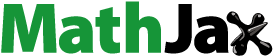
Abstract
The increase of bacterial strains resistant to most of the available antibiotics shows a need to explore novel antibacterial targets to discover antimicrobial drugs. Bifunctional bacterial FAD synthetases (FADSs) synthesise the flavin mononucleotide (FMN) and flavin adenine dinucleotide (FAD). These cofactors act in vital processes as part of flavoproteins, making FADS an essential enzyme. Bacterial FADSs are potential antibacterial targets because of differences to mammalian enzymes, particularly at the FAD producing site. We have optimised an activity-based high throughput screening assay targeting Corynebacterium ammoniagenes FADS (CaFADS) that identifies inhibitors of its different activities. We selected the three best high-performing inhibitors of the FMN:adenylyltransferase activity (FMNAT) and studied their inhibition mechanisms and binding properties. The specificity of the CaFADS hits was evaluated by studying also their effect on the Streptococcus pneumoniae FADS activities, envisaging differences that can be used to discover species-specific antibacterial drugs. The antimicrobial effect of these compounds was also evaluated on C. ammoniagenes, S. pneumoniae, and Mycobacterium tuberculosis cultures, finding hits with favourable antimicrobial properties.
Graphical Abstract

Introduction
An important innovation gap in the discovering of antibiotics has occurred during the last two decadesCitation1, with only five new classes available and 51 new antimicrobials in clinical developmentCitation2–4. In addition, the selection of multi-drug resistant microorganismsCitation5 encourages to search for new antimicrobial drugs capable of inhibiting novel protein targets, such as those controlling the biosynthesis of essential biomolecules. Flavin mononucleotide (FMN) and flavin adenine dinucleotide (FAD) are the cofactors of flavoproteins. All living organisms contain a great number of such proteins and many of them are involved in essential functionsCitation6–8, including protein foldingCitation9, electron transport in the respiratory and photosynthetic chainsCitation10, β-oxidation of fatty acidsCitation11, nucleotide synthesis or signal transductionCitation12, among others. Lack, or low levels, of FMN and FAD lead to the accumulation of apoflavoproteins, unable to perform the flavin-dependent functions, resulting in the concomitant death of the cell or the organismCitation13,Citation14. Prokaryotic bifunctional FAD synthetases (FADS) synthesise both FMN and FAD, being therefore potential new antimicrobial targetsCitation15. Such hypothesis is sustained by several facts; (i) halting the production of FMN and FAD prevents, from the very beginning, all pathways that involve flavoproteins and flavoenzymes, (ii) in most bacteria the only pathway for FMN and FAD biosynthesis occurs with bifunctional FADSCitation13,Citation14, (iii) prokaryotic FADSs differ structurally and biochemically from the mammalian proteins that transform FMN into FADCitation16–19, so drugs that target these proteins are likely to be selective for bacteria and (iv) the availability of structures of several bacterial FADSs facilitates the design of both inhibitory drugs and activity assaysCitation20–22.
Bacterial FADSs have both ATP:riboflavin kinase (RFK, EC 2.7.1.26) and ATP:FMN:adenylyltransferase (FMNAT, EC 2.7.7.2) activities, being the latter reversible (FAD pyrophosphorylase) in some speciesCitation17,Citation23. FADSs synthesise FMN and FAD from riboflavin (RF, vitamin B2) through two sequential reactions: RF is first phosphorylated to FMN by the RFK activity, and then the FMNAT activity transfers an adenylyl group from ATP to FMN producing FAD. These catalytic activities are performed by two almost independent modules (Supplementary Figure SD1). The C-terminus module produces FMN from RF (named RFK module), while the N-terminal module transforms FMN into FAD (FMNAT module). The RFK module shows sequence and structural homology with the monofunctional eukaryotic RFKs, while the FMNAT module does not present neither sequence nor structural similarity with the proteins that synthesise FAD in mammalsCitation16,Citation21,Citation24,Citation25. Because the enzymes leading to FAD production in prokaryotes and eukaryotes use different chemistry, and belong to different structural families, potential inhibitors that specifically target the FMNAT module of bacterial FADSs are an interesting option for the novel drug developmentCitation15.
In this work, we have used as a model the FADS from the non-pathogenic organism Corynebacterium ammoniagenes (CaFADS), which is the best known model to characterise members of the prokaryotic FADSs familyCitation17,Citation24,Citation26–30, in an activity-based high-throughput screening (HTS) assay to find potential inhibitors. The HTS hits were assayed to determine their specificity and potency for the RFK and FMNAT activities. We also studied the kinetic inhibition mechanism of the three most potent and selective inhibitors of the FMNAT activity (FMNAT hits), as well as their binding properties. Furthermore, considering the structural similarity among CaFADS and the FADSs from the human pathogens Streptococcus pneumoniae (included in the World Health Organisation [WHO] priority list of antibiotic resistant pathogens; SpnFADS) and Mycobacterium tuberculosis (the World’s leading infectious killer; MtFADS), we explored the potential antimicrobial effect of the FADS HTS hits in these microorganisms by determining their minimal inhibitory concentration (MIC). Some of the HTS hits demonstrated high FADS inhibitory activity in vitro, but their antimicrobial activity revealed that uptake of these compounds by bacterial cells could be suboptimal. Collectively, our results validate our approach for discovering antimicrobials targeting bacterial FADSs, and for identifying inhibitors that constitute a great starting point for future developments of novel antimicrobials.
Methods
Protein purification and quantification
Recombinant CaFADS was overexpressed in BL21 (DE3) E. coli cells and purified as previously describedCitation30. Recombinant SpnFADS was overexpressed in E. coli strain Bl21 StarTM (DE3) and purified as previously describedCitation23. Pure samples were dialysed against 20 mM PIPES, pH 7.0 and quantified using the theoretical extinction coefficients ε279=27.8 mM−1 cm−1 and 28.8 mM−1 cm−1 for CaFADS and SpnFADS, respectively. The purity of each protein was tested by 15% SDS-PAGE.
Chemicals
The Prestwick Chemical Library®, containing 1240 molecules approved by the Food and Drugs Administration (FDA) and European Medicines Agency (EMA), was selected for the HTS. Compounds were dissolved in 100% DMSO at 10 mM. All the HTS hits were subsequently acquired from Sigma Aldrich, Prestwick or Carbosynth and dissolved in 100% DMSO to prepare stock solutions at 50 and 10 mM. The purity of all compounds was >95%, as determined by High performance liquid chromatography (HPLC), thin layer chromatography (TLC), NMR, IR or basic titration.
Activity-based high-throughput screening for CaFADS
An activity-based HTS was performed on the 1240 compounds of the Prestwick Chemical Library®. The assays consisted in recording the time dependent decrease in the fluorescence of the isoalloxazine ring, produced upon transformation of RF and FMN into FAD, as a consequence of the fluorescence quenching in this later flavinCitation27. When either the RFK or the FMNAT activities were inhibited, less FAD was produced and, consequently, the fluorescence decrease registered in a specific time interval was less pronounced. Measurements were carried out using a multimode microplate reader, Synergy™ HT Biotek, with BRAND 96-well plates pure Grade™. To optimise the assay conditions, a previous study was performed using constant concentrations of RF, ATP and CaFADS (∼5, ∼50 and ∼0.4 µM, respectively) and variable concentrations of MgCl2 (0.2–10 mM) and DMSO (0–12.5% v/v). Optimum conditions were 2.5% DMSO, 10 mM MgCl2 and sensitivity 70.
HTS reaction mixtures contained 5 µM RF, 0.4 µM CaFADS, 10 mM MgCl2, in PIPES 20 mM, pH 7.0, 2.5% DMSO, and the corresponding compound of the chemical library at a final concentration of 250 µM. Reactions were initiated through addition of 50 µM ATP, being the final reaction volume 100 µl. Controls, which contained the reaction mixture but not any chemical from the library, were added both to the first and last columns of the plate. Flavin fluorescence in each well was registered at 25 °C, every 50 s during 15 min. Excitation and emission wavelengths were 440 and 530 nm, respectively. The slope of the resulting line, recorded between 0 and 6 min, was calculated for every compound, and also for the controls, as well as the fluorescence change per time unit (ΔF/Δt). The compounds that decreased the reaction rate below the average reaction rate of the controls minus the standard deviation could be preselected as potential inhibitors, but we reduced further the cut-off by selecting only those compounds inhibiting more than 50% of the controls activity as HTS hits.
Identification of the activity inhibited by each of the HTS hits
The decreasing of the reaction rate by the presence of the HTS hits might be consequence of the compounds inhibiting the RFK activity, the FMNAT one, or both of them; also, it could be a false positive due to the properties of pan assay interference compounds (PAINS). To clarify this point, we first checked that there were no PAINS among the HTS hits using the FAF-Drug4 web serverCitation31. Then, the RFK and FMNAT reactions were individually assayed in the presence of the HTS hits at 25 °C. Reaction mixtures contained 50 µM ATP, 5 µM RF, 0.4 µM CaFADS in 20 mM PIPES, pH 7.0, 0.8 mM MgCl2, when assaying the RFK activity, and 50 µM ATP, 10 µM FMN, 0.4 µM CaFADS in 20 mM PIPES, pH 7.0, 10 mM MgCl2 when measuring the FMNAT reaction. Each HTS hit was tested again at 250 µM for each of the two enzymatic reactions. Finally, reactions were stopped by boiling the samples at 100 °C for 5 min, and the precipitated protein was eliminated through centrifugation. The transformation of RF into FMN or FAD was evaluated through flavins separation by HPLC, as previously describedCitation27. Those HTS hits decreasing the FMNAT activity by more than 95% of the controls, without significantly affecting the RFK activity (rates over 75% those of the controls) were selected as FMNAT hits for further assessment. When assaying the HTS hits against the FMNAT activity of SpnFADS, similar conditions were used but samples contained 3 mM sodium dithionite to maintain the flavin in its reduced stateCitation23. Data were processed as previously reportedCitation27. All the experiments were performed in triplicate.
Determination of the potency of FMNAT hits
To determine the IC50 values of the FMNAT hits, the FMNAT activity was assayed at different concentrations of each inhibitor (0–100 µM range) and 25 °C. Experiments were performed and analysed through HPLC as described above. Positive controls (without any hit compound) were included in every reaction set. DMSO concentration was kept at 2.5% in all samples. All the experiments were performed in triplicate.
Determination of the inhibition mechanism of CaFADS by FMNAT hits 24, 27 and 31
The inhibition mechanism was further studied for the three FMNAT hits that showed the lowest IC50 and minimal residual FMNAT activities, namely, 24, 27 and 31. Reaction mixtures containing 0–100 µM of each compound, 1–20 µM FMN and 400 µM ATP were used when analysing the inhibitory effect of the compound regarding the FMN substrate, while 5–400 µM ATP and 15 µM FMN when analysing the effect of the inhibitor regarding the ATP substrate. All the experiments were carried out in 20 mM PIPES, 10 mM MgCl2, pH 7.0, 2.5% DMSO at 25 °C, being the final reaction volume 500 µl. The reactions were initiated by addition of CaFADS at a final concentration of ∼40 nM, followed by 1 min incubation. The flavin composition of the supernatant was analysed as previously describedCitation27. All the experiments were performed in triplicate. The effect of the inhibitors on Km and Vmax was determined by fitting the data sets to the Michaelis–Menten model. Additionally, data were globally fit to Lineweaver–Burk equations for competitive, uncompetitive, non-competitive or mixed inhibition, yielding Ki, as well as Ki′ when applying, for each compound (EquationEquations (1–4), respectively).
(1)
(1)
(2)
(2)
(3)
(3)
(4)
(4)
Thermodynamic characterisation of binding of hits 24, 27 and 31 through isothermal titration calorimetry (ITC)
ITC experiments were performed to characterise the protein’s affinity for the selected compounds, as also the thermodynamic parameters that drive the interaction. Experiments were carried out in an AutoITC200 (MicroCal) thermostated at 25 °C. In these experiments, ∼400 µM of each compound were used to titrate ∼25 µM CaFADS contained in a 200 µL cell. However, when saturation of the protein was not reached, higher concentrations of compounds were employed. The titrations were performed by stepwise injections of the titrating compound. Up to 19 injections of 2 µl were added to the cell sample and mixed using a 1000 rpm stirrer syringe. The compounds and the protein were dissolved in 20 mM PIPES, pH 7.0, 10 mM MgCl2 and degassed prior to titration. DMSO was added to the protein and ligand samples, until reach a final concentration of 3%. The association constant (Ka), the enthalpy variation (ΔH) and the binding stoichiometry (N), were obtained through non-lineal regression of the data to a model for one or two independent binding sites, implemented in Origin 7.0 (OriginLab) as previously describedCitation27,Citation29. The entropic contribution (−TΔS), the Gibbs free energy (ΔG) and the dissociation constant (Kd) were obtained through essential thermodynamic equations.
Docking of hits 24, 27 and 31 to the FMNAT module of CaFADS
The AutoDock4.2 softwareCitation32–34 and the coordinates of a monomer from CaFADS (PDB 2X0K)Citation24 were used to obtain the interaction models with 24, 27 and 31. The space sampling was defined using a grid box of 90 points in each dimension, and placing the H57 NE atom of the FMNAT module as the grid box centre. The grid size was 0.375 Å. The search was performed using the lamarkian genetic algorithm, with a starting population of 150 individuals, using 25,000,000 energy evaluations and 27,000 generations. The 24, 27 and 31 initial structures for the docking protocol were optimised using the functional B3LYP with the basis set 6–31 G (d,p) and the gaussian09 softwareCitation35. The structural poses with the lowest docking score were selected and analysed.
Determination of the antibacterial activity of the HTS hits
The antimicrobial activity of the HTS hits was tested by the colorimetric method of the resazurin microplate assayCitation36 according to broth microdilution method guidelines (CLSI; Clinical and Laboratory Standards Institute). Serial 2-fold dilutions of the HTS hits were performed in BHI medium, in 96-well microtiter plates, with a final volume of 100 µl per well. Subsequently, liquid cultures of C. ammoniagenes ATCC 6872 in logarithmic phase were adjusted to 106 CFU/ml in BHI broth, and 100 µl of this suspension were added to each well, making a final inoculum of 5 × 105 CFU/ml. Plates were incubated 16 h at 37 °C. 30 µl of 0.1 mg/ml resazurin solution were then added to each well, and results were observed after 4 h of incubation at 37 °C. Resazurin (blue) is an indicator of bacterial growth, since metabolic activity of bacteria reduces it to resorufin (pink). The minimum inhibitory concentration (MIC) is the lowest concentration of compound that does not change the resazurin colour from blue to pink.
Similarly, the HTS hits were also assayed at 37 °C against M. tuberculosis ATCC 27,294 and S. pneumoniae ATCC 49619 cells. In these experiments the initial cell concentration was also 5 × 105 CFU/ml, and plates were incubated for 10 h (S. pneumoniae), and 6 days (M. tuberculosis) before addition of resazurin. Results were observed after incubation with resazurin 4 h and two days for S. pneumoniae and M. tuberculosis, respectively. In these experiments, culture media were; Middlebrook 7H9 (Difco) supplemented with 10% ADC (0.2% dextrose, 0.5% V fraction BSA and 0.0003% bovine catalase) (BD Difco) and with 0.5% glycerol (Scharlau) for M. tuberculosis growth, and BHI supplemented with 4% FBS (Gibco) for S. pneumoniae growth.
Statistics
Results are expressed as the mean ± the standard deviation (SD) or as the mean ± the standard error (SE) of the regression. When indicated, one-way analysis of variance (ANOVA) was performed to determine statistical significance.
Results
Identification of potential inhibitors of the CaFADS activities through HTS
To identify potential inhibitors of the CaFADS activities we designed the activity-based HTS assay described in the methods section, which allowed to determine rates for the transformation of RF into FAD (via the FMN intermediate) in each one of the plate wells. Wells containing chemicals of the library and decreasing reaction rates relative to the controls (absence of chemicals) were preselected as containing compounds that are potential inhibitors of at least one of the CaFADS activities. Thus, among the 1240 compounds of the chemical library, 140 (13.5%) reduced the CaFADS activity levels below the mean of the positive controls minus twice its standard deviation, and of them, 37 (3.6%) reduced the positive controls average rate for FAD formation in a factor higher than 0.5 (). Those 37 compounds were selected as the HTS hits (Supplementary Chart SD1).
Figure 1. Activity-based high throughput screening (HTS) for the discovery of inhibitors of the RFK and/or the FMNAT activities of CaFADS. (A) Example of the flavin fluorescence evolution over time for three of the identified hits and for control assays. Reaction mixtures were incubated at 25 °C and contained 5 µM RF, 50 µM ATP, 0.4 µM CaFADS, 10 mM MgCl2, in PIPES 20 mM, pH 7.0, 2.5% DMSO. The black symbols and lines correspond to kinetic traces at wells containing library compounds at 250 µM, while grey ones correspond to control wells. (B) Initial velocities (Δfluorescence/s) for the reactions in each of the wells of a HTS plate. Data from wells containing chemical library compounds are in black while controls are in grey. The solid line represents the average velocity obtained for the positive controls of the reaction and the dotted lines are the average velocity plus and minus the standard deviation. The letters and numbers indicate the position of the well in the plate (row and a column respectively) for each specific selected measurement. A bold dashed line indicates 50% the rates of controls.

Effect of the HTS hits on the RFK and FMNAT activities of CaFADS
We then move to identify which one of the activities (RFK or FMNAT), and in which extension, was affected by each one of these 37 HTS hits. With this aim, we assayed the effect of the HTS hits both on the CaFADS RFK and FMNAT activities. and summarise the results. Comparison of shows that, in general and under the assay conditions, the 37 HTS hits produced a stronger deleterious effect on the FMNAT activity (all decreased the activity of the controls in more than 50%) than on the RFK one. The FMNAT module of CaFADS does not have sequence or structural homology with the mammalian protein but the RFK module belongs to the eukaryotic RFKs family. Therefore, we decided to continue the study with the HTS hits that inhibit the FMNAT activity, since they are more likely to be specific to the bacterial proteins. Thus, we choose the HTS hits that decreased the FMNAT activity below 5% of that of the controls, but maintained over 75% the RFK activity (, ). Thus, among (2)\(11), tiratricol (15), benzbromarone (17), oxantel pamoate (19), Chicago sky blue 6B (24), gossypol (27), flunixin meglumine (31) and oxaprozin (43) () were selected as FMNAT hits. It is worth noticing that although some of these compounds are apparently structurally related with other of the HTS hits, slight differences in functional groups and geometries induce different enzymatic responses in the FMNAT or RFK activities. This is a fact of particular interest when developing specific inhibitors.
Figure 2. Effect of the HTS hits on the RFK and FMNAT activities of CaFADS. Residual (A) RFK and (B) FMNAT activities when assayed in the presence of 250 μM of the 37 HTS hits. In (A), the columns below the dashed line present statistical significant inhibition by the corresponding hit (p < 0.002, 67% remaining activity) related to the control CaFADS RFK activity. In (B), all hits produce statistical significant inhibition (p < 0.0001, dashed line) when compared with the controls of the CaFADS FMNAT activity. Solid lines indicate 75 and 5% of the control RFK and FMNAT activities, respectively. The HTS hits displaying <5% and >75% of the control FMNAT and RFK activities, respectively, were selected for further study. Experiments carried out in 20 mM PIPES, pH 7.0, 2.5% DMSO at 25 °C, with 7.5 μM RF, 350 μM ATP, 0.8 mM MgCl2 (for the RFK activity) or 15 μM FMN, 350 μM ATP, 10 mM MgCl2 (for the FMNAT activity) (n = 3; mean ± SD).

Table 1. RFK and FMN residual activities of CaFADS in the presence of the HTS hits.
To rate the power of these 9 FMNAT hits as inhibitors of the CaFADS FMNAT activity, their half maximal inhibitory concentrations (IC50) and the remaining activity at 50 µM of each compound were determined (, ). The 9 compounds yielded IC50 values in the micromolar range, reducing by more than half the activity of the controls. Considering both their IC50 value and residual activity, the most potent inhibitors were 24 (IC50 = 0.4 ± 0.1 µM), 27 (IC50 = 0.5 ± 0.1 µM) and 31 (IC50 = 6.6 ± 0.6 µM). These three compounds produce residual activities below 25% of the controls. Compound 43 also showed high inhibitory potency, but due to its low solubility in the working buffer it was discarded.
Figure 3. Dose–response curves for the FMNAT activity of CaFADS in the presence of representative hits. Values derived from these representations are included in . Experiments performed at 25 °C in 20 mM PIPES, pH 7.0, 10 mM MgCl2, 2.5% DMSO, with 15 μM FMN and 350 μM ATP (n = 3, mean ± SD).

Table 2. Effect of the FMNAT hits on the FMNAT activity of CaFADS.
The inhibition mechanisms of 24, 27 and 31
To determine the inhibition mechanism of the hits 24, 27 and 31, we measured the FMNAT activity of CaFADS in the presence of increasing concentrations of each compound. Considering that this is a bi-substrate activity, for each compound we carried out two set of experiments at; (i) saturating ATP and different FMN concentrations, and (ii) saturating FMN and increasing ATP concentrations. Then we fit our experimental data to the Michaelis–Menten model, obtaining Km and kcat values. The analysis of the evolution of these constants on the hits concentrations, together with the corresponding Lineawever–Burk plots [representation of data as double inverses and fit to EquationEquations (1–4)] (, Supplementary Figures SD2 and SD3, Supplementary Table SP1) allowed identifying the inhibitory mechanisms of the 24, 27 and 31 hits, as well as the corresponding inhibition constants (Ki or, Ki and Ki′) (). The experiments carried out at saturating ATP (varying the FMN concentrations), revealed that the three compounds are strong non-competitive inhibitors of the CaFADS FMNAT activity regarding the FMN substrate (Ki values around 0.08 µM, ). Nevertheless, when using a constant and saturating FMN concentration but varying the ATP concentration, their inhibition mechanisms differ among them. 24 is a strong ATP uncompetitive inhibitor (Ki = 0.08 ± 0.03 µM), therefore, it is able to bind the CaFADS-ATP complex and reduce the amount of enzyme that is available to react. 27 is a strong competitive inhibitor regarding the ATP substrate (Ki = 0.06 ± 0.01 µM), while 31 is a considerably poorer mixed inhibitor. Thus, 31 is able to bind to both the free enzyme and the CaFADS-ATP complex, although binding constants indicate that binding to free CaFADS is preferred (Ki 3.5 ± 1.0 µM versus Ki′ 18.4 ± 4.0 µM, ).
Figure 4. Hit 27 as an inhibitor of the FMNAT activity of CaFADS. Michaelis–Menten plots at different concentrations of 27 and saturation (A) of ATP and (B) of FMN. Lineaweaver–Burk representations with global fit to (C) non-competitive inhibition at saturating ATP and (D) competitive inhibition at saturating FMN. Reaction rates obtained in 20 mM PIPES, pH 7.0, 10 mM MgCl2, at 25 °C, with 15 μM FMN and 10–450 μM ATP (FMN saturating) or with 350 μM ATP and 0.5–20 μM FMN (ATP saturating). All samples contained 2.5% DMSO (n = 3, mean ± SD).

Table 3. Inhibition constants and mechanisms of the best FMNAT hits relative to the FMNAT activity of CaFADS.
Binding of 24, 27 and 31 to CaFADS
The interaction of CaFADS with compounds 24, 27 and 31 was characterised using ITC. This is a very powerful technique because first the shape of the thermograms informs us about the number of binding sites related to the thermodynamic nature of binding. Then, fitting of experimental data to the equations describing binding models allow determining thermodynamic binding parameters, as well as binding stoichiometry at each of the different binding sites. Thus, analysis of our ITC titrations indicates that the three compounds bind the enzyme at, at least, one binding site (, ). Thus, we identified in CaFADS a unique binding site of moderate-low affinity for 31 (N ≈ 1, Kd = 30.9 ± 2.8 µM) and two binding sites of high and similar affinity for 27 (N ≈ 2, Kd,av = 0.7 ± 0.07 µM, this Kd,av value is an average value, since the similarity between the two binding sites prevents them to be distinguished). These 27 and 31 binding sites are expected to be located at the enzyme FMNAT domain, since that is the inhibited activity. The interaction between the hit 24 and the enzyme resulted more complicated, because our ITC data indicate that compound 24 binds to the protein at three sites. Two of them show similar and strong affinity (Kd,av = 1.1 ± 0.1 µM) and therefore, we suggest that they might be located at the FMNAT module, since that is the activity mainly inhibited by compound 24. The third site for 24 binding has lower affinity (Kd = 161 ± 20 µM). Since this compound also mildly inhibits the RFK activity, we presume this third binding site may be at the RFK module. All bindings here characterised (with the only exception of the low affinity binding site for 24) are favoured by the enthalpic contribution (, ). This suggests a net gain of H-bonding and ion-pair interactions and indicates specific binding interactions. Regarding the entropic contribution to the binding free energy, it is small and favourable for 24 and 27, and only slightly unfavourable for 31 (, ). However, it drives the binding of 24 to the RFK module (site 3 ), revealing that it might be non-specific and that could occur due to the compound hydrophobicity and rigidityCitation37.
Figure 5. Thermodynamic analysis of the binding of the selected FMNAT hits to CaFADS. (A) Calorimetric titrations for the 24, 27 and 31 compounds. The upper panels show the thermograms for the interaction and the lower panels show the corresponding binding isotherms with integrated heats. (B) Thermodynamic dissections of the interaction of CaFADS with each of the selected compounds. The binding Gibbs energy (ΔG), enthalpy (ΔH) and entropy (−TΔS) are represented in black, grey and white bars, respectively. Experiments were carried out at 25 °C, in 20 mM PIPES, pH 7.0, 10 mM MgCl2 and 3% DMSO.

Table 4. Thermodynamic parameters for the interaction of CaFADS with the hits 24, 27 and 31.
To investigate how the FMNAT module of CaFADS accommodates these compounds, we performed a computational protein-ligand docking ( and Supplementary Figure SD4). In the highest-scoring docking mode, as well as for the best five poses (purple molecule in , Supplementary Figure SD4, respectively), 241 (1 indicates the first molecule of 24 docked) is situated in the substrates binding pockets ()Citation24. One of the 241 moieties binds through one of its sulphates to S164 and H31. 241 is in addition H-bonded to the N125 catalytic baseCitation17, as well as to Y106, T127 and N131 at the loop that forms the upper flavin ring binding site (). Since our ITC data suggest two binding sites for compound 24 at the FMNAT module, we carried out an additional docking analysis to identify the second site, 242 (pink molecule in ), using as receptor our highest-scoring FADS:241 model. Considering the binding energies () this site is expected to be less populated, but given that 24 is an ATP uncompetitive inhibitor, the docking binding energy could improve if we consider the ATP substrate presence instead of the 241 molecule. Additionally, it is worth noting that in the presence of substrates or products, the 24 binding conformations might differ from the ones presented here. In the most favourable docking poses, the first molecule of 27 bound to the protein (271) is H-bonded by T127 and the N125 catalytic base at the binding site of the ATP phosphatesCitation17 (purple molecule in ), in agreement with 27 being an ATP competitive inhibitor. Because two binding sites were again predicted by ITC at the FMNAT site, we carried out a second docking using as receptor the highest-scoring FADS:271 model. 272 is stabilised by H-bonds with the N-terminal of the α6n helix, T165 and R168, at the ATP binding site entrance (pink molecule in ). We observed direct interaction between the two 27 molecules, and 272 somehow resembling the 241 binding (purple molecule in ). For 31, we only carried out the flunixin docking, given that this compound is the bioactive agent of 31 and meglumine is the excipient. The best poses for 31 binding are suggested at the ATP binding site ( and SD4), stabilised by a H-bond with H31. This binding is coherent with the ATP mixed inhibition mechanism of 31. In all cases, the H-bond interactions explain the favourable enthalpic binding contributions revealed by ITC, while the favourable entropic contributions can be attributed to the expelling of structural water molecules from the binding cavities, particularly at the substrates binding sites.
Figure 6. Docking models for the binding conformations of the selected FMNAT hits to the CaFADS FMNAT module. (A) Model of the theoretical placement of substrates. ATP in violet, Mg2+ as a green dot and FMN in yellow. Data from (24). Best docking pose of (B) FADS:241 and FADS:241:242 models (241 in violet, 242 in pink), (C) FADS:271 and FADS:271:272 models (271 in violet, 272 in pink), and (D) FADS:31 (31 in violet, docking corresponds only to the flunixin bioactive part of 31, meglumine is the excipient). Side-chains of key residues are shown as CPK sticks with carbons in wheat. H-bonds are indicated as dashed lines. The protein is shown as a cartoon, having the FMNAT and RFK modules coloured in grey and green, respectively. Docking was performed using Autodock4.2.

Effect of the HTS hits on the RFK and FMNAT activities of SpnFADS
To determine whether the 37 HTS hits were specific for CaFADS or might have effect on other similar bacterial FADS family members, we tested their effects on the RFK and FMNAT activities of the also bimodular and bifunctional SpnFADS. Eleven of the 37 HTS hits inhibited either the RFK or the FMNAT activities of SpnFADS (). However, most compounds exhibited IC50 in the high micromolar range (>65 µM). Only fluvastatin sodium salt (37) for the FMNAT activity, and thonzonium bromide (9) and 27 for the RFK one showed IC50 lower than 10 µM. 37 (IC50 = 7 ± 1 µM) and 9 (IC50 = 6 ± 1 µM) inhibit completely the corresponding activity, but the residual RFK activity with 27 was too high to be considered as a good inhibitor.
Table 5. Effect of selected HTS hits on the RFK and FMNAT activities of SpnFADS.
Effect of selected HTS hits on different bacterial cells
To assess the effect of the HTS hits on the growth of different bacteria, we determined their MIC (). Bacterial cells of C. ammoniagenes, M. tuberculosis and S. pneumoniae were grown in the presence of increasing concentrations of the selected HTS hits. Among the 37 HTS hits, only twelve, six and nine compounds inhibited, respectively, the growth of C. ammoniagenes, M. tuberculosis and S. pneumoniae. Among these, only 9, benzethonium chloride (14), methyl-benzethonium chloride (29), alexidine dihydrochloride (33) and verteporfin (47) showed MIC values for C. ammoniagenes lower than 2 µM, while 17, 24, diethylstilbestrol (32) and dienestrol (35) show values between 2 and 16 µM (). Interestingly, the compounds that presented better properties against the CaFADS FMNAT activity (24, 27 and 31) have a poor growth inhibitory effect on the bacterial cells (24 and 27 have MIC ≈ 32 and 64 µM, respectively, whereas the MIC of 31 was >64 µM). Nonetheless, it is worth to notice that 9, 14, 29, 33 and 47 show in common the considerabl ability to inhibit both of the CaFADS enzymatic activities as well as to greatly affect the C. ammoniagenes growth (, ). Regarding M. tuberculosis growth, sulfasalazine (8), 9, 24 and 29 produced mild effects on cell growth, but only 14 and 33 showed MIC values below 8 µM. summarises the effect of some selected compounds on S. pneumoniae, indicating that 9, 14, 29 and 33 inhibit moderately its growth.
Figure 7. Venn diagrams for the HTS hits effects on C. ammoniagenes and S. pneumoniae. (A) HTS hits that inhibit the RFK (dark grey circle) and FMNAT (medium grey circle) activities of CaFADS as well as the growth of C. ammoniagenes cells (pale grey circle). (B) HTS hits that inhibit the RFK (dark grey circle) and FMNAT (medium grey circle) activities of SpnFADS and the S. pneumoniae cellular growth (pale grey circle). In (A), The hits whose inhibition potency was experimentally assessed in this study (inhibit the FMNAT activity without affecting the RFK one) are highlighted in bold. The hits surrounded by a circle, both in (A) and (B), also inhibit the proliferation of M. tuberculosis.

Table 6. Minimal inhibitory concentration (MIC) of selected HTS hits against different microorganisms.
Discussion
Validation of the HTS protocol
Here we have designed and optimised an enzymatic activity-based HTS protocol to discover specific inhibitors for CaFADS. In this protocol, the direct evaluation of the FMNAT enzymatic activity allows for the selection of species-specific inhibitorsCitation38,Citation39. Our HTS protocol is simple, effective and consumes little protein (1.5 ng/compound against the ∼5 µg/compound required for some differential scanning fluorescence-based HTSs). Our protocol monitors directly the activity of the enzyme that we want to inhibit (), therefore, minimises the number of false positives and PAINS. In addition, because the chemicals in the library are approved drugs, their toxicity in mammalian cells is expected to be limited. The 37 HTS hits obtained (3.6% of the chemical library) were further assayed against the RFK and FMNAT activities of CaFADS. Nine of the HTS hits (when assayed at 250 µM) almost completely inhibited the FMNAT activity without practically affecting the RFK one (). Because the FMNAT activity of CaFADS appeared as a preferred target, due to the different active site regarding eukaryotic enzymes, we further assessed the inhibitory power of these FMNAT hits.
Inhibitors targeting the FMNAT activity of CaFADS
We choose the three FMNAT hits that showed the lowest IC50 and residual activity (24, 27 and 31, 8% of the HTS hits) () to further determine their binding affinities and inhibition mechanisms. Chicago Sky Blue 6B (CSB), here 24, is an allosteric inhibitor of the Macrophage Inhibitor Factor, and shows promising in vivo-effects for the treatment of spinal cord injuryCitation40. Additionally, 24 can act as an anticancer drug through the specific inhibition of Rad 51, as well as a potential resource for Alzheimer disease treatment by inhibiting the binding of β-amyloid to the prion proteinCitation41,Citation42. Furthermore, the inhibition of vesicular glutamate transporters by 24 attenuates expressions of behavioural sensitizationCitation43. In our study, CSB is the most potent inhibitor of the CaFADS FMNAT activity, as shown by its lowest values of residual activity and IC50 (), targeting the free enzyme as well as the FMN-protein and ATP-protein complexes (, Supplementary Figure SD2). Moreover, 24 binds to both enzyme modules being the binding to the FMNAT site highly favourable and enthalpically driven (, ). This is an advantage for an inhibitor, since high binding enthalpy denotes lots of specific interactionsCitation44.
Gossypol, here 27, was used some years ago in China as a masculine contraceptiveCitation45, however, its side effects stopped its pharmacological use. More recently, 27 has demonstrated anticancer effects through the inhibition of antiapoptotic proteins belonging to the Bcl-2 family and of molecules implicated in tumour progressionCitation46,Citation47. Additionally, 27 inhibits the HIV-1 replication in vitroCitation48. 27 is a potent inhibitor of the CaFADS FMNAT activity, as shown by the low IC50 and residual FMNAT activity (), while does not affect the RFK one (, ). This compound competes with ATP for its binding at the FMNAT site (, ). The lower Kd value for 27 when compared with that of FMNCitation29, makes the inhibitor a preferred ligand for CaFADS.
31, or flunixin meglumine, is a non-steroidal anti-inflammatory drug, analgesic and antipyretic extensively used in horses, pigs and cattleCitation49–51. This fact guarantees that 31 can be used securely in mammals. In this study, 31 arises as a mixed inhibitor of the CaFADS FMNAT activity. This is in agreement with our docking model and its small size, envisaging that binding of this compound might coexist with binding of substrates or products in non-competent conformations. Although 31 is the less potent inhibitor of the three here characterised (, ), its binding thermodynamic properties, together with its bio-security in mammals, reveals its potentiality as a drug precursor.
Our docking models supply additional details about the molecular inhibition mechanisms of 24, 27 and 31. Because 27 is a competitive inhibitor it occupies the active site substituting ATP, as demonstrated with the best docking poses (). However, the way in which 24 and 31 inhibit the FMNAT activity is not obvious. The 31 binding conformation () could be affected by the ATP ligand, and, given the small size of 31, it could coexist with ATP in the active site, causing the previously described intricate mechanism. In presence of ATP, the 24 binding conformation should be different to the one of our FADS:241 model, because ATP has to displace 24 from the binding site, given that 24 partially occupies itCitation24. It is probable that with ATP in the active site, and taking into account the large size of this molecule, 24 only interacts with the active site via the N-terminal of the α6n helix (pink molecule in ) and the flexible loop L4n that forms the external and entry part of the FMNAT substrates cavitiesCitation23,Citation24. In this way, the 24 sulphates would be able to coordinate the magnesium ion to potentially induce a change in the ATP phosphates orientation, negatively affecting their orientation for the FMNAT activity as well as the entry and exit of the reaction ligands.
Inhibition of other bacterial FADS by CaFADS FMNAT hits
S. pneumoniae causes more than 25% of the cases of community-acquired pneumoniaCitation52, generating more deaths than any other vaccine-preventable bacterial disease. M. tuberculosis causes tuberculosis, the most common cause of death among infectious diseasesCitation53. SpnFADS has as similar native structure to CaFADS (Supplementary Figure SD1), while the MtFADS sequence shows 45% identity with CaFADS and 59% of conservationCitation30. Since the attempts to produce stable purified MtFADS have so far failed, we considered CaFADS a good model for MtFADS, as reported for other proteins of these two generaCitation54. Thus, we tested the effect of the CaFADS HTS hits on the RFK and FMNAT activities of SpnFADS. We can find that only 30% of the HTS hits have inhibitory effects on SpnFADS, and the high values of residual activities and IC50 reveal that they are worse inhibitors for SpnFADS (). Nevertheless, among the HTS hits, 9, 27, 37 and 43 are interesting inhibitors of SpnFADS (). 27 and 43, which did not inhibit the CaFADS RFK activity (), have an important effect on the SpnFADS one. 9, inhibits both SpnFADS activities (). This compound is a monocationic detergent that has been commonly used in cortisporin-TC ear drops to help penetration of active ingredients through cellular debris. Additionally, 9 inhibits vacuolar ATPase, showing cytotoxic effects at concentrations higher than 10 µMCitation55. It is also an inhibitor of the RANK-L induced osteoclast formationCitation56. 37, inhibited both CaFADS activities, but only affects the FMNAT one in SpnFADS. 37 inhibits the HMG-CoA reductase, being used to treat hypercholesterolemiaCitation57. 37 has positive effects in myocardial fibrosis by favouring ACE2 expression, and also modestly inhibits replication of the hepatitis C virusCitation58,Citation59. 43 is used as analgesic (inhibits anandamide hydrolase in neurons) and as anti-inflammatoryCitation60,Citation61, acting as a no selective cyclooxygenase inhibitor. Additionally, it inhibits NF-κβ in activated monocytes, being a promising drug for the treatment of rheumatoid arthritisCitation62. Regarding the three FMNAT hits of CaFADS: 24 has a poor inhibitory effect on SpnFADS (), which might be of interest as selective inhibitor; 27 inhibits both CaFADS and SpnFADS activities ( and ), appearing as a broad inhibitor of FADS; and 31 has no effect on SpnFADS (), indicating the specificity of this compound for CaFADS.
Recent studiesCitation23,Citation28,Citation63–65 revealed important differences in catalysis among bifunctional FADS, probably related to dissimilarities in the active site conformation during catalysis. This is consistent with the differential inhibitory mechanisms of the HTS hits against CaFADS and SpnFADS, as observed in the present work. Such mechanistic variations could determine the binding or the inhibitory capability of the hits. These observations highlight the potentiality of our method to find selective drugs targeting a specific protein of a particular microorganism. The development of such species-selective drugs is of great importance for the treatment of infections by avoiding undesired side-effects on normal microbiota of the hostCitation66, and for minimising the selection of resistant bacterial strains.
Antimicrobial activity of the CaFADS FMNAT hits
Finally, we also tested the inhibitory activity of the best FMNAT hits on the growth of C. ammoniagenes, S. pneumoniae and M. tuberculosis cultures. 24 shows moderate effects on the C. ammoniagenes and M. tuberculosis growth (, ). However, it does not inhibit the pneumococci growth. 27 and 31 do not inhibit the growth of C. ammoniagenes, S. pneumoniae or M. tuberculosis cells. This might be due to their inability to enter in the bacterial cell, or because efflux pumps eject them once in the bacterial cytoplasm. Tools to favour their bactericide effects can be obtained by deriving second generation hits, using vehiculization systems to move drugs across the membrane, or using efflux pumps inhibitorsCitation67–69. In this context, we remark that inhibition of FAD synthesis in M. tuberculosis could have an immediate impact in current antituberculosis drug discovery programmes. Benzothiazinones are antituberculosis compounds that block arabinan synthesis by targeting the flavoprotein decaprenylphosphoryl-β-D-ribose 2'-epimerase DprE1Citation70. It is expected that the antituberculosis activity of benzothiazinones, which are currently in phase I clinical trialsCitation4, could be enhanced by FADS inhibition, in a synergistic manner. Among the other FMNAT hits, only 15, 17 and 31 show mild inhibitory activity on the growth of C. ammoniagenes, but do not have an effect on S. pneumoniae and M. tuberculosis cultures ().
We find five HTS hits (9, 14, 29, 33 and 47) as strong inhibitors of the C. ammoniagenes growth (, ). These five HTS hits also inhibit the growth of S. pneumoniae (, ), while only the first four mildly affect M. tuberculosis. Noticeably, these five HTS hits are good inhibitors of both CaFADS activities (, ). 9 and 29 also appear as potent inhibitors of the SpnFADS RFK and/or FMNAT activities. The effect of the other three compounds as SpnFADS inhibitors is milder, suggesting mechanisms that do not involve FADS in preventing cell proliferation. Overall, our results suggest that targeting both activities of bifunctional FADSs can be a strategy in the discovery of new antibacterial drugs. Thus, the non-selective antimicrobial properties of 9 and 29 seem interesting tools to be explored.
In this context, and despite all compounds in the library are approved by FAD and EMA, it remains for future studies to test the effect of the most promising compounds on the homologous human proteins and cells. In addition, future studies should also focus on the improvement of these compounds regarding potency, selectivity, pharmacokinetics and drug-likeness.
Conclusions
The FMNAT activity of bifunctional FADS enzymes is a potential antimicrobial target for drug discovery. The transformation of FMN into FAD is performed by different catalytic mechanisms in prokaryotes and eukaryotes, and the FMN and FAD deficiency inactivates an important number of flavoproteins. In this work, we have optimised an activity-based HTS that can be used to discover new antibacterial drugs, targeting the RFK and/or FMNAT activities of bifunctional FADSs. Our method allows identifying bacterial FADS inhibitors with different levels of selectivity regarding the inhibition of bacterial growth. The method is fast, effective and requires small protein quantities. We have confirmed that bacterial FADS are promising species-selective drug targets. Among the 1240 compounds from the Prestwick Chemical Library®, 37 inhibited CaFADS, and three were potent inhibitors of its FMNAT (but not of the RFK) activity. Two of these compounds were not species-selective because they also affected SpnFADS, but the third one, 31, was selective for CaFADS versus SpnFADS. These three compounds are promising as non-selective or selective inhibitors at the enzyme level. However, they do not produce observable antimicrobial effects, suggesting that they do not reach inhibitory concentrations at the intracellular level, possibly due to a poor uptake, efficient efflux or in vivo fast degradation. Nevertheless, some HTS hits show good antimicrobial properties, probably due to the inhibition of both RFK and FMNAT activities of FADS.
IENZ_1411910_Supplementary_Material.pdf
Download PDF (391.2 KB)Acknowledgements
The authors thank Dr J. Sancho for access to the chemical library, Dr A. Velázquez-Campoy for support on ITC experiments and both of them for helpful discussions. I.L. and P.C. were supported by Colciencias and University of Antioquia (Colombia) and the Max Planck society (Germany).
Disclosure statement
The authors report no conflicts of interest.
Additional information
Funding
References
- Spellberg B, Powers JH, Brass EP, et al. Trends in antimicrobial drug development: implications for the future. Clin Infect Dis 2004;38:1279–86.
- Conly J, Johnston B. Where are all the new antibiotics? The new antibiotic paradox. Can J Infect Dis Med Microbiol 2005;16:159–60.
- Walsh CT, Wencewicz TA. Prospects for new antibiotics: a molecule-centered perspective. J Antibiot (Tokyo) 2014;67:7–22.
- WHO. Antibacterial agents in clinical development: an analysis of the antibacterial clinical development pipeline, including tuberculosis. WHO/EMP/IAU/2017.11 ed. World Health Organization: Geneva; 2017.
- Brown ED, Wright GD. Antibacterial drug discovery in the resistance era. Nature 2016;529:336–43.
- Lienhart WD, Gudipati V, Macheroux P. The human flavoproteome. Arch Biochem Biophys 2013;535:150–62.
- Gudipati V, Koch K, Lienhart WD, Macheroux P. The flavoproteome of the yeast Saccharomyces cerevisiae. Biochim Biophys Acta 2014;1844:535–44.
- Barile M, Giancaspero TA, Leone P, et al. Riboflavin transport and metabolism in humans. J Inherit Metab Dis 2016;39:545–57.
- Gross E, Kastner DB, Kaiser CA, Fass D. Structure of Ero1p, source of disulfide bonds for oxidative protein folding in the cell. Cell 2004;117:601–10.
- Parsons HG, Dias VC. Intramitochondrial fatty acid metabolism: riboflavin deficiency and energy production. Biochem Cell Biol 1991;69:490–7.
- Myllykallio H, Lipowski G, Leduc D, et al. An alternative flavin-dependent mechanism for thymidylate synthesis. Science 2002;297:105–7.
- Susin SA, Lorenzo HK, Zamzami N, et al. Molecular characterization of mitochondrial apoptosis-inducing factor. Nature 1999;397:441–6.
- Sassetti CM, Boyd DH, Rubin EJ. Genes required for mycobacterial growth defined by high density mutagenesis. Mol Microbiol 2003;48:77–84.
- Griffin JE, Gawronski JD, Dejesus MA, et al. High-resolution phenotypic profiling defines genes essential for mycobacterial growth and cholesterol catabolism. PLoS Pathog 2011;7:e1002251.
- Serrano A, Ferreira P, Martínez-Júlvez M, Medina M. The prokaryotic FAD synthetase family: a potential drug target. Curr Pharm Des 2013;19:2637–48.
- Barile M, Passarella S, Bertoldi A, Quagliariello E. Flavin adenine dinucleotide synthesis in isolated rat liver mitochondria caused by imported flavin mononucleotide. Arch Biochem Biophys 1993;305:442–7.
- Serrano A, Frago S, Velázquez-Campoy A, Medina M. Role of key residues at the flavin mononucleotide (FMN):adenylyltransferase catalytic site of the bifunctional riboflavin kinase/flavin adenine dinucleotide (FAD) synthetase from Corynebacterium ammoniagenes. Int J Mol Sci 2012;13:14492–517.
- Efimov I, Kuusk V, Zhang X, McIntire WS. Proposed steady-state kinetic mechanism for Corynebacterium ammoniagenes FAD synthetase produced by Escherichia coli. Biochemistry 1998;37:9716–23.
- Mack M, van Loon AP, Hohmann HP. Regulation of riboflavin biosynthesis in Bacillus subtilis is affected by the activity of the flavokinase/flavin adenine dinucleotide synthetase encoded by ribC. J Bacteriol 1998;180:950–5.
- Herguedas B, Martínez-Júlvez M, Frago S, et al. Crystallization and preliminary X-ray diffraction studies of FAD synthetase from Corynebacterium ammoniagenes. Acta Crystallogr Sect F Struct Biol Cryst Commun 2009;65:1285–8.
- Wang W, Kim R, Yokota H, Kim SH. Crystal structure of flavin binding to FAD synthetase of Thermotoga maritima. Proteins 2005;58:246–8.
- Wang W, Kim R, Jancarik J, et al. Crystal structure of a flavin-binding protein from Thermotoga maritima. Proteins 2003;52:633–5.
- Sebastián M, Lira-Navarrete E, Serrano A, et al. The FAD synthetase from the human pathogen Streptococcus pneumoniae: a bifunctional enzyme exhibiting activity-dependent redox requirements. Sci Rep 2017;7:7609.
- Herguedas B, Martinez-Julvez M, Frago S, et al. Oligomeric state in the crystal structure of modular FAD synthetase provides insights into its sequential catalysis in prokaryotes. J Mol Biol 2010;400:218–30.
- Giancaspero TA, Galluccio M, Miccolis A, et al. Human FAD synthase is a bi-functional enzyme with a FAD hydrolase activity in the molybdopterin binding domain. Biochem Biophys Res Commun 2015;465:443–9.
- Serrano A, Sebastian M, Arilla-Luna S, et al. Quaternary organization in a bifunctional prokaryotic FAD synthetase: involvement of an arginine at its adenylyltransferase module on the riboflavin kinase activity. Biochim Biophys Acta 2015;1854:897–906.
- Serrano A, Frago S, Herguedas B, et al. Key residues at the riboflavin kinase catalytic site of the bifunctional riboflavin kinase/FMN adenylyltransferase from Corynebacterium ammoniagenes. Cell Biochem Biophys 2013;65:57–68.
- Herguedas B, Lans I, Sebastián M, et al. Structural insights into the synthesis of FMN in prokaryotic organisms. Acta Crystallogr D Biol Crystallogr 2015;71:2526–42.
- Frago S, Velázquez-Campoy A, Medina M. The puzzle of ligand binding to Corynebacterium ammoniagenes FAD synthetase. J Biol Chem 2009;284:6610–19.
- Frago S, Martínez-Júlvez M, Serrano A, Medina M. Structural analysis of FAD synthetase from Corynebacterium ammoniagenes. BMC Microbiol 2008;8:160.
- Lagorce D, Oliveira N, Miteva MA, Villoutreix BO. Pan-assay interference compounds (PAINS) that may not be too painful for chemical biology projects. Drug Discov Today 2017;22:1131–3.
- Cosconati S, Forli S, Perryman AL, et al. Virtual screening with AutoDock: theory and practice. Expert Opin Drug Discov 2010;5:597–607.
- Morris GM, Huey R, Lindstrom W, et al. AutoDock4 and AutoDockTools4: automated docking with selective receptor flexibility. J Comput Chem 2009;30:2785–91.
- Forli S, Olson AJ. A force field with discrete displaceable waters and desolvation entropy for hydrated ligand docking. J Med Chem 2012;55:623–38.
- Frisch MJ, Trucks GW, Schlegel HB, et al. Gaussian 09. Wallingford, CT: Gaussian Inc.; 2016.
- Palomino JC, Martin A, Camacho M, et al. Resazurin microtiter assay plate: simple and inexpensive method for detection of drug resistance in Mycobacterium tuberculosis. Antimicrob Agents Chemother 2002;46:2720–2.
- Olsson TS, Williams MA, Pitt WR, Ladbury JE. The thermodynamics of protein-ligand interaction and solvation: insights for ligand design. J Mol Biol 2008;384:1002–17.
- Deu E, Yang Z, Wang F, et al. Use of activity-based probes to develop high throughput screening assays that can be performed in complex cell extracts. PLoS One 2010;5:e11985.
- Zhang G. Protease assays. Available from: http://www.ncbi.nlm.nih.gov/books/NBK92006/
- Saxena T, Loomis KH, Pai SB, et al. Nanocarrier-mediated inhibition of macrophage migration inhibitory factor attenuates secondary injury after spinal cord injury. ACS Nano 2015;9:1492–505.
- Normand A, Rivière E, Renodon-Cornière A. Identification and characterization of human Rad51 inhibitors by screening of an existing drug library. Biochem Pharmacol 2014;91:293–300.
- Risse E, Nicoll AJ, Taylor WA, et al. Identification of a compound that disrupts binding of Amyloid-β to the prion protein using a novel fluorescence-based assay. J Biol Chem 2015;290:17020–8.
- He Z, Yan L, Yong Z, et al. Chicago sky blue 6B, a vesicular glutamate transporters inhibitor, attenuates methamphetamine-induced hyperactivity and behavioral sensitization in mice. Behav Brain Res 2013;239:172–6.
- Klebe G. Applying thermodynamic profiling in lead finding and optimization. Nat Rev Drug Discov 2015;14:95–110.
- Soufir JC. Hormonal, chemical and thermal inhibition of spermatogenesis: contribution of French teams to international data with the aim of developing male contraception in France. Basic Clin Androl 2017;27:3.
- Ferdek PE, Jakubowska MA, Nicolaou P, et al. BH3 mimetic-elicited Ca(2+) signals in pancreatic acinar cells are dependent on Bax and can be reduced by Ca(2+)-like peptides. Cell Death Dis 2017;8:e2640.
- Xiong J, Li J, Yang Q, et al. Gossypol has anti-cancer effects by dual-targeting MDM2 and VEGF in human breast cancer. Breast Cancer Res 2017;19:27
- Polsky B, Segal SJ, Baron PA, et al. Inactivation of human immunodeficiency virus in vitro by gossypol. Contraception 1989;39:579–87.
- Newby NC, Leslie KE, Dingwell HD, et al. The effects of periparturient administration of flunixin meglumine on the health and production of dairy cattle. J Dairy Sci 2017;100:582–7.
- Kleinhenz MD, Van Engen NK, Gorden PJ, et al. The pharmacokinetics of transdermal flunixin meglumine in Holstein calves. J Vet Pharmacol Ther 2016;39:612–15.
- Burkett BN, Thomason JM, Hurdle HM, et al. Effects of firocoxib, flunixin meglumine, and phenylbutazone on platelet function and thromboxane synthesis in healthy horses. Vet Surg 2016;45:1087–94.
- Torres A, Blasi F, Peetermans WE, et al. The aetiology and antibiotic management of community-acquired pneumonia in adults in Europe: a literature review. Eur J Clin Microbiol Infect Dis 2014;33:1065–79.
- WHO. Global Tuberculosis Report. Geneva: WHO; 2016.
- Seidel M, Alderwick LJ, Sahm H, et al. Topology and mutational analysis of the single Emb arabinofuranosyltransferase of Corynebacterium glutamicum as a model of Emb proteins of Mycobacterium tuberculosis. Glycobiology 2007;17:210–19.
- Chan CY, Prudom C, Raines SM, et al. Inhibitors of V-ATPase proton transport reveal uncoupling functions of tether linking cytosolic and membrane domains of V0 subunit a (Vph1p). J Biol Chem 2012;287:10236–50.
- Zhu X, Gao JJ, Landao-Bassonga E, et al. Thonzonium bromide inhibits RANKL-induced osteoclast formation and bone resorption in vitro and prevents LPS-induced bone loss in vivo. Biochem Pharmacol 2016;104:118–30.
- Weng TC, Yang YH, Lin SJ, Tai SH. A systematic review and meta-analysis on the therapeutic equivalence of statins. J Clin Pharm Ther 2010;35:139–51.
- Shin YH, Min JJ, Lee JH, et al. The effect of fluvastatin on cardiac fibrosis and angiotensin-converting enzyme-2 expression in glucose-controlled diabetic rat hearts. Heart Vessels 2017;32:618–27.
- Bader T, Fazili J, Madhoun M, et al. Fluvastatin inhibits hepatitis C replication in humans. Am J Gastroenterol 2008;103:1383–9.
- Dallegri F, Bertolotto M, Ottonello L. A review of the emerging profile of the anti-inflammatory drug oxaprozin. Expert Opin Pharmacother 2005;6:777–85.
- Kara IM, Polat S, Inci MF, et al. Analgesic and anti-inflammatory effects of oxaprozin and naproxen sodium after removal of impacted lower third molars: a randomized, double-blind, placebo-controlled crossover study. J Oral Maxillofac Surg 2010;68:1018–24.
- Montecucco F, Bertolotto M, Ottonello L, et al. Oxaprozin-induced apoptosis on CD40 ligand-treated human primary monocytes is associated with the modulation of defined intracellular pathways. J Biomed Biotechnol 2009;2009:478785.
- Grill S, Busenbender S, Pfeiffer M, et al. The bifunctional flavokinase/flavin adenine dinucleotide synthetase from Streptomyces davawensis produces inactive flavin cofactors and is not involved in resistance to the antibiotic roseoflavin. J Bacteriol 2008;190:1546–53.
- Matern A, Pedrolli D, Groszhennig S, et al. Uptake and metabolism of antibiotics roseoflavin and 8-demethyl-8-aminoriboflavin in riboflavin-auxotrophic listeria monocytogenes. J Bacteriol 2016;198:3233–43.
- Marcuello C, Arilla-Luna S, Medina M, Lostao A. Detection of a quaternary organization into dimer of trimers of Corynebacterium ammoniagenes FAD synthetase at the single-molecule level and at the in cell level. Biochim Biophys Acta 2013;1834:665–76.
- Lewis K. Platforms for antibiotic discovery. Nat Rev Drug Discov 2013;12:371–87.
- Stermitz FR, Lorenz P, Tawara JN, et al. Synergy in a medicinal plant: antimicrobial action of berberine potentiated by 5'-methoxyhydnocarpin, a multidrug pump inhibitor. Proc Natl Acad Sci USA 2000;97:1433–7.
- Rodrigues L, Parish T, Balganesh M, Ainsa JA. Antituberculosis drugs: reducing efflux = increasing activity. Drug Discov Today 2017;22:592–99.
- Ladbury JE, Klebe G, Freire E. Adding calorimetric data to decision making in lead discovery: a hot tip. Nat Rev Drug Discov 2010;9:23–7.
- Makarov V, Manina G, Mikusova K, et al. Benzothiazinones kill Mycobacterium tuberculosis by blocking Arabinan synthesis. Science 2009;324:801–4.