the gut microbiota and their metabolites to improve health, and treat and prevent diseases. Tryptophan, an essential amino acid, plays an important role in physiological processes including neuronal function, immunity, and gut homeostasis and it is linked to central nervous system malignancy, inflammatory bowel, and cardiovascular disease [Citation40]. There is limited information in literature with metabolites and radiation medical countermeasures used for acute lethal doses of radiation, and this article address that gap in knowledge [Citation9,Citation14,Citation41].
2.1. Radiation injury
Radiation exposures can result in different categories of radiation injuries requiring different analytic, diagnostic, and therapeutic approaches. The clinical development of ARS depends on the absorbed dose of radiation, its quality and intensity, and its distribution within the body [Citation42]. The extent of radiation injury to lymphohematopoietic tissues, gastrointestinal and neurovascular systems, lung, and skin plays an important role in the diagnosis, treatment, and prognosis of exposed victims [Citation43]. ARS manifests following total-body irradiation in humans at estimated radiation doses of >1 Gray (Gy) delivered at high dose rates (e.g. >0.05 Gy/h). Clinical displays of ARS include H-ARS (2–6 Gy), GI-ARS (>6 Gy), and the neurovascular (>10 Gy) sub-syndrome [Citation44]. The neurovascular syndrome was previously known as the cardiovascular and central nervous system sub-syndromes prior to the recommendation by the International Commission on Radiological Protection for this term. The neurovascular syndrome is generally considered to be untreatable, and at such high levels of irradiation, death occurs fast (usually within 24–48 h) as a result of vascular problems and multi-organ failure. A cutaneous sub-syndrome, characterized by an array of blistering, necrotic and fibrotic skin lesions, can manifest late following high doses of radiation to the skin (~20 Gy or higher) [Citation45–47]. A wide range of radiation doses trigger H-ARS and GI-ARS, and their impact on mortality/morbidity is both dose and time-dependent. Due to the relatively high radiosensitivity of hematopoietic tissues, rapid and efficient diagnoses coupled with early interventions with mitigative therapies are critical for the exposed victims and their clinical management [Citation48]. Such classification of ARS sub-syndromes represents an over simplification of the disease process since multi-organ failure plays an important role for the outcome of radiation exposure [Citation49–51].
Individuals that have been exposed to low or moderate doses of ionizing radiation and that present clinically with H-ARS and/or GI-ARS are expected to respond more favorably to countermeasure treatments; hence, these two sub-syndromes have received the majority of attention in terms of research and radiation medical countermeasure development. Radiation medical countermeasures have been classified into three groups based on the time of administration of such agents in relation to exposure: radioprotectors, radiomitigators, and radiation therapeutics [Citation52]. Despite significant progress over the last six decades in the research and development of safe, nontoxic, and efficacious medical countermeasures, relatively few agents have been approved by the US FDA for human use [Citation53]. Since these ARS medical countermeasures are largely being developed following the US FDA’s Animal Rule, a brief description and discussion of this regulatory guidance is particularly pertinent to this review of the analytics of radiation-associated metabolomics and its potential impact(s) on developing and accessing efficacy of medicinals that might counter ionizing radiation injury to cells, tissues, and organ systems of the body.
Though the focus of this article is on ARS and the early phases of pathogenesis, survivors of ARS are likely to suffer from late arising pathologies as well. These delayed effects of acute exposure, currently referred to by the acronym DEARE, involve different pathologies of different organs of the body, including the lungs, bone marrow, GI tract, kidneys, heart, and the central nervous system [Citation54]. DEARE takes months to years to develop and often results in multi-organ failure and death. For example, DEARE manifests as fibrosis in both the lungs and in the bone marrow [Citation55–57]. Late-arising cancers of various organs are well recognized, but fairly low frequency pathological events follow acute ionizing radiation exposures [Citation58,Citation59].
2.2. Metabolomic techniques
The optimal metabolite-based biomarker assay should be accurate and sensitive (in terms of assessing ionizing radiation exposure), rapid, and easy to measure, while requiring minimal training for individuals to conduct such assays. The metabolomic profiles of various organisms are complex and require different techniques to optimally identify the diversity of metabolites present in a sample [Citation60]. Saccharomyces cerevisiae (yeast) contains roughly 600 metabolites [Citation61], while the plants have approximately 200,000 primary and secondary metabolites [Citation62]. The human metabolomic profile is highly complex and is considered to significantly exceed these values. The two major techniques that are used for metabolomic analysis are nuclear magnetic resonance (NMR) and mass spectrometry (MS). NMR has low sensitivity and high reproducibility, and quantifies more abundant molecules. This technology is also used to identify structures of unknown compounds. One-dimensional [Citation1]H NMR is the most commonly used approach in metabolomics. In brief, NMR offers a number of significant benefits including high reproducibility, nondestructive sample analysis, and quantification with accuracy [Citation63,Citation64], while some shortcomings include low sensitivity, requiring a large sample volume, and its limitation in identifying high abundance metabolites. Due to ease of use and high sensitivity and resolution, there has been an exponential increase in the number of publications that use mass spectrometry-based approaches for biomarker development, while NMR is found more suitable for specialized metabolome applications that do not require high throughput analyses. Proponents of NMR, however, believe that it is underappreciated, and suggest that both techniques should be used complimentarily [Citation65].
MS offers higher sensitivity, high throughput, is accurate and quantitative, and also offers well-resolved metabolomic profiles depending on the type of MS approach used [Citation65,Citation66]. LC-MS/MS offers excellent specificity and sensitivity, Fourier-transform ion cyclotron resonance (FTICR) provides superior mass accuracy and resolution, and desorption electrospray ionization (DESI) yields spatially resolved metabolite profiles that can be visualized as an image [Citation67]. The use of complementary separation techniques such as ultra-performance liquid chromatography (UPLC), gas chromatography (GC), and capillary electrophoresis (CE) can minimize background and increase metabolomic coverage [Citation68]. It is important to be mindful of the strengths and weaknesses of each technique to optimize the identification of untargeted metabolites. All of the techniques used in metabolomics have recently been reviewed [Citation41]. We have presented the effects of ionizing radiation exposure on metabolomes and lipidomes in context of various radiation countermeasures under development in this article.
2.3. Types of samples used for metabolomics studies
Sample preparation for LC-MS metabolomic analysis, rapid preparation protocols for blood, and metabolite trends from various biosamples across multiple species, radiation doses, and time-points have been recently discussed [Citation14,Citation69,Citation70]. However, pre-analytical variables including inconsistent sample collection, processing and storage protocols can adversely impact downstream data analysis. In addition, metabolite profiles are influenced by diet as well as circadian and seasonal variations, and hence, it is imperative to control for these variables while designing radiation metabolomic studies.
3. Need of biomarkers for the development of radiation countermeasures
To date, only four agents have been approved by the FDA (Neupogen, Neulasta, Npand Leukine) for use in medically managing a single, sub-syndrome of ARS. These approved medicinals are all radiomitigators only to be used shortly after radiation exposure, and are designed to counter specifically H-ARS [Citation5,Citation7,Citation71–77]. These radiation medical countermeasures were in clinical use for several decades for other indications prior to their approval by the FDA as radiomitigators for H-ARS. No additional, new chemical agent has yet been FDA-approved as a radiation countermeasure for ARS [Citation78].
The development of radiation countermeasures requires the determination of an efficacious drug dose for humans to be based on studies conducted with animal models, and such determination of a proper and effective drug dose can be derived, in part, through biomarker analysis [Citation1,Citation79]. To estimate the dose of a countermeasure that would yield a therapeutic benefit to a patient, one would use a countermeasure-induced biomarker response as a surrogate for drug efficacy. In brief, the estimated efficacious dose for a specific countermeasure in humans can be expressed as a drug dose that stimulates the surrogate biomarker to the same degree as the demonstrated efficacy in animal models [Citation8]. The concept of drug-dose conversion between species of experimental animal models and humans is critical for drug development [Citation80]. Allometric scaling for dose conversion from various animal models to humans is based on the normalization of the dose per unit of body weight to bodily surface area. This strategy considers differences with respect to biochemical, anatomical, and physiological attributes as well as differences in pharmacokinetics. Different calculation methods for various preclinical animal models and humans have been elegantly discussed in an article which can be used as a reference for interspecies dose conversions [Citation80].
Briefly, biomarker induction that is dependent on the countermeasure’s mechanism of action should occur in both unirradiated and irradiated animals. The biomarker should be expressed in response to the administration of an efficacious dose of the countermeasure, and the response should correspond with the dose-dependent efficacy. The nature of the biomarker should be relevant to reducing the mortality and morbidity following radiation exposure, have the ability to be quantified using samples obtained by relatively noninvasive techniques, and have a demonstrable response across multiple species [Citation81]. Since animal diseases seldom recapitulate comparable human diseases, the drug efficacy data generated from animals may not be as conclusive as the human-derived data. To gain confidence that a specific drug will be effective in humans, it is vital to know how and why the drug will be effective for a particular indication. In this respect, biomarkers are crucial for countermeasure development. They are used not only as a clinical signal (as a trigger) to implement drug intervention, but are also used in selecting a drug dose, as well as for determining a given treatment regimen. Only a limited number of countermeasures have been investigated for identifying metabolites as efficacy biomarkers (as discussed below), but several additional agents are under investigation (unpublished observation). A collection of perturbed metabolites in irradiated or unirradiated animals treated with countermeasures are mentioned below under the section for different radiation countermeasures investigated.
3.1. US FDA animal rule
The US FDA Animal Rule suggests that a countermeasure may get marketing approval to treat or prevent a life-threatening or serious condition triggered by a permanently disabling or lethally toxic agent if animal efficacy investigation satisfactorily corroborates that the countermeasure will yield a clinical advantage [Citation79,Citation82,Citation83]. Countermeasures developed following the Animal Rule should follow the prevailing requisites for establishing the safety of new agents. The FDA will depend on the animal efficacy investigations to determine if the four criteria mentioned below are met:
The pathophysiological mechanism of injury or toxicity by the causal agent/stimulus is well-understood, as well as the role of the countermeasure in the prevention or treatment of the referred injury or toxicity.
The effectiveness of the countermeasure is shown in more than one animal species with a response predictive of human response, or a single animal species that has been well-characterized for envisaging the clinical response in humans.
The animal investigation endpoints undoubtedly correlate to the desired clinical result in humans, normally decreased morbidity or mortality.
The efficacious dose can be determined from kinetics and pharmacodynamics of the countermeasure (or relevant data) in both humans and animals.
This Animal Rule is expected to be used in the development of medical countermeasures that respond to debilitating or lethal conditions stemming from either accidental or deliberate exposure to chemical, biological, radiological, or nuclear agents when clinical efficacy studies in humans are not ethical or feasible.
The Animal Rule does not completely eliminate the evaluation of the drug in humans. Clinical trials under phase I are still needed to test the safety/toxicity of the countermeasure to determine the appropriate dose of the drug for humans. Substitution of animals for humans in efficacy testing does not make it easier to get FDA approval for a drug. Animal models of human disease or injury seldom, if ever, replicate human disease. Therefore, efficacy data generated in an animal model will not be as compelling as human data.
For countermeasure development following the Animal Rule, drug dosage needs to be extrapolated for humans based on the dose that was found to be most efficacious in the animal model. An important approach for human-dose selection may be through the identification and validation of an appropriate biomarker. The biomarker should be linked to the mechanism by which the countermeasure prevents or significantly reduces the etiologic agent-induced disease or condition and the anticipated clinical result. The expected clinical outcome may be decreased morbidity and/or mortality. Further, one needs to determine the dose of a given countermeasure in humans that can elicit the biomarker response that can be correlated with drug-induced responses within a well-controlled animal study. In the recent past, various omics platforms have been used to identify and validate such biomarkers to expedite the development and regulatory approval of radiation countermeasures [Citation84–86].
3.2. BIO 300
BIO 300 is a promising, new radiation injury mitigative agent under advanced development for H-ARS and DEARE, as well as for select oncologic indication(s) (e.g. non-small cell lung cancer (NSCLC)) [Citation87]. The active pharmaceutical ingredient (API) in BIO 300 is synthetic genistein. Efficacy following oral administration, lack of clinical side effects, drug storage at room temperature, and intended dual use (radiation countermeasure and anti-cancer agent) makes BIO 300 an optimal agent for civilian and military use. There are several ongoing studies using various animal models to identify biomarkers of efficacy and dose conversion for BIO 300 [Citation87]. Studies of metabolomics with BIO 300 in murine and NHP models are discussed below.
3.2.1. Metabolomic study in murine DEARE model
Targeted metabolomic studies with BIO 300 were conducted to identify potential biomarkers for lung injury and BIO 300 efficacy for mitigating radiation-induced tissue damage. Male C57L/J mice were treated daily with BIO 300 (400 mg/kg) for 2 or 6 weeks starting 24 h after 12.5 Gy whole-thorax lung irradiation (WTLI, ~LD90/180), and metabolomic analysis was conducted at 180 days post-irradiation [Citation88,Citation89].
Sphingolipids, including sphingomyelin (SM) and hydroxylated SM species were found to be highly correlated with BIO 300’s apparent radioprotective efficacy. SM forms a lipid raft domain within the plasma membrane and is essential for cell signaling and endothelial cell stress response [Citation90]. There was a decrease in sphingomyelinase activity which metabolizes SM into ceramide [Citation91]. Increased ceramide from the breakdown of SM disrupts lipid rafts, impairing cellular signaling that leads to increased apoptosis and inflammation [Citation21]. The presence of these select metabolites appears to distinguish an efficacious treatment schedule of BIO 300 that improves 180 day survival after 12.5 Gy WTLI from a non-efficacious treatment schedule; as such, these metabolites are likely biomarkers that could be validated for use in the development of BIO 300 and for its dose translation from animal to human.
3.2.2. Metabolomic study in NHP model
Serum samples from NHPs treated with single doses of BIO 300 either orally (po) or intramuscularly (im) were analyzed using ultra-performance liquid chromatography (UPLC) quadrupole time-of-flight mass spectrometry (QTOF-MS) to study global metabolomics and lipidomic changes [Citation92]. Appropriate comparisons were made to establish how im and po administration of BIO 300 changes metabolomic profiles. Alterations in glycerophosphocholine, glycerophosphoserine, phenylalanine, and tyrosine were detected, and levels of these metabolomes returned to pretreatment levels by seven days post-BIO 300 administration. The changes in the metabolite profiles induced after po and im administration of BIO 300 overlapped. BIO 300 administered po produced fewer metabolic changes compared to the im administration. This may be due to differences in the absorption and distribution of the drug following po and im administrations. Ingenuity functional pathway analysis demonstrated changes in the biosynthesis of phenylalanine degradation pathways after po administration of BIO 300. Functional pathway analyses also identified changes in glycerophospholipid and bile acid metabolism after im administration of BIO 300. These results demonstrate that the administration of BIO 300 leads to metabolic changes, and these metabolic changes may provide benefit in controlling the extent and duration of radiation-elicited injury by influencing various pathways related to radiation injury. This study highlights the use of metabolomics and lipidomics to uncover the underlying physiological mechanisms (such as drug stabilizing plasma membrane bilayer, ion channels, signaling systems, etc.) implicated in the radioprotective efficacy of this countermeasure.
3.3. Gamma-tocotrienol (GT3)
Vitamin E is a family of agents that function as antioxidants, that control free-radical production, regulate peroxidation reactions, as well as being recognized for their anti-inflammatory, antioxidant, and neuroprotective attributes [Citation93–95]. The Vitamin E family has eight components belonging to two different groups of four saturated analogues (α, β, γ, and δ) called tocopherols and unsaturated analogues (α, β, γ, and δ) called tocotrienols. These compounds are referred to collectively as tocols. GT3 is known as an effective inhibitor of 3-hydroxy-3-methylglutaryl-coenzyme A (HMG-CoA) reductase. Its antioxidant property was the primary reason for its initial investigation as a radioprotective agent, and it is currently recognized as the most promising countermeasure among tocols [Citation95,Citation96]. GT3 has also been investigated for its effects on modulating microRNA with an aim to identify radioprotective efficacy biomarkers [Citation85,Citation86].
3.3.1. Metabolomic study in unirradiated NHP
It has been demonstrated in an NHP model that alterations in metabolite profiles manifest 24 h after gamma-tocotrienol administration, but not earlier. Such metabolic changes were linked to an increase in the bioavailability of antioxidants including cholic acid, lactic acid, docosahexaenoic acid, and 3-deoxy-vitamin, an anti-inflammatory metabolites D3 [Citation97]. These results demonstrated that gamma-tocotrienol treatments produce metabolic shifts that are likely to yield protective benefits in combating injurious effects of ionizing radiation. Further, this study of global metabolomic and lipidomic effects appear to indicate that gamma-tocotrienol administration does not lead to any major adverse effects. In brief, serum metabolic profiles present insights into biochemical alterations produced by gamma-tocotrienol administration that may contribute to its radioprotection.
There appeared to be underlying differences in the metabolomic/lipidomic profiles of male and female NHPs at baseline; differences that are likely due to the gender-specific physiology [Citation97]. However, the time-points with the widest divergence from the untreated controls showed no significant gender effect on metabolite profiles. Among the top 20 validated metabolic biomarkers, only 3-deoxy-vitamin D3 at day 4 showed a significant adjusted p-value. The significance level of this observation diminished toward the end of the study period. With respect to validated biomarkers, no significant separation was observed between males and females in the control groups. These findings may be due to the fact that gamma-tocotrienol administration demonstrated limited metabolic alterations in the drug-administered NHPs and hence, gender effects may be too subtle under the current analytic parameters.
3.3.2. Metabolomic study in irradiated NHPs
A study using 16 NHPs (two groups of 8 NHPs each treated with gamma-tocotrienol and vehicle, respectively) irradiated with 6.5 Gy (dose rate 0.6 Gy/min) has been reported [Citation31]. The blood samples from these animals demonstrated several metabolites were altered significantly after irradiation, such as compounds involved in fatty acid β-oxidation, amino acid metabolism, and purine catabolism. The machine-learning algorithm, Random Forest, distinguished untreated controls (samples collected prior to drug administration and irradiation), gamma-tocotrienol-treated and irradiated animals, and vehicle-treated and irradiated NHPs at 12 h and 24 h, as observed in a multidimensional scaling plot. Primary metabolites validated in this study included carnitine/acylcarnitines, creatine, amino acids, and xanthine. Gamma-tocotrienol administration reduced high fluctuations in serum metabolite levels, suggesting possible beneficial dampening of these metabolite swings within in irradiated animals. This study emphasizes the usefulness of metabolomics in ascertaining underlying physiological mechanisms responsible for the efficacy of gamma-tocotrienol as a radioprotector.
3.4. Amifostine
Amifostine (WR2721, Ethyol) is a radioprotector which has been shown to reduce acute radiation injury mainly through free radical scavenging, although other cytoprotective mechanisms been reported [Citation98]. It also provides protection against late-arising, radiation-induced malignancies by dampening radiation-induced mutagenesis [Citation99–102]. Amifostine is an FDA-approved agent for limited indications: (i) to reduce the renal toxicity as a result of repeated use of cisplatin in ovarian cancer patients, and (ii) to reduce the xerostomia in head and neck cancer patients receiving radiotherapy [Citation103–107]. Due to adverse side effect issues, amifostine failed to get US FDA approval as a radioprotector for ARS [Citation98]. Nevertheless, it has been shown experimentally that by using its low doses prophylactically, its toxic side effects can be minimized and radioprotective efficacy can be retained [Citation102,Citation108,Citation109].
3.4.1. Metabolomic study in murine model
A metabolomics study coupled with a survival-based analysis was conducted using a murine CD2F1 male mouse radiation model: the ‘survival’ leg of the study was comprised of groups of mice given two distinct doses of the radioprotective drug, amifostine (or for controls administered drug-vehicle alone), shortly before (~30 min) either radiation exposure (9.6 Gy, LD70/30, dose rate 0.6 Gy/min) or sham-irradiation, with subsequent monitoring of survival (over ~30 d). For the metabolomics leg of the study, different organs were collected. In brief, the results of the study’s ‘survival’ leg confirmed prior reported observations [Citation36,Citation102]. The survival difference between 50 mg/kg and 200 mg/kg was significant (33% vs 100%, p = 0.001), suggesting that 200 mg/kg was optimal, and 50 mg/kg was the suboptimal dose for survival benefit against a lethal dose of radiation in the murine model [Citation36]. In terms of the ‘metabolomics’ assessments, global metabolomic and lipidomic alterations were investigated using UPLC QTOF-MS in jejunum, bone marrow, and lung tissue samples of amifostine/saline-treated mice either sham irradiated or gamma-ray irradiated. Metabolomic results suggested that irradiation results in tissue-specific metabolic changes were rectified partly by treatment with amifostine in a dose-dependent manner () [Citation36]. Specifically, bone marrow cells demonstrated robust changes in responses to irradiation, and equally robust changes to radioprotective doses of amifostine. These noted metabolic changes within marrow samples were greater than those seen in other organs; e.g. lung and jejunum. Comparative studies of the metabolomic and lipidomic profiles in bone marrow demonstrated restoration of a large number of endogenous metabolites. The endogenous levels of long-chain acylcarnitines (LCACs), N-acyl amino acids, phosphatidylethanolamines, and inositol changed in response to irradiation, and were corrected by amifostine treatment in a dose-dependent manner.
Figure 2. Experimental approach and analytical design of the metabolomic study using irradiated animals treated with radiation countermeasures. Blood/tissue samples are collected from irradiated or countermeasure-treated plus irradiated animals on various time-points pre and post-irradiation/treatment, and processed for untargeted LC-MS profiling. Metabolomics pathway and network analysis are analyzed, and validation is performed. LC-MS (Liquid chromatography–mass spectrometry), Tandem MS (Mass spectrometry)
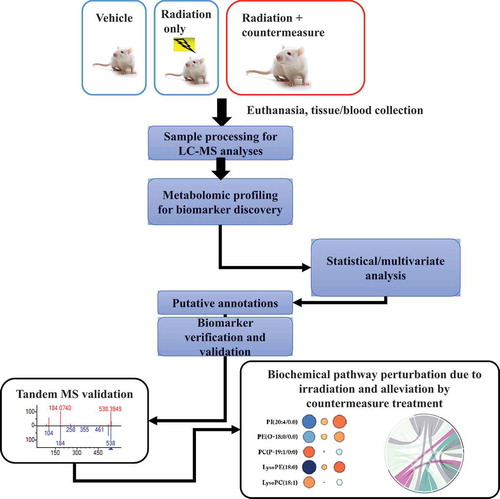
The analysis of jejunum revealed that bile acids and glycerophospholipids were maximally correlated with amifostine-mediated attenuation of radiation-induced metabolic disturbances. Pathway analysis demonstrated corrections of vitamin A, folate, and amino acid metabolism with the lower administered dose of amifostine, while at the higher drug dose, a higher number of pathway corrections were noted. These pathways included arachidonic acid metabolism, steroid hormone biosynthesis, glutathione, and amino acid metabolism. In sum, these results demonstrated that the higher treatment dose of amifostine resulted in broad-based metabolic pathway corrections, while the lower dose offered robust but partial alleviation of the radiation-induced metabolic alterations. Most importantly, amifostine treatment did not appear to elicit major metabolic perturbations in the sham-irradiated mice. In a follow-up study, additional organs such as the heart, spleen, and kidney were investigated for metabolic corrections of radiation-induced changes by amifostine in mice [Citation110]. The administration of amifostine partly corrected the metabolic perturbations present in the selected tissues. Heart and spleens were the most responsive tissues relative to metabolic changes and the extent of correction by amifostine treatments, regardless of drug dosage. By contrast, the kidney demonstrated lower levels of corrective action compared to the spleen and heart. The irradiation suppressed levels of various metabolites recovered in a drug dose-dependent manner in the heart as a result of amifostine treatment. In terms of specific metabolites, levels of 2-arachidonoylglycerol (2-AG), eicosadienoic acid, and N-arachidonoyl-L-alanine levels were lowered by irradiation alone, but appeared to increase with amifostine prophylaxis. The levels of glutamic acid, l-valine, and hypoxanthine were suppressed as well by irradiation, but suppression was only mitigated by amifostine prophylaxis. Opposite trends were noted with phosphatidylserine (PS (18:0/20:4)) and L-arginine; namely, these metabolites were initially elevated after irradiation alone, but with amifostine prophylaxis, the ionizing radiation-induced responses were dampened in a dose-dependent manner. The levels of carbocyclic thromboxane A2A, an inflammatory mediator, was significantly elevated after irradiation and, again with amifostine prophylaxis, the ionizing radiation-induced response was dampened. The kidney showed minimal dysregulation; the levels of arachidonic acid, citric acid, and eicosapentaenoic acid were reduced after irradiation at day 4, and were elevated at day 9. In both cases, amifostine prophylaxis appeared to promote more normal (a return to steady state) levels of these metabolites. Amifostine administration to mice did not stimulate major metabolic changes in sham-irradiated mice.
Such findings may prove valuable in identifying biomarkers for efficacy of radiation countermeasures under development and for approval from regulatory agencies, as the identification of biomarkers is a critical step for drug-dose conversion from animal models to humans for countermeasure development [Citation1,Citation8,Citation79,Citation111].
4. Challenges and limitation
Identifying and validating biomarkers for radiation injury and radiation medical countermeasure efficacy for drug-dose conversion from animals to humans is complex and poses multiple analytical and clinical challenges that are a barrier toward successful translation of these biomarkers for clinical use. The sample collection, processing, and storage conditions can significantly impact downstream metabolomics analyses and biomarkers detected, and should be accounted for in the study design. The selection of analytical platforms, data preprocessing, and computational tools used for data analysis are critical toward discrimination between samples collected from the radiation exposed and non-exposed study subjects as well as from countermeasure-treated and untreated subjects [Citation9]. Future studies will further elucidate which metabolites and lipids are suitable candidate biomarkers for prognostic and predictive purposes for radiation injury and suitable for countermeasure efficacy determination. The only reliable tissue injury-specific biomarker identified in the area of radiation biology through metabolomics is citrulline. In any radiological/nuclear event, burn (thermal), wound, trauma, and chemical injuries in combination with radiation exposure may significantly change the result of metabolic profiles. Metabolomic changes under the situation of combined injuries will demonstrate changes as a result of polytrauma. Furthermore, the use of a metabolomics only approach to determine radiation-dose uniformity, total-body versus partial-body radiation exposure, may not yield precise results and may have to be combined with an orthogonal method to improve predictive accuracy. Finally, the fields of bioinformatics is moving at a fast pace for the analysis of metabolomics data; as such, one needs to be cognizant of the latest cutting edge computational tools that could be used to reanalyze these data.
5. Conclusion
The emergency response plan for a mass radiological/nuclear incidence requires early and efficient assessments of potential radiation exposures to populations at risk. In part, research, development and fielding of sensitive, accurate and rapidly developing assessment tools, e.g. clinically relevant biomarkers, are essential components of such an emergency plan’s effectiveness and utility, as well as for its successful promulgation. Further, appropriately tested and validated clinical biomarkers would help establish targeted and individualized treatment options for victims exposed to radiation. Significant progress has been made with LC-MS as well as NMR-based metabolomic approaches using various animal models exposed to radiation, and also from radiation-exposed patients with various malignancies. A large number of studies have demonstrated metabolomic perturbations compared with samples collected from sham-irradiated or pre versus post-irradiated animals in patterns that are dependent on radiation quality, dose, and time post-exposure [Citation9,Citation14,Citation41].
Metabolites most frequently detected and validated post-irradiation in animal models of ARS and DEARE are carnitine, citric acid, citrulline, creatine, creatinine, hypoxanthine, taurine, threonine, uric acid, and xanthine. The majority of past studies have demonstrated consistent perturbations in citric acid, creatine, citrulline, and uric acid [Citation9,Citation14,Citation41]. These metabolites are promising biomarker candidates due to their time and dose-dependent responses shown in various models of radiation injury. However, additional research is required in order to demonstrate ‘radiation specificity.’ Similarly, some lipids (PC (36:1) and sphingolipid ceramide) have also shown promise as potential radiation-related biomarkers, but again, additional work is required in order to demonstrate and validate these biomarkers as being definitive of ionizing radiation-exposures/injuries [Citation9]. Nevertheless, recent studies on the role of ceramide in ionizing radiation-related cell and tissue injuries may be an appropriate counterpoint to the above comments; in fact, ionizing radiation-induced ceramide may be an appropriate therapeutic target, as there are reports demonstrating the role of ceramide in radiation injury and the use of its antibody as a radiation countermeasure [Citation21,Citation112–114]. Acid sphingomyelinase-mediated ceramide generation regulates apoptosis in response to ionizing radiation in GI endothelial cells. Transmembrane signaling of this apoptotic program is coordinated by clustering of plasma membrane rafts into ceramide-rich platforms (CRP). CRP generation plays a fundamental role in radiation-induced GI syndrome. Anti-ceramide antibodies inhibit ceramide-mediated endothelial apoptosis by providing radioprotection from the lethal radiation-induced GI syndrome in mice.
There is no doubt that metabolomics represents a potentially important and powerful approach to study the changes after irradiation even prior to the onset of clinical symptoms. Improvements in the methods of metabolite identification and associated databases will facilitate, without question, the development of multi-metabolite panels for FDA qualification and for the potential clinical use of the technology in the near future. However, the metabolomics literature rightfully points out the need for standardized protocols for investigation, validation of identified targeted biomarkers, conducting studies with female cohorts to test gender bias, as well as different age cohorts, and the establishment of metabolomic assays that are in compliance with FDA biomarker qualification guidelines.
6. Expert opinion
As stated above, it is difficult to identify a set of biomarkers for radiation injury that would be universally applicable under different scenarios. Several biomarkers have been qualified by the US FDA, but none have been qualified specifically for radiation injury. A number of significant, translational barriers exist including, but most certainly not limited to: (i) a primary, ethical barrier of limiting ionizing radiation exposures of individuals to injurious radiation for the sake of testing, and (ii) the extended process of identifying/validating biomarkers in compliance with the FDA’s Animal Rule (assessment of biomarkers in a small animal model followed by a large animal model). These compliance barriers have impeded the pace of countermeasure development and have extended the time necessary for approval for clinical use. In addition, lack of availability of well annotated and stringently collected and stored biospecimens from past radiological/nuclear attacks or accidents has led to slow progress in the field. Moreover, such studies need to be conducted over a reasonable time frame after radiation exposure. The majority of the reported radiation exposure studies have concentrated on total-body exposures, while fewer studies use partial-body exposures, with or without combined injuries (wounding plus irradiation, hemorrhage plus irradiation, burn plus irradiation) that are more probable scenarios. Combined injury (ionizing radiation plus burns or infections, etc.) clearly complicates and alters biodosimetric assessments and generally carries a higher mortality rate than radiation exposure alone [Citation115–117]. Thus, assessment of biomarkers in partial-body exposures and combined injury scenarios need to be investigated as well. It will be ideal to study the biomarkers for total-body, partial-body, and combined injury scenarios that include pediatric/juvenile as well as aged/geriatric animal models to represent the general population.
Without doubt, radiation metabolomics/lipidomics is a research area that holds promise, especially in terms of its noninvasive or minimally invasive nature and its capacity to measure rather subtle, ionizing radiation-induced fluctuations of the body’s metabolism networks. In the event of mass casualty incidents, clinical biomarkers that can help identify and segregate exposed from unexposed individuals are of critical importance. Although early physical symptoms (prodromal responses) of acute radiation syndrome have been identified and used for triage purposes, they are notoriously insensitive to lower intensities of ionizing radiation (< 1 Gy) that are still quite injurious to the victim’s health and well-being. Further, there is a latent period between the end of prodromal symptoms and the onset of physiological complications. A metabolomics approach offers the possibility of identifying and quantifying metabolites which comprise the metabolome, an essential network that directly reflects the physiological status of an individual. Recent advances in high-resolution chromatography and mass spectrometry have enabled researchers to use a metabolomics approach to discover and develop predictive biomarkers for radiation dosimetry; these recent advances have been comprehensively reviewed [Citation13]. Development of specific bio-signatures that can be evaluated in blood, urine, or feces offers the distinct advantage of screening large populations using minimally invasive procedures. Furthermore, the capacity to assess the severity of ionizing radiation injuries is critical for the clinical management of the at-risk population, as well as ruling out the possibility of ionizing radiation exposures for the ‘worried well.’ It is imperative that the biomarkers are stable over time after radiation exposure and allow for risk and exposure assessments to be established early, even before the appearance of clinical symptoms. However, in order for ionizing radiation-specific biomarkers to be fully established and ‘fielded,’ systematic research and development will be required and aimed at identifying, characterizing, and validating new, predictive biomarkers of acute exposures. This additional R&D effort for radiation-specific biomarkers would undoubtedly facilitate clinical management processes as related to the types and timing of therapeutic interventions, and would in turn, save lives.
In brief, several promising metabolomes and lipidomes have been identified for radiation injury and for the efficacy of radiation countermeasures. These biomarkers are at early stages of development and need further examination and validation using various animal models and humans. Improvements in metabolite identification databases will facilitate the development of multi-metabolite panels for clinical use.
Article highlights
Radiation countermeasure development includes identification of radiation injury biomarkers as well as biomarkers for radiation countermeasure efficacy.
Biomarkers are important for countermeasure dose conversion from animals to humans.
Currently, there is no fully FDA-‘qualified’ biomarker of radiation injury.
Studies have demonstrated that metabolomic markers in easily accessible biofluid samples may prove useful for the identification and validation of a radiation exposure biomarker.
Several metabolomes and lipidomes are being carefully investigated as potential biomarkers for radiation injury.
Recent metabolomic studies with radiation countermeasures using murine and NHP models are promising in terms of identifying agent-specific biomarkers.
Declaration of interest
The authors have no relevant affiliations or financial involvement with any organization or entity with a financial interest in or financial conflict with the subject matter or materials discussed in the manuscript. This includes employment, consultancies, honoraria, stock ownership or options, expert testimony, grants or patents received or pending, or royalties.
Reviewer disclosures
Peer reviewers on this manuscript have no relevant financial or other relationships to disclose.
Acknowledgments
The opinions or assertions expressed herein are those of the authors and do not necessarily represent the opinions or policies of the Uniformed Services University of the Health Sciences, the Department of Defense, or the U.S. government. The authors are thankful to Ms. Alana Carpenter for editing the manuscript. The authors apologize to those having contributed substantially to the topics discussed herein that we were unable to cite due to space constraints.
Additional information
Funding
References
- Singh VK, Newman VL, Romaine PL, et al., Use of biomarkers for assessing radiation injury and efficacy of countermeasures. Expert Rev Mol Diagn. 16(1): 65–81. 2016. .
- Singh VK, Pollard HB. Ionizing radiation-induced altered microRNA expression as biomarkers for assessing acute radiation injury. Expert Rev Mol Diagn. 2017;17(10):871–874.
- Hall EJ, Giaccia AJ. Radiobiology for the Radiobiologist. 7th ed. Philadelphia, PA: Lippincott Williams and Wilkins; 2012.
- Bushberg JT, Kroger LA, Hartman MB, et al. Nuclear/radiological terrorism: emergency department management of radiation casualties. J Emerg Med. 2007;32(1):71–85.
- Farese AM, MacVittie TJ. Filgrastim for the treatment of hematopoietic acute radiation syndrome. Drugs Today (Barc). 2015;51:537–548.
- Zhong Y, Pouliot M, Downey AM, et al. Efficacy of delayed administration of sargramostim up to 120 hours post exposure in a nonhuman primate total body radiation model. Int J Radiat Biol. 2020:1–17. 10.1080/09553002.2019.1673499. in press.
- Hankey KG, Farese AM, Blaauw EC, et al. Pegfilgrastim improves survival of lethally irradiated nonhuman primates. Radiat Res. 2015;183(6):643–655.
- Singh VK, Simas M, Pollard H. Biomarkers for acute radiation syndrome: challenges for developing radiation countermeasures following animal rule. Expert Rev Mol Diagn. 2018;18(11):921–924.
- Pannkuk EL, Fornace AJ Jr., Laiakis EC. Metabolomic applications in radiation biodosimetry: exploring radiation effects through small molecules. Int J Radiat Biol. 2017;93(10):1151–1176.
- Jones JW, Scott AJ, Tudor G, et al. Identification and quantitation of biomarkers for radiation-induced injury via mass spectrometry. Health Phys. 2014;106(1):106–119.
- Gatto L, Hansen KD, Hoopmann MR, et al. Testing and validation of computational methods for mass spectrometry. J Proteome Res. 2016;15(3):809–814.
- Rustam YH, Reid GE. Analytical challenges and recent advances in mass spectrometry based lipidomics. Anal Chem. 2018;90(1):374–397.
- Coy SL, Cheema AK, Tyburski JB, et al., Radiation metabolomics and its potential in biodosimetry. Int J Radiat Biol. 87(8): 802–823. 2011. .
- Vicente E, Vujaskovic Z, Jackson IL. A systematic review of metabolomic and lipidomic candidates for biomarkers in radiation injury. Metabolites. 2020;10(6):259.
- Menon SS, Uppal M, Randhawa S, et al. Radiation metabolomics: current status and future directions. Front Oncol. 2016;6:20.
- Laiakis EC, Canadell MP, Grilj V, et al. Serum lipidomic analysis from mixed neutron/X-ray radiation fields reveals a hyperlipidemic and pro-inflammatory phenotype. Sci Rep. 2019;9(1):4539.
- Laiakis EC, Wang YW, Young EF, et al. Metabolic Dysregulation after Neutron Exposures Expected from an Improvised Nuclear Device. Radiat Res. 2017;188(1):21–34.
- Quehenberger O, Armando AM, Brown AH, et al. Lipidomics reveals a remarkable diversity of lipids in human plasma. J Lipid Res. 2010;51(11):3299–3305.
- Jelonek K, Pietrowska M, Ros M, et al. Radiation-induced changes in serum lipidome of head and neck cancer patients. Int J Mol Sci. 2014;15(4):6609–6624.
- Lin X, Fuks Z, Kolesnick R. Ceramide mediates radiation-induced death of endothelium. Crit Care Med. 2000;28(Supplement):N87–93.
- Kolesnick R, Fuks Z. Radiation and ceramide-induced apoptosis. Oncogene. 2003;22(37):5897–5906.
- Jones JW, Carter CL, Li F, et al. Ultraperformance convergence chromatography-high resolution tandem mass spectrometry for lipid biomarker profiling and identification. Biomed Chromatogr. 2017;39(3):e3822.
- Pannkuk EL, Laiakis EC, Authier S, et al. Targeted metabolomics of nonhuman primate serum after exposure to ionizing radiation: potential tools for high-throughput biodosimetry. RSC Adv. 2016;6(56):51192–51202.
- Goudarzi M, Weber WM, Chung J, et al. Serum dyslipidemia is induced by internal exposure to Strontium-90 in Mice, Lipidomic profiling using a data-independent liquid Chromatography–Mass Spectrometry Approach. J Proteome Res. 2015;14(9):4039–4049.
- Carter CL, Jones JW, Farese AM, et al. Lipidomic dysregulation within the lung parenchyma following whole-thorax lung irradiation: markers of injury, inflammation and fibrosis detected by MALDI-MSI. Sci Rep. 2017;7(1):10343.
- Pannkuk EL, Laiakis EC, Mak TD, et al. A lipidomic and metabolomic serum signature from nonhuman primates exposed to ionizing radiation. Metabolomics. 2016;12(5):80.
- Pannkuk EL, Laiakis EC, Singh VK, et al., Lipidomic signatures of nonhuman primates with radiation-induced hematopoietic syndrome. Sci Rep. 7(1): 9777. 2017. .
- Cheema AK, Hinzman CP, Mehta KY, et al., Plasma derived exosomal biomarkers of exposure to ionizing radiation in nonhuman primates. Int J Mol Sci. 19(11): 3427. 2018.
- Khan AR, Rana P, Devi MM, et al. Nuclear magnetic resonance spectroscopy-based metabonomic investigation of biochemical effects in serum of γ-irradiated mice. Int J Radiat Biol. 2011;87(1):91–97.
- Kurland IJ, Broin P, Golden A, et al. Integrative Metabolic Signatures for Hepatic Radiation Injury. PloS One. 2015;89(6):e0124795.
- Pannkuk EL, Laiakis EC, Fornace AJ Jr., et al., A metabolomic serum signature from nonhuman primates treated with a radiation countermeasure, gamma-tocotrienol, and exposed to ionizing radiation. Health Phys. 115(1): 3–11. 2018. .
- Pannkuk EL, Laiakis EC, Authier S, et al. Gas chromatography/mass spectrometry metabolomics of urine and serum from nonhuman primates exposed to ionizing radiation: impacts on the tricarboxylic acid cycle and protein metabolism. J Proteome Res. 2017;16(5):2091–2100.
- Bujold K, Hauer-Jensen M, Donini O, et al. Citrulline as a biomarker for gastrointestinal-acute radiation syndrome: species differences and experimental condition effects. Radiat Res. 2016;186(1):71–78.
- Wang J, Shao L, Hendrickson HP, et al. Total body irradiation in the “hematopoietic” dose range induces substantial intestinal injury in non-human primates. Radiat Res. 2015;184(5):545–553.
- Jang WG, Park JY, Lee J, et al. Investigation of relative metabolic changes in the organs and plasma of rats exposed to X-ray radiation using HR-MAS1 HNMR and solution 1HNMR. NMR Biomed. 2016;29(4):507–518.
- Cheema AK, Li Y, Girgis M, et al., Metabolomic studies in tissues of mice treated with amifostine and exposed to gamma-radiation. Sci Rep. 9(1): 15701. 2019. .
- Cheema AK, Suman S, Kaur P, et al. Long-term differential changes in mouse intestinal metabolomics after γ and heavy ion radiation exposure. PloS One. 2014;9(1):87079.
- Rotolo JA, Fong CS, Bodo S, et al. Anti-ceramide single-chain variable fragment mitigates radiation GI syndrome mortality independent of DNA repair. JCI Insight. 2021;6(8). 10.1172/jci.insight.145380.
- Wang Z, Zhao Y. Gut microbiota derived metabolites in cardiovascular health and disease. Protein Cell. 2018;9(5):416–431.
- Comai S, Bertazzo A, Brughera M, et al. Tryptophan in health and disease. Adv Clin Chem. 2020;95:165–218.
- Satyamitra MM, Cassatt DR, Hollingsworth BA, et al., Metabolomics in Radiation Biodosimetry: current Approaches and Advances. Metabolites. 10(8): 328. 2020. .
- Dorr H, Meineke V. Acute radiation syndrome caused by accidental radiation exposure - therapeutic principles. BMC Med. 2011;9(1):126.
- Gourmelon P, Benderitter M, Bertho JM, et al. European consensus on the medical management of acute radiation syndrome and analysis of the radiation accidents in Belgium and Senegal. Health Phys. 2010;98(6):825–832.
- McCann DGC. Radiation poisoning: current concepts in the acute radiation syndrome. Am J Clin Med. 2006;3:13–21.
- Peter RU, Gottlober P, Nadeshina N, et al. Radiation lentigo. A distinct cutaneous lesion after accidental radiation exposure. Arch Dermatol. 1997;133(2):209–211.
- Peter RU, Gottlober P. Management of cutaneous radiation injuries: diagnostic and therapeutic principles of the cutaneous radiation syndrome. Mil Med. 2002;167(suppl_1):110–112.
- Peter RU, Braun-Falco O, Birioukov A, et al. Chronic cutaneous damage after accidental exposure to ionizing radiation: the Chernobyl experience. J Am Acad Dermatol. 1994;30(5):719–723.
- Dainiak N, Gent RN, Carr Z, et al. Literature review and global consensus on management of acute radiation syndrome affecting nonhematopoietic organ systems. Disaster Med Public Health Prep. 2011;184(3):183–201.
- Fliedner TM, Dorr DH, Meineke V. Multi-organ involvement as a pathogenetic principle of the radiation syndromes: a study involving 110 case histories documented in SEARCH and classified as the bases of haematopoietic indicators of effect. British Journal of Radiology Suppl. 2005;Supplement_27(1):1–8.
- Hill RP. Radiation effects on the respiratory system. British Journal of Radiology Suppl. 2005;27(1):75–81.
- Moulder JE, Cohen EP. Radiation-induced multi-organ involvement and failure: the contribution of radiation effects on the renal system. British Journal of Radiology Suppl. 2005;27(1):82–88.
- Stone HB, Moulder JE, Coleman CN, et al. Models for evaluating agents intended for the Prophylaxis, mitigation and treatment of radiation injuries report of an NCI workshop, December 3–4, 2003. Radiat Res. 2004;162(6):711–728.
- Singh VK, Seed TM. Pharmacological management of ionizing radiation injuries: current and prospective agents and targeted organ systems. Expert Opin Pharmacother. 2020;21(3):317–337.
- MacVittie TJ. The MCART consortium animal model series: MCART animal model refinement and MCM development: defining organ dose, organ-specific tissue imaging, model validation and the natural history between the acute radiation syndrome (ARS) and the delayed effects of acute radiation exposure (DEARE). Health Phys. 2015;109(5):335–341.
- Molls M, Budach V, Bamberg M. Total body irradiation: the lung as critical organ. Strahlenther Onkol. 1986;162(4):226–232.
- MacVittie TJ, Farese AM, Kane MAARS. DEARE, and multiple-organ injury: a strategic and tactical approach to link radiation effects, animal models, medical countermeasures, and biomarker development to predict clinical outcome. Health Phys. 2019;116(4):453.
- MacVittie TJ, Farese AM, Parker GA, et al. The time course of radiation-induced lung injury in a nonhuman primate model of partial-body irradiation with minimal bone marrow sparing: clinical and radiographic evidence and the effect of neupogen administration. Health Phys. 2019;10(3):328–382.
- Seed TM, Blakely WF, Knudson GB, et al. International conference on low-level radiation injury and medical countermeasures. Mil Med. 2002;167:1–143.
- Seed TM, Fry SA, Neta R, et al. Prevention and treatments: summary statement. Mil Med. 2002;98(suppl_1):87–93.
- Ralston-Hooper K, Jannasch A, Adamec J, et al. The use of two-dimensional gas chromatography-time-of-flight mass spectrometry (GCxGC-TOF-MS) for metabolomic analysis of polar metabolites. Methods Mol Biol. 2011;708:205–211.
- Forster J, Famili I, Fu P, et al. Genome-scale reconstruction of the Saccharomyces cerevisiae metabolic network. Genome Res. 2003;13(2):244–253.
- Fiehn O. Metabolomics–the link between genotypes and phenotypes. Plant Mol Biol. 2002;48(1/2):155–171.
- Pannkuk EL, Laiakis EC, Girgis M, et al. Temporal effects on radiation responses in nonhuman primates: identification of biofluid small molecule signatures by gas chromatography–mass spectrometry metabolomics. Metabolites. 2019;9(5):98.
- Horning EC, Horning MG. Metabolic profiles: chromatographic methods for isolation and characterization of a variety of metabolites in man. Methods in Medical Research. 1970;12:369–371.
- Markley JL, Bruschweiler R, Edison AS, et al. The future of NMR-based metabolomics. Curr Opin Biotechnol. 2017;43:34–40.
- Cheng J, Lan W, Zheng G, et al. Metabolomics: a high-throughput platform for metabolite profile exploration. Methods Mol Biol. 2018;1754:265–292.
- Clendinen CS, Monge ME, Fernandez FM. Ambient mass spectrometry in metabolomics. Analyst. 2017;142(17):3101–3117.
- Fuhrer T, Zamboni N. High-throughput discovery metabolomics. Curr Opin Biotechnol. 2015;31:73–78.
- Taraboletti A, Goudarzi M, Kabir A, et al. Fabric Phase Sorptive Extraction—A metabolomic preprocessing approach for ionizing radiation exposure assessment. J Proteome Res. 2019;18(8):3020–3031.
- Laiakis EC. Metabolomic applications in radiation biodosimetry. Methods Mol Biol. 2019;1978:391–402.
- Singh VK, Seed TM. An update on sargramostim for treatment of acute radiation syndrome. Drugs Today (Barc). 2018;54(11):679–693.
- Gale RP, Armitage JO. Use of molecularly-cloned haematopoietic growth factors in persons exposed to acute high-dose, high-dose rate whole-body ionizing radiations. Blood Rev. 2021;45:100690.
- Singh VK, Newman VL, Seed TM. Colony-stimulating factors for the treatment of the hematopoietic component of the acute radiation syndrome (H-ARS): a review. Cytokine. 2015;71(1):22–37.
- Amgen Inc. Neupogen (filgrastim) injection for subcutaneous or intravenous use. 2015. Available at: http://pi.amgen.com/united_states/neupogen/neupogen_pi_hcp_english.pdf [Last accessed 2015 Apr 02]
- Amgen Inc. Neulasta (pegfilgrastim) injection for subcutaneous use. 2015. Available at: http://pi.amgen.com/united_states/neulasta/neulasta_pi_hcp_english.pdf [Last accessed 2015 Nov 19]
- Sanofi-Aventis U.S. LLC. LEUKINE® (sargramostim) for injection, for subcutaneous or intravenous use. 2018. Available at: https://www.accessdata.fda.gov/drugsatfda_docs/label/2018/103362s5240lbl.pdf?utm_campaign=20180329%20MCMi&utm_medium=email&utm_source=Eloqua [Last accessed 2018 Apr 01]
- Farese AM, Cohen MV, Katz BP, et al. Filgrastim improves survival in lethally irradiated nonhuman primates. Radiat Res. 2013;179(1):89–100.
- Singh VK, Seed TM, Olabisi AO. Drug discovery strategies for acute radiation syndrome. Expert Opin Drug Discov. 2019;14(7):701–715.
- U.S. Food and Drug Administration. Guidance for industry: product developoment under the Animal Rule. 2015. Available at: http://www.fda.gov/downloads/Drugs/GuidanceComplianceRegulatoryInformation/Guidances/UCM399217.pdf [Last accessed 2016 Jul 5].
- Nair AB, Jacob S. A simple practice guide for dose conversion between animals and human. J Basic Clin Pharm. 2016;54(2):27–31.
- U.S. Food and Drug Administration. More about biomarkers & qualification. 2018. Available at: https://www.fda.gov/Drugs/DevelopmentApprovalProcess/DrugDevelopmentToolsQualificationProgram/BiomarkerQualificationProgram/ucm535408.htm [Last accessed 2018 May 18]
- Aebersold P. FDA experience with medical countermeasures under the animal rule. Adv Prev Med. 2012;2012:507571.
- Administration USFaD. CDER Drug and biologics animal rule approvals. 2020. Available at: https://www.fda.gov/media/107839/download [Last accessed 2010 Oct 11]
- Patterson AD, Lanz C, Gonzalez FJ, et al. The role of mass spectrometry-based metabolomics in medical countermeasures against radiation. Mass Spectrom Rev. 2010;29(3):503–521.
- Fendler W, Malachowska B, Meghani K, et al. Evolutionarily conserved serum microRNAs predict radiation-induced fatality in nonhuman primates. Sci Transl Med. 2017;9:eaal2408.
- Ghosh SP, Pathak R, Kumar P, et al. Gamma-tocotrienol modulates radiation-induced microRNA expression in mouse spleen. Radiat Res. 2016;185(5):485–495.
- Singh VK, Seed TM. BIO 300: a promising radiation countermeasure under advanced development for acute radiation syndrome and the delayed effects of acute radiation exposure. Expert Opin Investig Drugs. 2020;29(5):429–441.
- Jackson IL, Zodda A, Gurung G, et al. BIO 300, a nanosuspension of genistein, mitigates pneumonitis/fibrosis following high-dose radiation exposure in the C57L/J murine model. Br J Pharmacol. 2017;174(24):4738–4750.
- Jones JW, Jackson IL, Vujaskovic Z, et al., Targeted metabolomics identifies pharmacodynamic biomarkers for BIO 300 mitigation of radiation-induced lung injury. Pharm Res. 12(12): 369–371. 2017. .
- Niaudet C, Bonnaud S, Guillonneau M, et al. Plasma membrane reorganization links acid sphingomyelinase/ceramide to p38 MAPK pathways in endothelial cells apoptosis. Cell Signal. 2017;33:10–21.
- Corre I, Guillonneau M, Paris F. Membrane signaling induced by high doses of ionizing radiation in the endothelial compartment. Relevance in radiation toxicity. Int J Mol Sci. 2013;14(11):22678–22696.
- Cheema AK, Mehta KY, Santiago PT, et al., Pharmacokinetic and metabolomic studies with BIO 300, a nanosuspension of genistein, in a nonhuman primate model. Int J Mol Sci. 20(5): 1231. 2019. .
- Sen CK, Khanna S, Roy S. Tocotrienols: vitamin E beyond tocopherols. Life Sci. 2006;78(18):2088–2098.
- Singh VK, Beattie LA, Seed TM. Vitamin E: tocopherols and tocotrienols as potential radiation countermeasures. J Radiat Res. 2013;54(6):973–988.
- Singh VK, Hauer-Jensen M. γ-Tocotrienol as a Promising Countermeasure for Acute Radiation Syndrome: current Status. Int J Mol Sci. 2016;17(5):e663.
- Singh VK, Kulkarni S, Fatanmi OO, et al. Radioprotective efficacy of gamma-tocotrienol in nonhuman primates. Radiat Res. 2016;185(3):285–298.
- Cheema AK, Mehta KY, Fatanmi OO, et al., A Metabolomic and lipidomic serum signature from nonhuman primates administered with a promising radiation countermeasure, gamma-tocotrienol. Int J Mol Sci. 185(1): 79. 2017. .
- Singh VK, Seed TM. The efficacy and safety of amifostine for the acute radiation syndrome. Expert Opin Drug Saf. 2019;18(11):1077–1090.
- Carnes BA, Grdina DJ. In Vivo Protection by the Aminothiol WR-2721 against Neutron-induced Carcinogenesis. Int J Radiat Biol. 1992;61(5):567–576.
- Rasey JS, Spence AM, Badger CC, et al. Specific protection of different normal tissues. Pharmacol Ther. 1988;39(1–3):33–43.
- Weiss JF. Pharmacologic approaches to protection against radiation-induced lethality and other damage. Environ Health Perspect. 1997;105(Suppl 6):1473–1478.
- Seed TM, Inal CE, Singh VK. Radioprotection of hematopoietic progenitors by low dose amifostine prophylaxis. Int J Radiat Biol. 2014;90(7):594–604.
- Cumberland Pharmaceuticals Inc. ETHYOL- amifostine injection, powder, lyophilized, for solution. 2017. Available at: https://www.accessdata.fda.gov/drugsatfda_docs/label/2017/020221s033lbl.pdf [Last accessed 2020 Feb 12]
- Brizel DM, Wasserman TH, Henke M, et al. Phase III randomized trial of amifostine as a radioprotector in head and neck cancer. J Clin Oncol. 2000;18(19):3339–3345.
- Schuchter LM. Exploration of platinum-based dose-intensive chemotherapy strategies with amifostine (Ethyol). Eur J Cancer. 1996;32(Suppl 4):S40–2.
- Ali BH, Al Moundhri MS. Agents ameliorating or augmenting the nephrotoxicity of cisplatin and other platinum compounds: a review of some recent research. Food Chem Toxicol. 2006;44(8):1173–1183.
- Chirino YI, Pedraza-Chaverri J. Role of oxidative and nitrosative stress in cisplatin-induced nephrotoxicity. Experimental and toxicologic pathology: official journal of the Gesellschaft fur Toxikologische Pathologie. 2009;61(3):223–242.
- Seed TM. Radiation protectants: current status and future prospects. Health Phys. 2005;89(5):531–545.
- Singh VK, Fatanmi OO, Wise SY, et al. The potentiation of the radioprotective efficacy of two medical countermeasures, gamma-tocotrienol and amifostine, by a combination prophylactic modality. Radiat Prot Dosimetry. 2016;172(1–3):302–310.
- Cheema AK, Li Y, Girgis M, et al., Alterations in tissue metabolite profiles with amifostine-prophylaxed mice exposed to gamma radiation. Metabolites. 10(5): 211. 2020. .
- Singh VK, Santiago PT, Simas M, et al. Acute radiation syndrome: an update on biomarkers for radiation Injury. Journal of Radiation and Cancer Research. 2018;9(4):132–146.
- Rotolo J, Stancevic B, Zhang J, et al. Anti-ceramide antibody prevents the radiation gastrointestinal syndrome in mice. J Clin Invest. 2012;122(5):1786–1790.
- Singh VK, Fuller J, Rotolo J, et al. Anti-ceramide antibody as treatment for the acute radiation gastrointestinal syndrome. 3rd International Conference on the Molecular Medicine of Sphingolipids. French Lick Resort, French Lick, Indiana, USA, 2016
- Singh VK, Fuller J, Rotolo J, et al. Anti-ceramide antibody as treatment for the acute radiation gastrointestinal syndrome. Conference on normal tissue radiation effects and countermeasures. Winthrop Rockfeller Institute, University of Arkansas Medical Sciences, Little Rock, AR, USA, 2018
- Kiang JG, Jiao W, Cary LH, et al. Wound trauma increases radiation-induced mortality by activation of iNOS pathway and elevation of cytokine concentrations and bacterial infection. Radiat Res. 2010;173(3):319–332.
- DiCarlo AL, Maher C, Hick JL, et al. Radiation injury after a nuclear detonation: medical consequences and the need for scarce resources allocation. Disaster Med Public Health Prep. 2011;5(Suppl S1):S32–44.
- DiCarlo AL, Ramakrishnan N, Hatchett RJ. Radiation combined injury: overview of NIAID research. Health Phys. 2010;98(6):863–867.