Abstract
Tryptophanase (tryptophan indole-lyase, Tnase, EC 4.1.99.1), a bacterial enzyme with no counterpart in eukaryotic cells, produces from L-tryptophan pyruvate, ammonia and indole. It was recently suggested that indole signaling plays an important role in the stable maintenance of multicopy plasmids. In addition, Tnase was shown to be capable of binding Rcd, a short RNA molecule involved in resolution of plasmid multimers. Binding of Rcd increases the affinity of Tnase for tryptophan, and it was proposed that indole is involved in bacteria multiplication and biofilm formation. Biofilm-associated bacteria may cause serious infections, and biofilm contamination of equipment and food, may result in expensive consequences. Thus, optimal and specific factors that interact with Tnase can be used as a tool to study the role of this multifunctional enzyme as well as antibacterial agents that may affect biofilm formation. Most known quasi-substrates inhibit Tnase at the mM range. In the present work, the mode of Tnase inhibition by the following compounds and the corresponding Ki values were: S-phenylbenzoquinone-L-tryptophan, uncompetitively, 101 μM; α-amino-2-(9,10-anthraquinone)-propanoic acid, noncompetitively, 174 μM; L-tryptophane-ethylester, competitively, 52 μM; N-acetyl-L-tryptophan, noncompetitively, 48 μM. S-phenylbenzoquinone-L-tryptophan and α-amino-2-(9,10-anthraquinone)-propanoic acid were newly synthesized.
Keywords::
Introduction
Tryptophanase (tryptophan indole-lyase, Tnase, EC 4.1.99.1) is a pyridoxal 5′-phosphate (PLP)-dependent enzyme. It is a multifunctional enzyme that catalyses α,β-elimination and β-replacement reactions of L-tryptophan and a number of other β-substituted amino acids. The quaternary structure of Tnase consists of 4 identical 52 kDa subunits; each binds one molecule of PLP via an aldimine bond with a lysine residue. Its holo (active) form exhibits a pH-dependent absorption and CD spectra with maxima at 420 and 337 nm typical for covalently bound PLP Citation1, Citation2, Citation3. In addition, it requires certain monovalent cations (K+, , TI+) for activity and for tight PLP binding Citation4, Citation5, Citation6. The enzyme undergoes a reversible inactivation followed by dissociation into dimers or monomers, depending on the bacteria species, after incubation for several hours at 2°C [Citation7]. The enzymatic reaction with tryptophan is shown in Scheme .
Scheme 1. The enzymatic reaction of Tnase with tryptophan results in pyruvate, ammonia and indole production [Citation8].
![Scheme 1. The enzymatic reaction of Tnase with tryptophan results in pyruvate, ammonia and indole production [Citation8].](/cms/asset/4a1edcc3-146f-48b7-b8c6-bb65a7b70db0/ienz_a_318928_f0001_b.gif)
A class of biofilm regulatory molecules known as “cell-to-cell signaling molecules” or CCSMs was identified. Among them are N-acylhomoserine lactones, peptides, and quinolones [Citation9]. Recently it was suggested that indole is a CCSM at the site of infection both in Escherichia coli and Vibrio cholera, Citation10, Citation11, Citation12, Citation13, Citation14, Citation15, Citation16, Citation17. The prokaryotic enzyme Tnase is widely distributed in pathogenic Gram-negative bacteria, including E. coli, Shigella flexeneri, V. cholera, Haemophilus influenzae, and Clostridium tetani, with no counterpart in eukaryotic cells. Escherichia coli are a very efficient colonizer of a wide variety of surfaces. Recent experiments demonstrated that the tnaA gene, which encodes Tnase, is important for biofilm formation in E. coli and in several other species of pathogenic bacteria Citation10, Citation11, Citation12, Citation13, Citation14, Citation15, Citation16, Citation17. It was reported that indole can restore biofilm phenotype in tnaA mutants [Citation18]. On the other hand, it was recently reported that indole signaling plays an important role in the stable maintenance of multicopy plasmids. In addition, Tnase was shown to be capable of binding Rcd, a short RNA molecule involved in resolution of plasmid multimers. Binding of Rcd increases the affinity of Tnase for tryptophan, suggesting that Tnase serves as a multifunctional enzyme [Citation19].
The most efficient inhibitor of Tnase known hitherto, oxindolyl-L-alanine, (Ki = 5μM) inhibits both the formation of indole and the creation of biofilm [Citation20,Citation11]. Other quasi-substrates of Tnase such as alanine and phenylalanine inhibit the enzyme in the mM concentrations range [Citation21].
We now report on our finding of four inhibitors of Tnase, which inhibit at sub-milli to micro molar concentrations. The compounds were chosen based on mechanistic rationales, i.e., N-acetyl-L-tryptophan and S-phenyl benzoquinone-L-tryptophan, solubility, i.e., L-tryptophan-ethylester, and a combination of known inhibitory characteristics with active site targeting, i.e., α-amino-2-(9,10-anthraquinone)-propanoic acid. Here we describe the synthesis of two new compounds S-phenylbenzoquinone-L-tryptophan and α-amino-2-(9,10-anthraquinone)-propanoic acid.
Experimental
Materials
N-acetyl-L-tryptophan and L-tryptophan-ethylester were purchased from Sigma-Aldrich.
Protein expression and purification
E. coli wild-type (wt) Tnase was expressed and purified as described before [Citation22].
Synthesis of inhibitors
α-Amino-9,10-dihydro-9,10-dioxo-2-anthracenepropanoic acid
The L isomer of this compound was prepared in 3 stages starting from 2-anthracenepropanoic acid according to the method of Matsubara et al. [Citation23]: mp 230-231°C (lit mp 229–230°C).
1H-NMR (DMSO-d6): δ = 8.23 ppm (m, 2H, aromatic CH), δ = 8.14 ppm (d, J = 7.25 Hz, 1H, aromatic CH) δ = 7.95 ppm (d, J = 1.2 Hz, 1H, CH aromatic), δ = 7.73 ppm (m, 2H, aromatic CH), δ = 7.45 ppm (dd, J = 7.5 Hz, 1.2 Hz, 1H, aromatic CH), δ = 6.65 ppm (2H, bs, NH2,), δ = 4.2 ppm (m, 1H, chiral CH), δ = 3.4 ppm (m, 2H, CH2).
MS. (FAB) m/z (%)296 (40)MH+223(27).
S-phenylbenzoquinone-L-tryptophan
To a hot stirring solution of 2-phenylthio-1,4-benzoquinone (1.08 gr, 5 mmol) in 95% ethanol (50 mL) an aqueous solution (20 mL) of tryptophan (1.02 gr, 5 mmol) was added drop wise [Citation24]. The mixture was left to stir at room temperature for 24 h. Within this time, the obtained hydroquinonic product was re-oxidized to the quinonic end product. The solvents were evaporated in vacuo and the crude product was purified on a silica gel column using a gradient of dichloromethane/ethanol. Dark red micro-crystals were obtained (1.67 gr, 80% yield). Mp 223-225°C (dec).
In the HR-MS (CI in CH4) the compound loses HCOOH, thus we obtain: m/z (M+‐HCOOH) 372.0934, calc. for C22H16O2N2S, 372.0932.
1H-NMR (DMSO-d6): δ = 10.3 ppm (s, 1H, COOH), δ = 7.5 ppm (s, 5H, aromatic CH), δ = 7.48 ppm (d, J = 8 Hz, 1H, aromatic CH), δ = 7.2-7.3 ppm (d, J = 8 Hz aromatic, 1H, CH), δ = 7.0 ppm (s, 1H, indolic CH), δ = 7.0 ppm (t, J = 8 Hz, 1H, aromatic CH), δ = 6.9-7.0 ppm (t, J = 8 Hz, 1H, aromatic CH) δ = 5.4 ppm (s, 1H, quinonic CH), δ = 5.2-5.3 ppm (s, 1H, quinonic CH), δ = 3.3 ppm (m, 1H, chiral CH), δ = 2.8 ppm (m, 2H, CH2).
IR(KBR): OH (carboxylic) (3346 cm− 1), CH aromatic (3025, 2823 cm− 1), C = O (carboxylic and quinonic) (1651 cm− 1, 1598 cm− 1, 1553 cm− 1).
Kinetic measurements
The activity of Tnase was determined spectrophotometrically with the use of S-(o-nitrophenyl)-L-cysteine (SOPC) as a substrate [Citation25]. In the presence of Tnase SOPC undergoes α,β-elimination reaction as shown bellow in Scheme .
Scheme 2. The α,β-elimination reaction of SOPC results in the production of o-nitrothiophenolate, pyruvate and ammonia.

SOPC has a maximum absorption band at 370 nm with a molar absorption coefficient ε = 2700M− 1 cm− 1 and the product o-nitrothiophenolate has a maximum absorption band at 412 nm with a molar absorption coefficient ε = 1860 M− 1 cm− 1. All analyses were done in potassium phosphate buffer (50 mM, pH = 7.8) (KPB) using 0.06-0.6 mM SOPC. The reaction mixture and the enzyme solution are kept at 25°C for ten minutes. Then, 1 mL of the reaction mixture is placed in a cuvette, and 10 μL of the enzyme solution (0.3-0.5 mg protein/mL, specific activity 40-47 μmol × min− 1 mg− 1) is added. The reaction is followed for 1 min. The reaction is carried out at [S]≫Km (Km = 0.06 mM) and therefore, V = Vmax, thus, the velocity of the reaction is not dependent on the concentration of the substrate (zero order kinetics). One activity unit of Tnase is defined as the amount of enzyme needed to break down 1 μmol of the substrate SOPC to its products in one minute at 25°C (1 unit = 1 μmol × min− 1). The specific activity of Tnase is defined according to the following equation:
where, ΔA370 nm is the change in absorption during a period of 1 minute (O.D. 0.2-0.5); Vt – the total volume of the reaction mixture in mL; Ve – the volume of protein taken for analysis, in mL, C - enzyme concentration, and the difference between the molar extinction coefficients of SOPC and its degradation products at pH = 7.8 (1860 M− 1 × cm− 1 = 1.86 mL × μmole− 1 × cm− 1) [Citation25].
The measurements were done with an 8453A Hewlett Packard spectrophotometer connected to a UC-F-10 Julabo thermostated bath ( ± 0.1°C).
All kinetic experiments were repeated three to four times (results show the average; standard deviation amounted to less than 5%).
Results
A Michaelis-Menten fit for S-phenylbenzoquinone-L-tryptophan calculated with non-linear regression, as a function of SOPC concentration is shown in . All calculations were done with Calidagraph (version 4). summarizes the values calculated with non-linear regression for the inhibitors N-acetyl-L-tryptophan, S-phenylbenzoquinone-L-tryptophan, tryptophan-L-ethylester and α-Amino-2-(9,10-anthraquinone)-propanoic acid; R (correlation factor) was found to be >0.99 for all. presents the suggested mode of Tnase inhibition by each inhibitor and the corresponding Ki values. All four inhibitors are shown to be reversible, whether competitive, non-competitive, or uncompetitive (). Lineweaver-Burk plots of the specific activity as a function of SOPC concentration resulted in similar inhibition characteristics.
Figure 1. Michaelis-Menten plot of Tnase with S-phenyl-benzoquinone tryptophan. SOPC at different concentrations was used as a substrate, as described in the experimental section. Analyses were done with Calidagraph version 4. Measurements were performed in the absence, ○, and the presence of the inhibitor at a concentration of 47.5 μM, ◊; 95 μM, ▪; 190 μM, X.
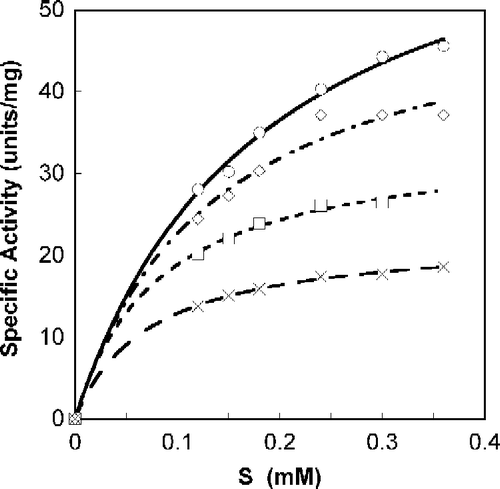
Table I. Kinetics parameters of the Tnase inhibitors.
Figure 2. Michaelis-Menten plot of Tnase with N-acetyl-tryptophan. SOPC was used as a substrate at different concentrations. Measurements were performed in the absence, ○, and the presence of the inhibitor at a concentration of 15.5 μM, ◊; 31 μM, ▪; 62 μM, X.
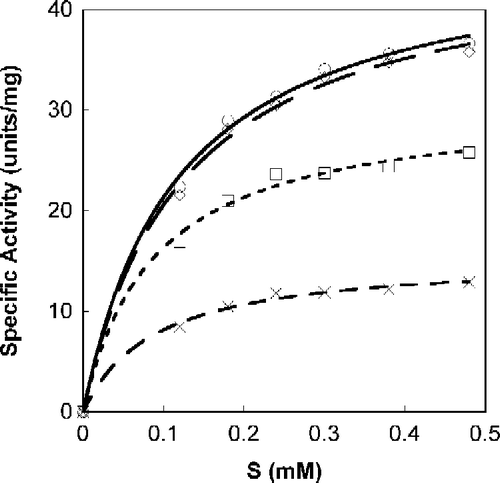
Discussion
Several crystal structures of Tnase were reported. The structure of E. coli apo enzyme was solved in two crystal forms [Citation26,Citation27] and the structure of a highly homologous Tnase from P. vulgaris was solved in the holo form [Citation28]. The three structures hold significant deviations in the relative orientation of the ‘large’ and ‘small’ domains and, as a consequence, show variations in the width of the catalytic site cleft and the geometry of the cofactor binding site. The conformational changes of Tnase molecule are supposed to be of functional importance [Citation26], in a way similar to tyrosine-phenol lyase [Citation29]. The flexibility of Tnase makes a structure based design of specific inhibitors a challenging task. Here we present our finding of four Tnase inhibitors. Two of the inhibitors, N-acetyl-L-tryptophan and S-phenyl benzoquinone-L-tryptophan were rationalized based on the known mechanism of Tnase catalysis. It is suggested that designing tryptophan derivatives with enhanced detachment of the α-proton (the proton attached to the chiral carbon) will result in the acceleration of the initial step of the catalytic mechanism (Scheme , the conversion of (2) to (3) [Citation21]).
Scheme 3. The mechanism of catalysis of Tnase as proposed by Snell & Mari [Citation21].
![Scheme 3. The mechanism of catalysis of Tnase as proposed by Snell & Mari [Citation21].](/cms/asset/6d84e86d-994d-4a61-87a5-a3d958d6f3a5/ienz_a_318928_f0007_b.gif)
In this step the α-proton of the complexed L-tryptophan (2) is detached by a catalytic amino acid, which serves as a base in the Tnase active site, giving rise to structure (3). The present results suggest that S-phenyl benzoquinone-L-tryptophan is uncompetitive inhibitor while N-acetyl-L-tryptophan is noncompetitive. L-tryptophan-ethylester was chosen as a possible inhibitor due to its decreased polarity which enables it to cross non polar barriers (e.g., the cell membrane) better than the free acid [Citation30,Citation31]. Since a competitive inhibition is observed with L-tryptophan-ethylester, it is proposed that this inhibitor binds to the active site of the enzyme.
A variety of quinones are known to act as inhibitors of various metabolic paths in the cell. Some natural quinones and some specially designed synthetic quinones are known to serve as antibacterial, antiviral, and even anti-cancer agents Citation32, Citation33, Citation34, Citation35. Tryptophanase can bind several amino acids; therefore, we designed and prepared in a 4-stage synthesis an anthraquinone that is covalently attached to the side chain of alanine, α-amino-2-(9,10-anthraquinone)-propanoic acid. This compound showed most likely a non-competitive inhibition with a Ki of 174 μM. Such a noncompetitive inhibition may suggest that it does not enter the active site. Instead, it may either sterically block the entrance of the substrate or induce conformational changes in Tnase which prevent binding of the substrate to the active site. Lineweaver-Burk analysis led to similar conclusions regarding the mode of inhibition.
It was suggested that indole can act as an extracellular signaling molecule that activates the astD, tnaB, and gabT genes in a concentration-dependent manner. To date, there is no direct evidence that E. coli produces any of the N-acyl homoserine lactone signaling molecules commonly used in other Gram-negative bacteria. Therefore, E. coli may have evolved to utilize alternative signals, such as the accumulation of certain metabolites. Signaling via metabolites may allow cells to fine-tune the regulation of target genes in response to changing environmental conditions [Citation10].
In conclusion, we evaluated four new inhibitors of tryptophanase with Ki values between 48 μM and 174 μM. The compounds were chosen based on mechanistic rationales, solubility and a combination of known inhibitory effects. Thus, optimal and specific factors that interact with Tnase can be used as a decorous tool to study the role of this multifunctional enzyme.
Acknowledgements
We thank Dr. Yana Sotovsky for her helpful synthesis discussions. We are grateful for Prof. Yuri Torchinsky for introducing Tnase into our laboratory. This work was supported in part by the James Frank foundation, to AHP.
Declaration of interest: The authors report no conflicts of interest. The authors alone are responsible for the content and writing of the paper.
References
- T Ben-Kasus, A Markel, G Gdalevsky, YM Torchinsky, RS Phillips, and AH Parola. (1996). Interactions of Escherichia coli tryptophanase with quasisubstrates and monovalent cations studied by the circular dichroism and fluorescence methods. Biochim Biophys Acta 1294:147–152.
- EE Snell. (1962). Comparison between some pyridoxal-dependent enzymatic and non-enzymatic reactions. Brookhaven Symp Biol 15:32–51.
- CM Metzler, R Viswanath, and DEJ Metzler. (1991). Equilibria and absorption spectra of tryptophanase. Biol Chem 266:9374–9381.
- A Hogberg-Raibaud, O Raibaud, and ME Goldberg. (1975). Kinetic and equilibrium studies on the activation of Escherichia coli K12 tryptophanase by pyridoxal 5′-phosphate and monovalent cations. J Biol Chem 250:3352–3358.
- CH Suelter, and EE Snell. (1977). Monovalent cation activation of tryptophanase. J Biol Chem 252:1852–1857.
- T Toraya, T Nihira, and S Fukui. (1976). Pyridoxal-5′-phosphate-sensitized photoinactivation of tryptophanase and evidence for essential histidyl residues in the active sites. Eur J Biochem 69:411–419.
- O Almog, A Kogan, M de Leeuw, R Cohen-Luria, and AH Parola. (2008). A structural insight into cold inactivation of tryptophanase and cold adaptation of S41: Minireview. Biopolymers 89:354–359.
- WA Newton, and EE Snell. (1962). An inducible tryptophan synthase in tryptophan auxotrophs of Escherichia coli. Proc Natl Acad Sci USA 48:1431–1439.
- MB Miller, and BL Bassler. (2001). Quorum sensing in bacteria. Annu Rev Microbiol 55:165–199.
- D Wang, X Ding, and PN Rather. (2001). Indole can act as an extracellular signal in Escherichia coli. J Bacteriol 183:4210–4216.
- P DiMartino, R Fursy, L Bret, B Sundararaju, and RS Phillips. (2003). Indole can act as an extracellular signal to regulate biofilm formation in Escherichia coli and in other indole-producing bacteria. Can J Microbiol 49 (7):443–449.
- RS Mueller, D McDougald, D Cusumano, N Sodhi, S Kjelleberg, F Azam, and DH Bartlett. (2007). Vibrio cholerae strains possess multiple strategies for abiotic and biotic surface colonization. J Bacteriol 189 (14):5348–5360.
- X Zhang, R García-Contreras, and TK Wood. (2007). YcfR (BhsA) influences Escherichia coli biofilm formation through stress response and surface hydrophobicity. J Bacteriol 189 (8):3051–3062.
- J Domka, J Lee, and TK Wood. (2006). YliH (BssR) and YceP (BssS) regulate Escherichia coli K-12 biofilm formation by influencing cell signaling. Appl Environ Microbiol 72 (4):2449–2459.
- J Lee, A Jayaraman, and TK Wood. (2007). Indole is an inter-species biofilm signal mediated by SdiA. BMC Microbiol 7:42. (doi:10.1186/1471-2180-7-42)
- J Lee, T Bansal, A Jayaraman, WE Bentley, and TK Wood. (2007). Enterohemorrhagic Escherichia coli biofilms are inhibited by 7-hydroxyindole and stimulated by isatin. Appl Environ Microbiol 73:4100–4109.
- J Domka, J Lee, T Bansal, and TK Wood. (2007). Temporal gene-expression in Escherichia coli K-12 biofilms. Environ Microbiol 9 (2):332–346.
- RS Phillips, K Ravichandran, and RL Von Tersch. (1989). Synthesis of L-tyrosine from phenol and S-(o-nitrophenyl)-L-cysteine catalysed by tyrosine phenol-lyase. Enzyme Microb Technol 11:80–83.
- EL Chant, and DK Summers. (2007). Indole signalling contributes to the stable maintenance of Escherichia coli multicopy plasmids. Molec Microbiol 63 (1):35–43.
- RS Phillips, EW Miles, and LA Cohen. (1984). Interactions of tryptophan synthase, tryptophanase and pyridoxal phosphate with oxindolyl-L-alanine and 2,3-dihydro-L-tryptophan: Support for an indolenine intermediate in tryptophan metabolism. Biochemistry 23:6228–6234.
- EE Snell, and SJ Mari. Schiff base intermediates in enzyme catalysis. In: PD Boyer. editor. The Enzymes., vol. 2 (1970) p 335–370.
- A Kogan, GY Gdalevsky, R Cohen-Luria, AH Parola, and Y Goldgur. (2004). Crystallization and preliminary X-ray analysis of the apo form of Escherichia coli tryptophanase. Acta Cryst sec B 60:273–275.
- T Matsubara, H Shinohara, and M Sisido. (1997). Synthesis and conformation of poly(L-2-anthraquinonylalanine). Macromolecules 30:2651–2656.
- O Dimroth, L Kraft, and K Aichinger. (1940). Action of thiophenols on quinines. Ann Chem 54:5124–5139.
- CH Suelter, J Wang, and EE Snell. (1976). Application of a direct spectrophotometric assay employing a chromogenic substrate for tryptophanase to the determination of pyridoxal and pyridoxamine 5′-phosphate. Anal Biochem 76:221–232.
- N Tsessin, A Kogan, GY Gdalevsky, JP Himanen, R Cohen-Luria, AH Parola, Y Goldgur, and O Almog. (2007). The crystal structure of apo tryptophanase from Escherichia Coli reveals a wide open conformation. Acta Cryst D Biol Cryst 63:969–974.
- SY Ku, P Yip, and LP Howell. (2006). Structure of Escherichia coli tryptophanase. Acta Cryst D 62 (7):814–823.
- MN Isupov, AA Antson, EJ Dodson, GG Dodson, IS Dementieva, LN Zakomirdina, KS Wilson, Z Dauter, AA Lebedev, and EH Harutyunyan. (1998). Crystal structure of tryptophanase. J Mol Biol 276 (3):603–623.
- D Milic, D Matkovic-Calogovic, TV Demidkina, VV Kulikova, NI Sunitzina, and AA Antson. (2006). Structures of apo- and holo-tyrosine phenol-lyase reveal a catalytically critical closed conformation and suggest a mechanism for activation by K+ ions. Biochemistry 45 (24):7544–7522.
- BJ Gulielmo, and C MacDougall. (2004). Pharmacokinetics of valaciclovir. J Antimicrob Chemother 53 (6):899–901.
- CP Landowski, X Song, PL Lorenzi, JM Hifinger, and G Amidon. (2005). Floxuridine amino acid ester prodrugs: Enhancing Caco-2 permeability and resistance to glycosidic bond metabolism. Pharm Res 22 (9):1510–1518.
- AJ Lin, LA Coby, and AC Sartorelli. (1985). Quinones as anticancer agents: potential bioreductive alkylating agents. J Toxicol Environ Health 16 (5):665–672.
- H Chung, RG Harvey, RN Armstrong, and J Jarabak. (1987). Polycyclic aromatic hydrocarbon quinones and glutathione thioethers as substrates and inhibitors of the human placental NADP-linked 15-hydroxyprostaglandin dehydrogenase. J Biol Chem 262 (26):12448–12451.
- T Frew, G Powis, M Berggren, A Gallegos, RT Abraham, CL Ashendel, LH Zalkow, C Hudson, E Gruszecka-Kowalik, and EM Burgess. (1995). Novel quinone antiproliferative inhibitors of phosphatidylinositol-3-kinase. Anticancer Drug Des 10 (4):347–359.
- W Oettmeier, K Masson, and H Hecht. (2001). Heterocyclic ortho-quinones, a novel type of photosystem II inhibitors. Biochim Biophys Acta 1504 (2–3):346–351.