Abstract
The cholinesterases have been investigated in terms of the effects of methanol and ethanol on substrate and carbamate turnover, and on their phosphorylation. It was found: 1) that at low substrate concentrations the two alcohols inhibit all three tested cholinesterases and that the optimum activities are shifted towards higher substrate concentrations, but with a weak effect on horse butyrylcholinesterase; 2) that methanol slows down carbamoylation by eserine and does not influence decarbamoylation of vertebrate and insect acetylcholinesterase and 3) that ethanol decreases the rate of phosphorylation of vertebrate acetylcholinesterase by DFP. Our results are in line with the so-called ‘approach-and-exit’ hypothesis. By hindering the approach of substrate and the exit of products, methanol and ethanol decrease cholinesterase activity at low substrate concentrations and allow for the substrate inhibition only at higher substrate concentrations. Both effects appears to be a consequence of the lower ability of substrate to substitute alcohol rather than water. It also seems that during substrate turnover in the presence of alcohol the transacetylation is negligible.
Introduction
The cholinesterases catalyze the hydrolysis of choline esters in two successive steps: the first is a trans-esterification that is known to involve acylation of a serine residue, and the second is deacylation, which involves a water molecule [Citation1]. In all of the cholinesterases, the gorge-like active site is buried at a depth of some 20 Å within the core of the molecule [Citation2]. Each substrate molecule first occupies a peripheral binding site that comprises several residues at the mouth of the gorge, including a tryptophan and an aspartic acid. The substrate molecule then moves to the bottom of the gorge [Citation3], where the acylation site with the catalytic triad is situated. Here, another tryptophan forms a catalytic anionic site that interacts with the choline moiety via cation-π interactions, and thus accommodates the substrate in an extended conformation. During the nucleophilic attack by an activated serine, a second substrate molecule can become attached to the peripheral site. Additionally, this second substrate molecule can substitute for the choline at the bottom of the active site prior to the deacylation, and then the binding of a third substrate molecule at the mouth of the gorge results in a fully occupied cholinesterase active site [Citation4].
The specific architecture of the active surface of the cholinesterases allows a very high substrate turnover, which also displays atypical kinetic behavior: at low substrate concentrations, the activities exceed the expected values for a Michaelian-type enzyme, and at high substrate concentrations, varying degrees of inhibition can be seen. Homotropic inhibition is a consequence of at least two distinctive events: the binding of a second substrate molecule to the peripheral site hinders the exit of the choline part of the substrate which has just been cleaved [Citation5], while the binding of a substrate molecule to the acylated enzyme within the active-site gorge blocks deacetylation by sterically preventing the access of water and the exit of the acyl part of the first substrate molecule [Citation6]. On the other hand, the binding of a substrate molecule to the peripheral anionic site of the acylated cholinesterase increases the probability of hydrolytic deacylation, by converting an open active-site gorge into a narrow hollow [Citation4].
If an active-site-directed inhibitor is mixed with a cholinesterase and its substrate in a buffer solution, two molecules of inhibitor or substrate, or one of each, can occupy the free or acylated enzyme. At low substrate concentrations, competition between inhibitor and substrate occurs, leading to lower enzymic activity. At high substrate concentrations, however, the relative potency of the inhibitor and substrate for blocking the choline exit and the deacylation determines how the activity is affected. As expected, this situation is additionally complicated as different enzymes and substrates show different rates of the different steps in the reaction [Citation6,Citation7].
In the present study, we have investigated the relationships between the acylation and deacylation steps by comparing the effects of methanol and ethanol on a vertebrate (electric eel), an insect (Drosophila melanogaster) acetylcholinesterase (AChE), and also a vertebrate (horse) butyrylcholinesterase (BChE). It has been suggested that alcohol perturbs the structure of water around hydrophobic areas of cholineterases, causing an instability in the enzyme conformation and subsequently decreasing the activity [Citation8]. However, alcohols may compete with the substrate for the active site, but they could also compete or even help the water molecule when nucleophilically attacking the esteratic bond of the acylated enzyme. We have tested this hypothesis by following substrate hydrolysis in the presence of methanol and ethanol, and by studying the effect of methanol on carbamate turnover and ethanol on the time-course of irreversible inhibition by an organophosphate. The possible transacetylation in the presence of ethanol was also determined by measuring the presence of ethyl acetate in samples of pre-incubated reaction mixture, using time-resolved gas chromatography.
Materials and methods
Materials
Enzyme sources
Electric eel AChE and horse BChE were purchased from Sigma Chemicals (St Louis, MO, USA). Enzyme concentrations were determined by so-called pseudo-irreversible titration, according to the procedure described previously [Citation15], with the calibration factors of 6.6 determined for 1 nM AChE and 0.4
for 1 nM BChE. Truncated cDNA encoding the soluble wild-type AChE of D. melanogaster was expressed using a baculovirus system. Secreted AChE was purified and stabilized with 1 mg/mL BSA, as previously reported [Citation15,Citation16]. The concentration of AChE was determined by active-site titration using 7-(methylethoxyphosphinyloxy)-1-methylquinolinium iodide, a high-affinity phosphorylating agent [Citation17].
Chemicals
ATCh, BTCh and 2,2-bisdithionitrobenzoic acid (DTNB, Ellman's reagent) were purchased from Sigma (St Louis, MO, USA). Eserine was from BDH Biochemicals and DFP from Sigma. All other reagents were of analytical grade and purchased from a local supplier of chemical reagents.
Kinetic measurements
Substrate hydrolysis
The initial rates of acetylthiocholine (ATCh) and butyrylthiocholine (BTCh) hydrolysis by AChE and BChE were measured photometrically at 412 nm, as described by Ellman et al. [Citation9]. The initial rate measurements were performed at substrate concentrations from 20 microM to 50 mM in the absence and the presence of 2.5% to 20% methanol and ethanol concentrations. The activities were followed for 1 min after the addition of the cholinesterases to the mixture, and the spontaneous hydrolysis of the substrate was subtracted. Each measurement was repeated from two to five times. The experiments were carried out at 25°C in 25 mM phosphate buffer, pH 7.0.
Eserine hydrolysis
The activities of the residual free cholinesterase portions after various incubation times with eserine were measured as follows. The cholinesterase stock solutions (10 nM) were incubated with eserine concentrations from 30 nM to 500 nM in a buffered incubation mixture in a total volume of 0.5 ml. After time intervals of zero to 180 min, aliquots of the incubation mixtures were tested for residual cholinesterase activity using the Ellman procedure [Citation9]. The measurements were repeated in 15% methanol. All of these measurements were carried out in triplicate.
Enzyme phosphorylation with diisopropyl fluorophosphate (DFP)
After mixing the electric eel AChE stock solution (10 nM) with DFP (0.1 mM), the time-course of activity of the residual free electric eel AChE portion was followed essentially as for eserine (see above). The loss of activity was followed in the absence and in the presence of 10% ethanol. These measurements were carried out in quadruplicate.
Semiquantitative analysis for the presence of ethyl acetate by gas chromatography
To test for the appearance of ethyl acetate during the enzymatic hydrolysis of actylcholine by horse BChE, head space gas chromatography with FID was performed. Here, equal volumes of BChE (100 pM), the substrate acetylcholine (2 mM) and ethanol (20 vol%) were mixed together at room temperature (24°C). After time intervals from zero to 120 min, aliqots were analyzed for ethanol and ethyl acetate by a Hewlett-Packard HP 7694 Headspace Sampler HP 7694 (equilibration temperature 50°C, equilibration time 5 min), HP 6890 Series GC-System with packed column 0.2% Carbowax 1500 on Carbopack CAW, 60/80 mesh and temperature 80°C. For chromatographic tests we deliberately chose BChE because it is stable at 50°C [Citation10]. Chromatograms of control solutions were carried out to allow the identification of reactants and products.
Kinetic analysis
Substrate hydrolysis
The characteristic kinetic parameters for the hydrolysis of their substrates by different cholinesterases in the absence and presence of methanol and ethanol were determined using the kinetic model recently developed for the interpretation of substrate hydrolysis and inhibition in D. melanogaster AChE [Citation6]. Briefly, two molecules of substrate or of inhibitor, or one of each, were allowed to bind simultaneously to the free or acylated enzyme. The alcohols were treated as inhibitors, although deacylation of the cholinesterase in the presence of alcohol molecules at the catalytic anionic site (CAS) was also allowed. The concentration of water was included as a proportional factor in the corresponding deacylation constants to compensate for the presence of high alcohol concentrations (). The modified initial rate equation was derived and implemented using a non-linear regression computer program (see Appendix, A).
Table I. Concentrations and proportional factors for ethanol.
Cholinesterase carbamoylation
Carbamoylation of the cholinesterases was analyzed by a simple two-step reaction scheme, similar to that of Van Slyke and Cullen [Citation11]:
where E represents the free enzyme, C, eserine (carbamate), EC, the carbamoylated enzyme, and P1,2 are eseroline and cabamoylic acid, respectively.
Since the concentrations of the cholinesterases and eserine in the analyses were similar, it is impossible to derive an explicit equation for the time-course of the residual free-enzyme activity. To evaluate both rate constants, the differential equations for this model had to be fitted to the corresponding data, as shown previously [Citation12,Citation13].
Cholinesterase phosphorylation
The phosphorylation of electric eel AChE followed a double-exponential time-course. Several different reaction mechanisms can account for this behavior. The most plausible among these are sequential or parallel mechanisms, with one reversible step and one irreversible step. A similar two step mechanism could also be a consequence of a mixture of DFP and its hydrolytic product in the sample. Indeed, the mixture of two cholinesterase variants would also behave in this way, or a multiple binding of DFP might convert the cholinesterase to a more active isomer. However, in the present study, our interest was to investigate a possible effect of ethanol on the rate of phosphorylation, and not to determine the exact mechanism of double inactivation of electric eel AChE by DFP. Nevertheless, we tested these mechanisms by fitting the corresponding equations to the experimental data of the residual free-enzyme portion after various times of incubation with DFP, both in the absence and presence of ethanol. Here, a large surplus of DFP was used, so that the analysis could be carried out using the equation derived by Alberty and Miller [Citation14] (see Appendix, B). It should be emphasized that proper interpretation of this equation can account for all the above mentioned mechanisms.
Results
The dependence of the cholinesterase initial rates on substrate concentration at various methanol and ethanol concentrations are shown in . Although the effects of these alcohols is quite variable, some common characteristics can be clearly seen. First, at low substrate concentrations, both methanol and ethanol inhibit all three of the cholinesterases tested. Secondly, the effects of methanol and ethanol are very pronounced with vertebrate AChE, with less effects with insect AChE, while horse BChE is affected very little. Thirdly, upon addition of these alcohols, the optimum activities are shifted towards higher substrate concentrations for the AChEs, with no effects on the BChE. Finally, in the presence of methanol, the optimum activities are higher than under control conditions, while for ethanol they can be lower (eel AChE), higher (insect AChE) or unaffected (BChE). The kinetic parameters determined according to the initial rate equation (see Appendix, A) are listed in .
Figure 1 Cholinesterase activities vs. substrate concentrations (pS curves) for electric eel and insect AChE, and horse BChE (as indicated) in the absence and presence of various concentrations of methanol and ethanol (as indicated).
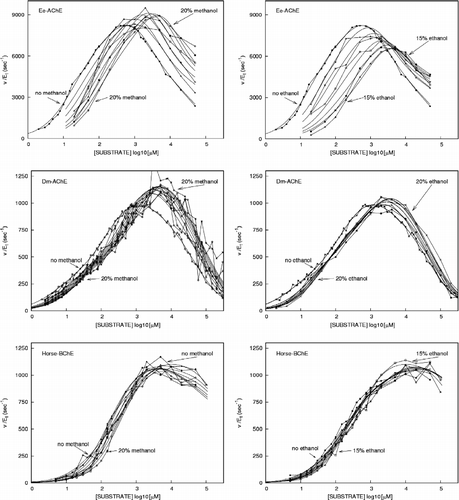
Table II. Kinetic constants for the cholinesterases in the presence of substrate, methanol and ethanol according to the scheme given in Appendix.
The time-course of eserine turnover by the electric eel AChE has been analyzed previously [Citation12,Citation13]. shows the comparison between this time-course in the absence and presence of 15% methanol for the two AChEs (electric eel and insect), which were substantially affected by methanol (see ). Again, in line with the substrate hydrolysis determinations, the effects of methanol are very obvious for vertebrate AChE, and only just perceivable for the insect AChE. Analysis of the shapes of the curves of the time-courses of eserine turnover at approximately equimolar concentrations with the enzyme shows a change over the period investigated. It appears that a sort of protection slows down the first, inhibition portion, and also the second, regeneration portion. A simple two-step analysis, however, reveals that the carbamoylation part (k1 = 2.3+/ − 0.1 × 106 M− 1s− 1) is indeed approximately 4-fold slower in the presence of methanol (k1 = 0.56+/ − 0.02 × 106 M− 1s− 1), while the decarbamoylation step is unchanged (k2water = 0.023+/ − 0.002 s− 1 and k2methanol = 0.020+/ − 0.001 s− 1, respectively). Indeed, slower regeneration is expected, because the entire eserine must be hydrolyzed by the cholinesterase and the slowing in the first part will slow down the whole process.
Figure 2 Time-courses of acetylcholinesterase inhibition by eserine. A. Electric eel AChE. B. Drosophila melanogaster AChE.
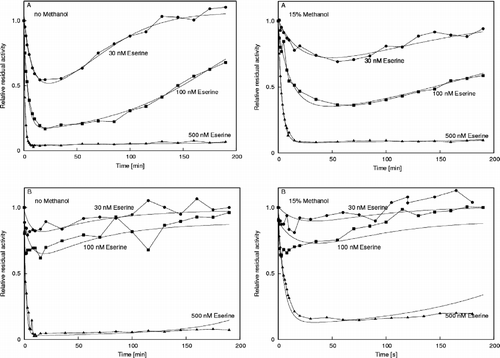
To gain further information on the mechanism of AChE protection by ethanol, we studied the first, acylation part of the AChE reaction by determining the influence of ethanol on electric eel AChE phosphorylation by DFP. The curves in show the time-course of residual AChE activity after various incubation times with 0.1 mM DFP, both in the absence and presence of 10% ethanol. It should be stressed here that both of these curves are double exponential (see inset in ), and that both of the rate constants are approximately 3-fold slower in the presence of 10% ethanol. The overall pseudo first-order rate constant m1 decreased from 0.0145+/ − 0.041 s− 1 to 0.00638+/ − 0.00097 s− 1, and the overall pseudo first-order rate constant m2 decreased from 0.00225+/ − 0.00035 s− 1 to 0.000807+/ − 0.000064 s− 1.
Figure 3 Time-courses of electric eel AChE inhibition by 0.1 mM DFP in the absence and presence of 10% ethanol. Inset: semilogarithmic plot.
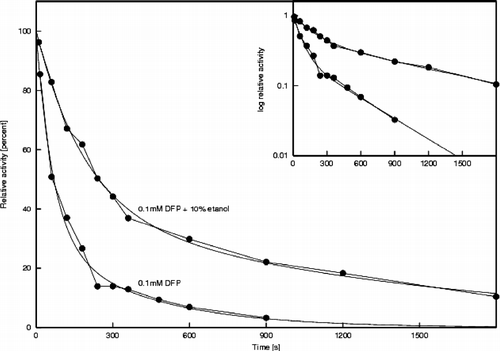
Finally, a possible transacetylation in the presence of high alcohol concentrations in a cholinesterase reaction was investigated by mixing horse BChE with acetylcholine in a buffer solution containing 20% ethanol. Aliquots were analyzed by gas chromatography immediately and after various incubation times. shows selected sweeps performed under several conditions. Here, it can be seen that under these conditions acetylcholine () is partially non-enzymatically converted into ethyl acetate, but only after 2 hours of incubation with ethanol (Figure 5E). In the presence of the BChE, however, some acetylcholine is immediately hydrolyzed (compare and ), and the concentration of ethyl acetate after a 2-hour incubation is much lower than in the absence of the BChE (compare and ).
Figure 4 Gas chromatograms of various mixtures of ethanol, acetylcholine, cholinesterase, ethyl acetate and sulfuric acid: A) the remaining ethanol from the previous run + ethyl acetate (20%); B) sodium acetate (2 mM) + ethanol (20%)+ sulfuric acid (2 mM); C) ethanol (20%)+ acetylcholine (50 mM); D) ethanol (20%)+ acetylcholine (50 mM) + cholineterase (∼1 nM); E) ethanol (20%)+ acetylcholine (50 mM), after a 2-h incubation; F) ethanol (20%)+ acetylcholine (50 mM) + cholineterase (∼1 nM), after a 2-h incubation.
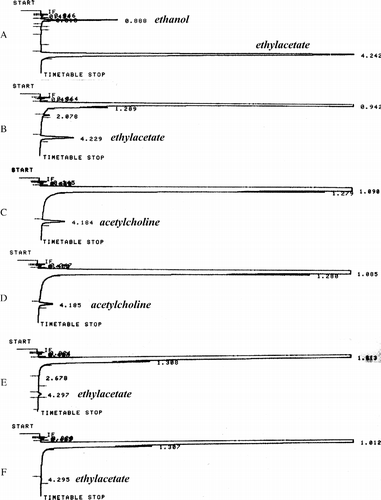
Discussion
Cholinesterases convert their substrates at very high rates. Depending on the source of cholinesterase, the turnover number is between one thousand and ten thousand per second. This is achieved through a highly adapted active site, which is buried deep inside the molecule. Consequently, a substrate molecule that collides with the enzyme is conducted very rapidly towards the catalytic triad by two-dimensional surface diffusion, where the cleavage takes place within a few vibration cycles. Similar events occur when a water molecule regenerates the enzyme. However, the buried active site can also have some disadvantages, and the most distinctive of these is inhibition by excess of substrate. This can occur as a result of impaired traffic upon the subsequent approach of a second substrate molecule, before the preceding hydrolytic products have left the gorge. Our results here suggest that alcohol molecules can interfere with precisely these processes. They hinder the substrate molecules when they approach the active-site gorge, and in this way decrease the enzyme activity; however, they also compete with the substrate when it obstructs its own turnover. These effects are seen as inhibition at low substrate concentrations and a shift in the optimum and the substrate inhibition towards higher substrate concentrations. Moreover, the optimum can be increased or reduced, and again this depends on the source of the enzyme and the size of the alcohol; while the smaller methanol molecule increases the optimum in all of the three cholinesterases tested (), the larger ethanol molecule reduces it in the vertebrate AChE, increases it slightly in the insect AChE, and has no influence on the optimum of the horse BChE. The uniform effect of methanol and the diverse effects of ethanol might not be a surprise. It is the size of ethanol that allows it to fit into the gorge to prevent the entrance of substrate into the best adapted electric eel AChE, while the predominant effect is on the approach of the substrate to the insect AChE, the enzyme with the most pronounced peripheral site. On the other hand, the active-site gorge of the horse BChE is larger, more open and, due to the lack of a tryptophan, with a less defined peripheral site. The approach of the substrate is thus more chaotic, and the products are less efficiently hindered upon their exit, even by very high substrate concentrations.
To verify this ‘approach and exit’ hypothesis, we investigated the effects of methanol on the turnover by the vertebrate and insect AChEs of the related carbamate, eserine. This powerful inhibitor can actually be treated as a true substrate, but with a slow carbamoylation, i.e. the inhibition step, and a very slow decarbamoylation, i.e. the regeneration process. Therefore, this allows the hydrolysis of eserine to be followed through its entire course, until completion. Although it might appear at first glance from that the inhibition (roughly, the downward parts of the curves) and regeneration (the upward parts of the curves) are equally delayed in the presence of 15% methanol, the determination of the corresponding rate constants revealed a 4-fold slower carbamoylation, with decarbamoylation being unaffected. Moreover, approximately the same delays were also measured in the phosphorylation of electric eel AChE by DFP in the presence of 10% ethanol (). It therefore appears that the only reasonable explanation for these findings is a protection of the AChE by these alcohols towards the attack by acylating agents. Since the reaction mechanism is essentially the same for the fast-converting substrate, our interpretation of the information in appears appropriate.
Finally, to determine directly the possible effects of ethanol on the chemical steps in the reaction mechanism of the cholinesterases, we compared a possible accumulation of ethyl acetate following the putative enzymatic ethanolysis of acetylcholine with the spontaneous process and with the process in the presence of sulphuric acid. confirms that without sulphuric acid, the equilibrium is very unfavorable for the ‘synthesis’ of ethyl acetate, and that the so-called transacetylation in the presence of ethanol is negligible. This, in turn, suggests that the deacylation step of the enzyme with the ethanol molecule at the catalytic anionic site (see steps ek3, fk3 and gk3) allows for productive water accommodation and product exit, rather than enzymatic (m)ethanolysis.
Abbreviations | ||
AChE: | = | acetylcholinesterase; |
BChE: | = | butyrylcholinesterase; |
ATCh: | = | acetylthiocholine; |
BTCh: | = | butyrylthiocholine; |
DFP: | = | diisopropyl fluorophosphate |
Acknowledgements
This work was supported by the Ministry of Defence of the Republic of Slovenia, grant no. PO-0505-0381.
References
- Krupka RM. Chemical structure and function of the active centre of acetylcholinesterase. Biochemistry 1966a; 5: 1988–1997
- Sussman JL, Harel M, Frolow F, Oefner C, Goldman A, Toker L, Silman I. Atomic structure of acetylcholinesterase from Torpedo californica: A prototypic acetylcholine-binding protein. Science 1991; 253: 872–879
- Masson P, Froment MT, Bartels CF, Lockridge O. Asp70 in the peripheral anionic site of human butyrylcholinesterase. Eur J Biochem 1996; 235: 36–48
- Colletier JP, Fournier D, Greenblatt HM, Stojan J, Sussman JL, Zaccai J, Silman I, Weik M. Structural insights into substrate traffic and inhibition in acetylcholinesterases. EMBO J 2006; 25: 2746–2756
- Szegletes T, Mallender WD, Rosenberry TL. Non-equilibrium analysis alters the mechanistic interpretation of inhibition of acetylcholinesterase by peripheral site ligands. Biochemistry 1998; 37: 4206–4216
- Stojan J, Brochier L, Alies C, Colletier JP, Fournier D. Inhibition of Drosophila melanogaster acetylcholinesterase by high concentrations of substrate. Eur J Biochem 2004; 271: 1364–1371
- Stojan J. Rational polynomial equation helps to select among homeomorphic kinetic models for the cholinesterase reaction mechanism. Chem Biol Interact 2005; 15: 173–179
- Shin S, Wu P, Chen C-H. Biochemical studies of the actions of ethanol on acetylcholinesterase activity: Ethanol-enzyme-solvent interaction. Int J Biochem 1991; 23(2)169–174
- Ellman GL, Courtney KD, Andres V, Featherstone RM. A new and rapid colorimetric determination of acetylcholinesterase activity. Biochem Pharmacol 1961; 7: 88–95
- Pavlič M, Alif M, Božič M, Stojan J. On the stability of some enzymes under various experimental conditions. Period Biol 1991; 93(2)211–216
- Van Slyke DD, Cullen GE. The mode of action of urease and of enzymes in general. J Biol Chem 1914; 19: 141–180
- Stojan J. Analysis of progress curves in an acetylcholinesterase reaction: A numerical integration treatment. J Chem Inf Comput Sci 1997; 37: 1025–1027
- Stojan J, Zorko M. Kinetic characterization of all steps of the interaction between acetylcholinesterase and eserine. Biochim Biophys Acta 1997; 1337: 75–84
- Alberty RA, Miller WG. Integrated rate equations for isotopic exchange in simple reversible reactions. J Chem Phys 1957; 26: 1231–1237
- Chaabihi H, Fournier D, Fedon Y, Bossy JP, Ravallec M, Devauchelle G, Cerutti M. Biochemical characterization of Drosophila melanogaster acetylcholinesterase expressed by recombinant baculoviruses. Biochem Biophys Res Commun 1994; 203: 734–742
- Estrada-Mondaca S, Fournier D. Stabilization of recombinant Drosophila acetylcholinesterase. Protein Expr Purific 1998; 12: 166–172
- Levy D, Ashani Y. Synthesis and in vitro properties of a powerful quaternary methylphosphonate inhibitor of acetylcholinesterase. A new marker in blood-brain barrier research. Biochem Pharmacol 1986; 35: 1079–1085
Appendix
A. The reaction scheme for substrate hydrolysis by the cholinesterases in the presence of an inhibitor that competes at the catalytic site and the peripheral anionic site. The inhibitor is assumed to be small, so that all of the acylated enzyme species that include this inhibitor in the active site can yield products.
The derived initial rate equation using mixed equilibrium and steady-state assumptions is as follows:
where
and
B. The following general reaction scheme can be used to describe data of residual enzyme activities after various times of incubation with surplus concentrations of DFP, both in the absence and presence of ethanol:
This scheme represents mechanism 10 in reference 12. The corresponding equation is:
where Et represents the activity at any time, C1 and C2 are complex constants that are composed of the corresponding mechanism-specific rate constants and initial enzyme activities, and m1 and m2 are mechanism-specific combinations of rate constants (for details see Equations 11–14 in ref. [Citation12].