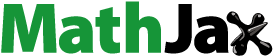
Abstract
α-Amylase has been considered an important therapeutic target for the management of type 2 diabetes mellitus (T2DM), decreasing postprandial hyperglycaemia (PPHG). In the present work, a panel of 40 structurally related flavonoids was tested, concerning their ability to inhibit α-amylase activity, using a microanalysis screening system, an inhibitory kinetic analysis and molecular docking calculations. From the obtained results, it was possible to observe that the flavone with a -Cl ion at 3-position of C-ring, an –OH group at 3′- and 4′- positions of B-ring and at 5- and 7- positions of A-ring and the C2 = C3 double bond, was the most active tested flavonoid, through competitive inhibition. In conclusion, some of the tested flavonoids have shown promising inhibition of α-amylase and may be considered as possible alternatives to the modulation of T2DM.
Graphical Abstract

Introduction
α-1,4-Glucan-4-glucanohydrolases (EC 3.2.1.1.), belonging to the family 13 of glycoside hydrolases (GH) and found in saliva and pancreatic juice, are known by the common name of α-amylaseCitation1,Citation2. Both are isozymes expressed respectively from genes AMY1 and AMY2 and are composed of 496 amino acid residues in a single polypeptide chain, presenting a 97% identity of the amino acids sequence and a 92% similarity of the residues in the catalytic domainsCitation3–5. They have three different domains (A, B and C) and a common calcium-binding site that stabilizes the interface between the central A domain and the variable B domainCitation6,Citation7. The catalytic triad (Asp, Asp, Glu) is present in the A domain and the active site is located in a long cleft between the carboxyl end of both A and B domains. The C domain consists of a β-sheet structure linked to the A domain by a simple polypeptide chainCitation5.
α-Amylase catalyzes the initial hydrolysis of starch and other carbohydrate polymers into shorter oligosaccharides through cleavage of α-1,4- bondsCitation5,Citation7,Citation8. The salivary isozyme provides an initial partial cleavage into shorter oligomers (10–30%)Citation7. Once these partially digested saccharides reach the gut, they are extensively hydrolyzed by the α-amylase synthesized in the pancreas and excreted in the lumen into smaller oligosaccharides, such as maltose, maltotriose and α-limit dextrins ()Citation3. These sugars are finally broken down into glucose by the intestinal brush border α-glucosidases, which is in turn absorbed from the intestinal mucosa into the portal blood, by means of the glucose transporter (GLUT2) and sodium-glucose co-transporter 1 (SGLT1), leading to postprandial hyperglycaemia (PPHG)Citation2. Impaired regulation of PPHG constitutes a common feature in type 2 diabetes mellitus (T2DM), the most prevalent form of diabetes, accounting for about 90% of all diabetes casesCitation2,Citation9,Citation10.
Figure 1. Schematic representation of the pancreatic α-amylase and α-glucosidase activity. After a meal, α-amylase synthesized in pancreas and released in the duodenum, catalyzes the hydrolysis of α-1,4 glycosidic linkages in partially hydrolyzed starch (amylopectin and amylose). From this reaction, intermediate unbranched, such as maltose and maltotriose, and branched (α-limit dextrins) oligosaccharides are formed. α-Glucosidase present in the brush border of the intestinal epithelium (enterocytes) is responsible for the final step of carbohydrates digestion, prior to their absorption. This enzyme converts the disaccharides and oligosaccharides into glucose, which is then transported by sodium/glucose co-transporter 1 (SGLT1) from the intestinal lumen to the cytosol of enterocytes. In turn, glucose transporter 2 (GLUT2), found in the basolateral membrane of enterocytes, transports glucose from cytosol to blood via facilitated diffusion.

The pancreatic α-amylase activity has been targeted for inhibition by means of the so-called starch blockers in order to mitigate PPHGCitation1,Citation3. Acarbose is the most widely prescribed α-amylase inhibitor, and in spite of its efficiency in the control of PPHG, the administration of this drug is associated with gastrointestinal adverse effects in diabetic patients, namely abdominal distention, flatulence and diarrhoeaCitation11. Thus, the search and development of new effective and safer agents, able to control glucose levels is of high importance for the management of T2DMCitation12.
In the last few years, the activity of flavonoids has been studied even up to clinical trials concerning the modulation of diabetes in humansCitation13,Citation14. Promising inhibitory potential against important targets related with the T2DM pathophysiology was evidenced regarding the inhibition of isolated enzymes such as α-glucosidaseCitation15 and protein tyrosine phosphatase 1B (PTP1B)Citation16. Some bibliographic reviews compile the obtained results of the antidiabetic activity of flavonoidsCitation17–20. Further isolated studies also describe flavonoids as α-amylase inhibitors, such as apigeninCitation21,Citation22, luteolinCitation21,Citation23, kaempferolCitation21,Citation22, naringeninCitation21, quercetinCitation21,Citation22,Citation24,Citation25, myricetinCitation21,Citation22,Citation25, chrysinCitation22 and baicaleinCitation21,Citation22. Nevertheless, significant differences in the experimental conditions among studies, such as the source and concentrations of enzyme and substrate and the different incubation times applied, turn difficult the establishment of a reliable structure-activity relationship. To fill this gap, a panel of 40 structurally related flavonoids (), most of them studied for the first time, was assessed regarding their inhibitory activity against α-amylase. This study comprehends an in vitro microanalysis screening system, modelling of kinetics inhibition and an in silico molecular docking analysis.
Materials and methods
Chemicals
α-Amylase from porcine pancreas, 2-chloro-4-nitrophenyl-α-D-maltotrioside (CNPG3), acarbose, DMSO, NaHPO4, Na2HPO4, flavonoids D3 (baicalein), D4 (apigenin), D6 (kaempferol), D7 (luteolin), D8 (quercetin), D9 (myricetin), D10 (morin), D17 (acacetin), D19 (rutin), E1 (naringenin), E2 (eriodictyol) and E3 (taxifolin) were obtained from Sigma-Aldrich Co. LLC (St. Louis, MO). The following flavonoids were obtained from Indofine Chemical Company, Inc. (Hillsborough, NJ): A1, A2, A3, A4, A5, A6, B1, B2, B3, B4, C1, C2, C3, C4, C5, C6, C7, C8, D1 (chrysin), D2 (galangin) and D5. The flavonoids D11, D12, D13, D14, D15, D16and D18 were synthesized according to previous companion papersCitation26,Citation27.
In vitro pancreatic α-amylase inhibition assay
The evaluation of inhibitory effect on α-amylase activity was based on the method described by Trinh et al.Citation28, with slight modifications. In each assay, the α-amylase mediated hydrolysis of the substrate CNPG3 into 2-chloro-nitrophenol (CNP), 2-chloro-4-nitrophenyl-α-D-maltoside (CNPG2), maltotriose and glucose was monitored. The initial rate of CNP generation, measured spectrophotometrically at 405 nm, is proportional to the concentration of α-amylase present. In brief, in a 96-well plate, the enzyme (0.2 U/mL), dissolved in 20 mM phosphate buffer (20 mM Na2HPO4 and 7 mM NaCl, pH 6.8) was exposed to the flavonoids under study (0–200 µM), dissolved in DMSO [final concentration of DMSO of 4.5% (v/v)]. After an incubation time of 10 min, at 37 °C, the reaction was started by the addition of CNPG3 (0.5 mM), dissolved in 20 mM phosphate buffer (pH 6.8) and monitored along 30 min, at 37 °C, in a microplate reader (Synergy HT, BIO-TEK), with the wavelength set to 405 nm. The concentration of α-amylase and the tested flavonoids was determined before the addition of the substrate. Increasing absorbance values obtained within the 5 to 30 min interval (slope) served for the calculation of catalytic rates and the results in the form of inhibition percentages, representing at least three independent experiments. Acarbose (0–16 µM) was used as positive control. The amount of DMSO used did not interfere with the assay. The results of the in vitro inhibitory activity of flavonoids against the pancreatic α-amylase activity are expressed as mean ± standard error of mean (SEM). Statistical comparison between the active flavonoids was estimated by applying the one-way analysis of variance (ANOVA). Differences were considered to be significant at p values lower than 0.05. All the statistical analysis were performed using GraphPad Prism™ (version 7.0; GraphPad Software).
Inhibitory kinetics of α-amylase
The estimation of kinetic parameters and prediction of the actual mechanism of inhibition was performed by means of Microsoft Office Excel™ spreadsheets and using the Solver supplement add-in, according to Bezerra et al.Citation29 and Dias et al.Citation30. Therefore, the kinetics of conversion of CNPG3 by α-amylase in each microplate well was fitted by nonlinear least squares regressionCitation31 using the general model for enzymatic reactions translated by the EquationEquation (1)(1)
(1) , and each one of its simplifications regarding the different types of inhibition:
(1)
(1) where vinic and Vmax represent respectively the initial velocity of formation of absorbing CNP in µmol/min and the maximum achievable velocity when for the 0.2 U/mL of enzyme used all catalytic sites are saturated by the substrate, S is the CNPG3 concentration in mM, Km is the Michaelis–Menten constant in mM and Kic, Kiu are the constants for competitive and uncompetitive inhibition processes expressed in µM−1.
To study the enzyme kinetics, nonlinear regression was applied to the nontransformed data for the most active flavonoids of each group: B4 (0–200 µM), C5 (0–100 µM), D11 (0–100 µM) and acarbose (0–2 µM). For each concentration of inhibitor, three concentrations of the substrate CNPG3 were tested: 0.25, 0.5 and 1 mM. The obtained results represent at least three independent experiments.
For each tested condition, the best guesses for equation parameters were thrown by Solver tool after iterative minimization of the sum of squared residuals between the experimental values of Vmax and the corresponding counterparts estimated according to each model used. Hence, the values obtained for the parameters of the simplest model (without inhibition) were used as initial values, proceeding to competitive inhibition, noncompetitive inhibition, uncompetitive inhibition and finishing with the more complex model, mixed inhibition. The actual mechanism of inhibition was established after comparison and discrimination between the models with a different number of parameters applying the extra sum-of-square F testCitation32 and the Akaike information criterion (AIC) testCitation33.
The jackknife procedure was applied to determinate the error of the kinetic constant values. It consisted of the calculation of standard deviation of all estimates guessed by Solver when each experimental data point was in turn drawn from the initial setCitation34.
Molecular docking analysis
Seventeen entries in the Protein Data Bank (PDB) correspond to Sus Scrofa α-amylase (E.C. 3.2.1.1.). From these, it was chosen the PDB entry 1HX0Citation35 corresponding to the highest resolution X-ray structure (1.38). This structure was co-crystalized with a trisaccharide inhibitor K2, derived from acarbose after α-amylase cleaved one glucose unit at the reducing end. The crystal structure shown two α-1,4-linked K2 molecules, condensed in situ. The K2 unit bound at the active site was used to compare the binding mode of the tested flavonoids and as a template to model the substrate for receptor minimization and conformational sampling.
The protonation states of the enzyme were predicted using the Protoss serverCitation36. Accordingly, Glu233 and Glu390 were modelled in the neutral form and His101, His201 and His215 were modelled in the positively charged for, with both side chain nitrogen atoms protonated. All other residues were protonated according to their pKa, at pH = 7.
From the hexasaccharide compound present in 1HXO (resultant of two α-1,4-linked K2 molecules), the substrate maltohexose was modelled. On this system, Molecular Mechanics minimization was performed, followed by 20 ns of classical molecular dynamics (MD) simulation to sample receptor conformations. This protocol was performed within the AMBER 12 suiteCitation37 using the ff99SBCitation38 force field for the protein and GLYCAM06Citation39 force field for maltohexose. Further details for the energy minimization and MD simulation can be found in Supporting Information.
To choose and validate the conformation of the receptor for the docking protocol several receptor conformations were evaluated: the X-ray conformation, the conformation of the receptor minimized with maltohexose and four representative conformations of the clustered MD simulation. The suitability of each conformation was assessed by evaluating if the docking of the inhibitor acarbose in the active site reproduced the X-ray conformation and how well the ranking of the tested flavonoids with the lowest IC50 was reproduced. The conformation of the receptor with the best performance was obtained after 3.3 ns of simulation.
The binding mode of the selected flavonoids was predicted by means of the AutoDock VinaCitation40. The binding poses were generated using default parameters (see Supporting Information for details), such as the exhaustiveness of the global search (8) or the maximum number of output poses (9). The search space was confined to a box of 19 Å × 12 Å × 17 Å centered on the site occupied by the glycosidic oxygen between the –1 and –2 glucose units of maltohexose. The receptor was treated as a rigid body and prepared using openbabelCitation41 for atom-typing and charge assignment using Gasteiger–Marsili chargesCitation42. The atom-types and charges for the tested flavonoids were attributed the same way.
Subsequently, the poses predicted by AutoDock Vina for each flavonoid were scored using the scoring function GoldScore within the GOLD software suiteCitation43, using the standard parameters for a “Rescoring Run” with local optimization.
Results
In vitro pancreatic α-amylase inhibition assay
The tested flavonoids were divided into five groups (A–E), according to their substitution pattern. The substitution pattern of flavonoids from the A group differs at 3-position of the C-ring and at 3′- and 4′-positions of the B-ring; flavones from B group have an –OH group at 5-position of the A-ring and different substitutions at 3′- and 4′-positions of the B-ring; flavonoids from C group show –OH, –OMe and/or –OBn groups at 3-position of the C-ring, at 3′- and 4′-positions of the B-ring and at 7- and 8-position of the A-ring; flavonoids from D group present –OH substitutions at 5- and 7- positions of the A-ring and also present –OH, –OMe and/or –Cl substitutions at 3-position of the C-ring, at 2′-, 3′-, 4′- and 5′-positions of the B-ring and at 6- and 8-positions of the A-ring; flavanones from E group have –OH groups at 5- and 7-positions of the A-ring and their substitution pattern differs in the presence or absence of –OH groups at 3′- and 4′-positions of the B-ring ().
Table 1. Inhibitory activity of flavonoids against porcine pancreatic α-amylase (IC50, µM ± SEM).
No relevant inhibitory activities were observed, up to the highest tested concentration (200 µM), for the flavones belonging to A group with different substitutions in 3-position of C-ring and in 3′- and 4′-positions of B-ring.
For flavones of B group, with an –OH group at 5-position of A-ring and different substitutions in 3′- and 4′-positions of B-ring, the most effective was B4, presenting an IC50 value of 148 ± 5 µM. This result demonstrates that the presence of the catechol group at B-ring translated in a relatively slight increase of the inhibitory effect over α-amylase catalysis when compared to the other flavones of this group. The presence of an –OH group at 5-position of A-ring also seems to contribute to such effect, since flavone B4 was more active than flavone A4 (also with 3′- and 4′–OH in B-ring, but without 5–OH in A-ring).
From C group, which includes flavonoids with different substitutions at 3-position of C-ring, at 3′ and 4′ positions of B-ring and at 7 and 8 positions of A-ring, the most active flavonoid was compound C5, tested here for the first time, with an IC50 value of 59 ± 4 µM. It seems that the presence of –OH groups at 3-position of C-ring, at 4′-position of B-ring and at 7-position of A-ring, is accompanied by a significant inhibitory effect on α-amylase. On the other hand, the presence of -OMe groups in the flavonoid scaffold (C7, C8) did not bring any advantage for the intended effect, since the initial conversion rates of the CNPG3 substrates remained unchanged even for the highest tested concentration (200 µM).
All members of D group have –OH groups at 5- and 7-positions of A-ring and the substitution pattern varies according to the presence or absence of –Cl ions, –OH and –OMe groups in their structure. The most effective flavonoid found was the chlorinated flavone D11, with an IC50 value of 44 ± 3 µM. Thus, the presence of a –Cl ion at 3-position of C-ring, as well as the presence of a catechol in B-ring and –OH groups at 5- and 7-positions of A-ring, showed to be crucial for the inhibition of α-amylase activity. Comparing flavonoid D11 with D8 (quercetin) (IC50= 138 ± 5 µM), it is clear that the substitution of the Cl- ion for an OH-group at 3-position of C-ring results in a significant decrease of the corresponding inhibitory activity. It was also observed that the presence of a -OMe group at 3′- position of B-ring [D16 (chrysoeriol)] slightly improves the ability to inhibit α-amylase activity (IC50= 192 ± 7 µM), comparing with D17 (acacetin), which presents a -OMe group at 4′-position of B-ring. An increase of the number of -OMe groups in the structure of flavonoids is not favourable for the intended effect, as it can be concluded from a comparison of D16 (chrysoeriol) with D18, the later with an extra -OMe group at 4′-position of B-ring. A glycosylated flavonoid, D19 (rutin), was also tested, but no activity was found up to the highest tested concentration (200 µM).
The studied flavanones of E group, E1 (naringenin), E2 (eriodictyol) and E3 (taxifolin) were not able to inhibit α-amylase activity, at the maximum tested concentration (200 µM). These results demonstrate that the presence of C2 = C3 double bond is very relevant for the intended effect.
Inhibitory kinetics of α-amylase
In accordance with the described results, the most effective flavonoids of B, C and D groups, B4, C5 and D11, respectively, and the positive control, acarbose, were henceforth selected for their type of inhibition calculation. No flavonoids from A and E group were tested, due to their lack of inhibitory activity.
By comparing the sum of the square errors values among the different enzyme inhibition models and, for models with different number of parameters, applying the extra sum-of-square F test and the AIC test, it was possible to select the best model (without inhibition, competitive inhibition, noncompetitive inhibition, uncompetitive inhibition or mixed inhibition). In that sense, the model with the lowest sum of the square error value corresponds to the best kinetic model. The comparison between models with a different number of parameters can be done by comparing the extra sum-of-square F test, at the desired level of probability (ƒ 0.95): if the –ƒ value is superior to 0, the more complex model should be applied. The Akaike test was also used to discriminate the different enzyme inhibition models, with a different number of parameters: the model with the lower corrected AIC (AICc) score is the model more likely to be correct.
By the obtained results, it was possible to conclude that acarbose [see Supporting Information, Figures 1(S)–5(S)] is a mixed-type inhibitor, and flavonoids B4 [see Supporting Information, Figures 6(S)–10(S)], C5 [see Supporting Information, Figures 11(S)–15(S)] and D11 are competitive inhibitors [see Supporting Information, Figures 16(S)–20(S)].
After the definition of the best model, it was possible to summarize the kinetic constants values (Vmax, Km, Kic and/or Kiu) (). The tested compounds presented the following order of Kic values: B4>C5>D11>acarbose.
Table 2. Type of inhibition and Vmax, Km, Kic, and Kiuvalues ± SD (µM) for the inhibition of α-amylase by the selected flavonoids.
Molecular docking analysis
Binding mode of the selected inhibitors
Once evidenced the inhibition mechanism through experimental data and calculations using Solver supplement of Microsoft Excel Office™, it was predicted the binding pose of flavonoids within the active site of α-amylase. Special attention is given to B4, C5 and D11 derivatives, the ones exhibiting a higher inhibitory effect in their groups.
The binding pocket of α-amylase is usually divided in –1 to N subpockets, each one creating a particular environment around each sugar ring. The innermost subpocket is numbered as –1 subpocket and is immediately followed the +1 subpocket while the glycosidic bond to be cleaved stays positioned in-between the sugars and also in-between the –1 and +1 subpockets. The next subpockets are numbered +2, +3, etc. An even deeper subpocket (–2) can be occupied by inhibitors, but generally not by natural substrates.
We started by evaluating the accuracy of our Molecular Docking Protocol. For this purpose, we performed a docking assay using the crystal structure of alpha-amylase complexed with acarbose (Bacillus subtilis, PDB structure 1UA7, and resolution 2.21 Å). We deleted the acarbose hexasaccharide from the 1UA7 PDB file and re-docked it into the active site, following the protocol described in the methods, using the same parameters, except for the size of the box (which was, in this case, 21.75 × 22.50 × 22.50 Å). The top-ranking solution of the docking assay displayed a very similar pose to that of acarbose in the crystallographic structure [see Supporting Information, Figure 21(S)]. Root mean square deviation (RMSD) analysis of the heavy atoms in the docking solution concurs with visual inspection, with a very low heavy-atom RMSD value of 0.482 Å, confirming that the used protocol has significant predictive power.
The study started with the prediction of the binding mode of acarbose, which in the chosen receptor perfectly reproduces the binding interactions of the substrate maltohexose seen in the MD simulation (). This was expected, as it was a condition that we imposed to choose the receptor conformation for the docking calculations. The hydroxymethyl conduritol ring of acarbose accommodates to the acarviosine moiety bound to the –1 and +1 subpockets while the two farthest glucose units extend from the +2 subpocket to onwards. In such conformation, this non-hydrolyzable N-glycosidic bond stays moderately distant from the general acid Glu233 (4.2 Å), but the nucleophilic Asp197 is only at 3.3 Å from the anomeric carbon, Asp300 is hydrogen bonded to the 2-hydroxyl and 3-hydroxyl groups of the glucose unit of the –1 subpocket. An additional hydrogen bond is established between the hydroxymethyl group and Asp197.
Figure 3. Predicted binding mode for acarbose. Hydrogen bonds are represented by dashed lines and hydrophobic interactions by green lines. The distances between the catalytic residues Asp197 and Glu233 and the anomeric carbon/N-glycosidic bond are represented by dashed gray lines.

The most relevant interaction between the glucose unit in the +1 subpocket and the receptor seems to be the hydrophobic interaction between its methyl group and a hydrophobic pocket formed by Leu162 and Val163, and a hydrogen bond between the 3–OH and the backbone of Glu233. For the glucose unit at the +2 subpocket, the most relevant interaction seems to be a hydrogen bond between the 3–OH group and the side-chain of Tyr151. The glucose unit at the +3 subpocket is completely exposed to the solvent and does not interact meaningfully with the receptor.
Among flavonoids from A group, which carry substitutions at the 3-position of C-ring and at 3′- and 4′-positions of B-ring, a clear trend is observed: from flavonoids A1 to A5, the –OH groups at 3-position of C-ring and at 3′-position of B-ring establish hydrogen bonds with Asp300, while the –OH group at 4′-position of B-ring establishes hydrogen bonds with Asp197, with this hydrogen bonding network being optimized in flavonoid A5.
Therefore, flavonoids A1 to A5 bind the active site with the B-ring oriented towards the catalytic triad (Asp197, Asp300, Glu233), occupying a site equivalent to the –1 subpocket of the K2 inhibitor in the PDB structure 1HXOCitation35, while the A- and C-rings are pointing outwards occupying the binding site cleft equivalent to the –2 subpocket, which is a pocket not usually occupied by the natural substrate, located in the opposite direction in relation to the +1 subpocket.
For the less substituted A1 to A3 flavonoids, the described set of hydrogen bond interactions is not optimized and as such, they do not bind so deep in the active site. Instead, aromatic stacking interactions are observed between the A- and C-rings and Trp58, and between the B-ring and Tyr62.
When two –OH groups are added to B-ring (flavonoids A4 and A5) the hydrogen bonds with the catalytic triad are optimized and these flavonoids bind deeper in the active site, hydrogen bonding with Asp197, Glu233 and Asp300, which might explain the higher inhibitory activity observed for these two flavones within A group [].
Figure 4. (A) Predicted binding mode of flavonoid A5, occupying the –2 and –1 subsites with the more hydrophobic A-ring on the –2 subsite interacting with Leu162 and Val163. (B) The predicted binding mode for B4 shows the C-ring carbonyl and A-ring hydroxyl hydrogen bonded to the catalytic residues Glu233, Asp300 and His299.

The steric hindrance introduced by methoxylation at 3′- and 4′-positions of B-ring (flavonoid A6), flips the binding pose, placing instead the A- and C-rings in the –1 subpocket. This allows the –OH group at 3-position of C-ring to establish a hydrogen bond with Asp300 and Glu233.
As evidenced before, within B group of structurally related flavonoids, the best inhibitor is B4 with an IC50 value of 148 ± 5 µM. This compound shares with A4 and A5 the same hydroxyl substituents in the B-ring, but an additional –OH group at 5-position of the A-ring, which jointly promotes a stabilized conformation and an increase of the inhibition of α-amylase activity.
Looking at the pose predictions, it is clear that the C-ring carbonyl and the –OH group at the A-ring, promote the positioning of the A- and C-rings close to the catalytic triad. The orientation of the flavonoid, however, depends on the substitutions present in the B-ring [].
The two –OH substitutions present in the B-ring of flavonoid B4 increase the hydrophilicity of the catechol, which seems to distinguish B4 from the rest of the group. This allows the B-ring to occupy the +1 subpocket, with His201 hydrogen bonding the oxygen of the –OH group at 3′ position of B-ring, while that same –OH group makes a hydrogen bond with the oxygen of the Tyr151 hydroxyl []. This orientation is unlike B1 and B2, where the more hydrophobic B-ring binds the –2 subpockets, in the more hydrophobic region formed by Val163 and Leu165. Despite positioning the A- and C-ring between the catalytic triad, as the other flavonoids, this different orientation of the A- and C-ring of B4, seems to optimize the hydrogen-bonding network around the active site. Namely, the –OH group in the 5-position of A-ring establishes a hydrogen bond with Asp300 and to His299, while the C-ring carbonyl is hydrogen bonded to Glu233.
In turn, from C group, the 3–OH substitution present in C-ring of flavonoids C4 and C5 gives rise to a substructural motif of adjoined hydroxyl and carbonyl groups, similarly to the one found in the B group. On the latter, a C-ring carbonyl group adjoined by a –OH group at 4-position of A-ring allows the positioning of A- and C-rings within the catalytic triad. Therefore, the C-ring carbonyl and 3–OH substitution in C4 and C5 flavonoids also promotes the placing of the A- and C-rings within the catalytic triad, with the hydrogen of the –OH group at 3-position of C-ring bonded to Asp300 and to His299, and with the Glu233 hydrogen bonding the carbonyl group []. These positions of the carbonyl and –OH groups, exactly as in B4, and the same hydrogen-bonding network, are observed between these groups and the active site. This also means that C4 and C5 are bound in a flipped orientation when compared to flavonoid B4. Thus, these flavonoids occupy the subpockets +2 to –1, with the –OH group at 7-position of A-ring in the –1 subpocket, while the B-ring binds in the other side of the active site cleft, with the B-ring facing the more hydrophobic side of the substrate cleft characterized by Val163, Leu165 and Tyr62 (+2 subpocket). The B-ring establishes aromatic stacking interactions with Tyr62 and in the case of flavonoid C5, the 3′–OH substitution is hydrogen bonded to the backbone of Trp58. Moving this –OH substitution to 3′-position of B-ring, as in C4, leads to the breakage of the hydrogen bond with Trp58 and leaves the –OH at 3′-position of B-ring more exposed to the solvent, lowering the inhibitory activity of C4.
Figure 5. (A) The binding mode predicted for C5 shows the C-ring carbonyl and –OH group interacting with the active site residues in a similar way as in B4. This requires the B-ring to be placed in the –1/–2 subsites, stabilized mostly by hydrophobic interactions with Tyr62, Val163 and Leu165. (B)The best tested inhibitor, flavonoid D11, and flavonoid B4 binds very similarly to α-amylase. The most important difference seems to come from the 3–Cl substitution that interacts with Ile235 and Phe256.

The absence of an –OH group at 3-position of C-ring, as in C6, and the presence of an –OH substitution in the 3′ position of B-ring, define a completely different mode of interaction. In C6, the lack of hydrogen bond donors in the C-ring precludes the binding within the catalytic triad. Instead, B-ring binds the catalytic triad with the two –OH substitutions hydrogen bonding Asp300, while Glu233 hydrogen bonds the 3′–OH (+1 subpocket). In this binding mode, the A- and C-rings occupy the site of the substrate cleft corresponding to the –1 and –2 subpockets of the leaving maltose, with the hydrogen of the –OH at 7-position of A-ring bonding Glu240.
In what concerns D group, the binding mode of D11, the flavonoid with the higher inhibitory activity, is almost identical to B4. The C-ring carbonyl group adjoined by the –OH group of A-ring allow the binding of A- and C-rings within the catalytic triad, establishing the same hydrogen bonding network with the active site residues Asp300, His299 and Glu233 (subpocket +1). This also means that B-ring of flavonoid D11 binds in the same site as the B-ring of flavonoid B4 and, like B4, His201 hydrogen bonds the –OH group at 3′-position of B-ring, while the 3′–OH hydrogen bonds Tyr151 (subpocket –1). On the other hand, the –OH group at 7-position of A-ring seems to establish no specific interactions, while the –Cl ion at 3-position of C-ring fills the cavity formed by Ile235 and Phe256 and hydrogen bonds the backbone of Ile234 [].
A similar binding mode is predicted when the –Cl ion at 3-position of C-ring of flavonoid D11 is substituted by an -H or –OH group (D7 and D8, respectively), but the inhibitory activity is decreased by two-fold and three-fold, respectively. The lower shape complementarity of D7 and the resistance of binding the more electronegative oxygen in the pocket formed by Ile235 and Phe256 for flavonoid D8 might explain the decreased inhibitory activity.
It is interesting to compare D11 with D14, which bears an additional –Cl substitution at 8-position of A-ring. The binding mode of D14 is exactly the same as D11, thus the 8–Cl substitution binds in the hydrophobic pocket formed by Leu162, Val163 and Leu165. Despite the increased shape complementarity, the IC50 value increased by twofold. Perhaps a similar sized but more hydrophobic group in this position would be advantageous.
Methoxylation of the B-ring in D16, D17 and D18 flipped the mode of binding, when compared to D11, and disrupted the interactions with the active site triad.
Among flavonoids of the E group, it was possible to observe that exchanging the sp2 C2 carbon on the C-ring for a sp3 carbon, has a dramatic effect on the inhibitory efficiency when compared to D5, D7 and D8. The predicted mode of binding is the same for the three flavonoids, with the A- and C-rings occupying the +3 and +2 subpockets, while the B-ring occupies the +1 subpocket and hydrogen bonds Asp197. These three flavonoids clearly cannot occupy the subpockets +1 and –1 simultaneously to maximize the interaction with the catalytic triad, thus cannot inhibit α-amylase.
Discussion
The search for safer, specific and effective hypoglycaemic agents has been a concern of the scientific community. As a major player in the route leading to the assimilation of polysaccharides, the enzyme α-amylase plays an important role in controlling the postprandial blood glucose levels, thus being an interesting therapeutic target forT2DM. It was previously shown that the molecular models for the human and porcine pancreatic α-amylases are extremely similarCitation44, which enables the use of a porcine pancreatic α-amylase surrogate in microanalysis screening of novel α-amylase inhibitors [see Supporting Information, Figure 22(S), showing the superposition of the human and porcine enzymes].
In the present work, we tested the inhibition of pancreatic α-amylase activity by a panel of 40 flavonoids, that were divided among five groups (A–E) according to the respective parent structure scaffolds, with –OH, –OMe and/or –Cl substitutions, in order to elucidate a rationale for structure-activity relationship. The kinetic analysis, inhibitory mechanism determination and the corresponding molecular docking calculations were also applied, in order to explain the binding model of the most effective flavonoid of each group and the positive control, acarbose, regarding the three-dimensional structure of the α-amylase catalytic site. The obtained results clearly show that α-amylase inhibition by flavonoids varies with the nature, number and position of the substituents present in the derivative flavonoids’ structure. From the studied flavonoids, the most effective compound found was the chlorinated flavone D11.
In what concerns the A group, the tested flavonoids (A1–A6) present low or no ability to inhibit α-amylase up to the highest tested concentration, 200 µM. Comparing the flavone A1 with A2, A3, A4 and A5, it is clear that addition of –OH groups at 3-position of C-ring and at 3′- and/or at 4′-positions of B-ring lead to no effect against the α-amylase activity. In addition, the substitution of –OH groups by –OMe groups at 3′-and 4′-positions of B-ring (A6) also did not lead to any effect for the inhibitory activity of α-amylase.
The most active flavonoid of B group was B4 (IC50= 148 ± 5 µM). Thus, the presence of a catechol group in B-ring, together with the –OH group at 5-position of A-ring, seems distinctive features for inhibitory activity, especially if a comparison is made with those derivatives without –OH groups in B-ring (B1) or with just one –OH group at 3′- or 4′-positions of B-ring (B2 and B3, respectively). In addition, by comparing A5 with B4, it can be noticed that the –OH group seems to be more favourable at 5-position of A-ring than at 3-position of C-ring for the intended activity. This is in accordance to previous studies showing that the hydroxylation of A- (at 5- and 7-positions) and B-rings (at 3′- and 4′-positions) of flavonoids improve their inhibitory effect against this enzymeCitation4,Citation45–47.
The most effective flavonoid from the C group was C5 (IC50= 59 ± 4 µM), which was tested here for the first time. Taking into account the obtained result, it is possible to conclude that the presence of an –OH group at 3-position of C-ring, at 4′-position of B-ring and at 7-position of A-ring are advantageous for the inhibitory effect. Accordingly, it was previously reported, through the study of various structurally-related flavonoids, that the hydroxylation at 4′-position of B-ring and at 7-position of A-ring in flavonoids’ scaffold is relevant for their inhibitory activity against α-amylaseCitation4,Citation21,Citation45–48. The importance of the presence of an –OH group at 4′-position in B-ring is also highlighted by comparing C5 with C4 (–OH group at 3′-position of the B-ring), since flavonoid C4 presented low activity up to 200 µM, the highest tested concentration. The relevance of the presence of an –OH group at 3-position of C-ring together with an OH group at 4′-position of B-ring for the inhibition of α-amylase, is highlighted by the lack of inhibitory activity of C3, when compared with C5.
Concerning the studied methoxylated flavonoids, C7 and C8, both presented no inhibitory activity against α-amylase, corroborating the idea that the inhibitory activity of flavonoids against α-amylase is not favoured through methoxyl substitution. Accordingly, it was previously described in in vitro studies that the methylation and methoxylation in the structure of flavonoids reduce the inhibitory activity towards α-amylaseCitation45,Citation46,Citation48.
In D group we found the most active compound of this work, flavone D11, with a –Cl ion at 3-position of C-ring, a –OH group at 3′ and 4′-positions of B-ring and at 5- and 7-positions of A-ring, presenting an IC50 value = 44 ± 3 µM. Chlorinated flavonoids were previously reported by our research group as potent anti-inflammatory agents due to their ability to suppress mechanisms engaged at the onset and progression of inflammationCitation27,Citation49. Since both oxidative stress and inflammation play a key role in the pathogenesis of insulin resistance and dysfunction of β-cellsCitation50, we selected these promising chlorinated flavonoids and studied their effectiveness as α-amylase inhibitors. In addition, these flavonoids already proved to be effective compounds in the inhibition of α-glucosidaseCitation15 and PTP1BCitation16. Comparing flavones D11 and D8 (quercetin) (IC50 = 138 ± 5 µM), it is possible to conclude that the substitution of the –Cl ion by an –OH group, at 3-position of C-ring, significantly decreased the α-amylase inhibition. Moreover, the removal of the –Cl ion from 3-position of C-ring also led to a decrease of the inhibitory activity of flavonoids, as it is possible to verify by comparing D11 with D7 (luteolin) (IC50 = 78 ± 3 µM). Furthermore, the addition of an –OH group at 3-position of C-ring [D8 (quercetin)] significantly decreased the inhibitory activity towards α-amylase (138 ± 5 µM). The comparison of flavone D11 with D14 show that addition of a –Cl ion at 8-position of A-ring, led to a twofold increase of the IC50 value (82 ± 4 µM). Thus, the presence of a –Cl ion at 3-position of the C-ring is especially advantageous for the ability of flavonoids to inhibit α-amylase activity.
Through the comparison of the results obtained for flavone D9 (myricetin) (IC50 = 107 ± 6 µM) and D8 (quercetin) (IC50 = 138 ± 5 µM), it was possible to observe that the addition of an –OH group at 5′-position of B-ring slightly enhanced α-amylase inhibition. The results of the present work are in accordance with those reported by Meng et al.Citation25 and Tadera et al.Citation21, who also studied the α-amylase inhibition by quercetin and myricetin and found that myricetin was slightly more effective than quercetin.
By comparing D7 (luteolin) (IC50= 78 ± 3 µM) and D8 (quercetin) (IC50 = 138 ± 5 µM), it was also possible to conclude that the addition of an –OH group at 3-position of C-ring decreased the flavonoids’ inhibitory activity towards α-amylase. The obtained results are in agreement with the study of Tadera et al.Citation21, who also tested the mentioned flavonoids. In the study of Kim et al.Citation23, the authors also observed that luteolin was an effective α-amylase inhibitor.
Comparing methoxylated flavonoids with their parent hydroxylated flavonoids, it is possible to conclude that the presence of –OMe groups decrease α-amylase inhibition, as it can be seen comparing flavone D16 (chrysoeriol) and D18 with D7 (luteolin), and D17 (acacetin) with D5 (apigenin). These results are in agreement with previous studies found in literatureCitation45–48,Citation51.
A glycosylated flavonoid was also tested, D19 (rutin), and the presence of a sugar in the flavonoid structure did not favour its ability to inhibit α-amylase activity. In this sense, the obtained results showed that the substitution of an –OH group [D8 (quercetin)] by a rutinose [D19 (rutin)] at 3-position of C-ring reduced the α-amylase inhibition by flavonoids. Accordingly, it was previously described in the literature that the glycosylation of flavonoids decreases their inhibitory effect against α-amylase, possibly due to the increasing molecular size and polarity, and to the nonplanar structureCitation45,Citation46,Citation48. In addition, this conclusion is in agreement with the study of Li et al.Citation24, who tested the interaction between quercetin, rutin and isoquercetin with α-amylase, and found that the binding affinity of quercetin to the enzyme is higher when compared to rutin.
The flavanones from E group did not inhibit α-amylase, up to the highest tested concentration (200 µM). Comparing E1 (naringenin) with D5 (apigenin), E2 (eriodictyol) with D7 (luteolin) and E3 (taxifolin) with D8 (quercetin), it was observed that the lack of the C2 = C3 double bond did not favour the intended effect. This conclusion is in agreement with Tadera et al.,Citation21 who observed that naringenin was not able to inhibit porcine pancreatic α-amylase activity, at the highest tested concentration of 0.5 mM. It is well known that the C2 = C3 double bond allows an easier entry of the molecules into the hydrophobic pockets of α-amylase since the presence of this double bond favours the near-planarity of flavonoidsCitation4,Citation45,Citation46,Citation48.
Acarbose is a pseudotetrasaccharide produced by Actinoplanes sp. fermentation and its structure comprises a hydroxymethyl conduritol residue α-(1,4) linked to a 4-amino-4,6-dideoxyglucose which is, in turn, α-(1,4) linked to maltoseCitation52,Citation53. It is the most widely prescribed α-glucosidase and α-amylase inhibitor. However, it was previously shown that only a mild inhibition of pancreatic α-amylase is recommended in order to avoid gastrointestinal side-effects as a result of excessive bacterial fermentation of carbohydrates in colonCitation11, that has been shown as a limiting factor for T2DM treatment, in some countriesCitation11. In the present work, the IC50 values found for the tested flavonoids were higher than the IC50 value found for acarbose, which is 1.3 ± 0.2 µM. The mild pancreatic α-amylase inhibition prevents the abnormal bacterial fermentation of carbohydrates in the colon and consequent gastrointestinal adverse effects, such as abdominal distention, flatulence and diarrheaCitation11. Moreover, it was previously shown by our research group that, in general, the tested flavonoids were more effective concerning the inhibition of α-glucosidase than the inhibition of α-amylaseCitation15. As such, the obtained results suggest that flavonoids could be promising α-amylase inhibitors and cause less gastrointestinal side-effects. Interestingly, it was found that the combination of baicalein or apigenin with acarbose showed additive inhibition of α-amylase at lower concentrations and antagonistic inhibition at higher concentrations. On the other hand, the combination of baicalein with acarbose synergistically inhibits α-glucosidase and lowers HGPPCitation54.
After the study of α-amylase inhibition by flavonoids, we selected the most active flavonoid of each group (B4, C5 and D11) and the positive control, acarbose, to test their type of inhibition.
It was described that the discrimination between mechanisms of enzymatic inhibition based on linear transformation of Michaelis–Menten equation is less accurate than the use of the nonlinear regression, since the transformation can also mask the variability inherent to kinetic dataCitation29,Citation30. By the same reason, modelling on the means of repeated experiments instead of using individual data can also lead to misinterpreted mechanisms. In that sense, for the enzyme kinetic analysis, it was used the nonlinear regression to determine kinetic parameters and the type of inhibition of the most active flavonoids of each group. For this purpose, it was applied the Solver supplement of Microsoft Office Excel™. By means of iterative guesses for variables in each model equation, Solver was asked to minimize the sum of squared residuals between individual raw experimental results and the corresponding values generated by the model.
In accordance, kinetic analysis consisted in the sequential fitting of available models (without inhibition, competitive inhibition, noncompetitive inhibition, uncompetitive inhibition and mixed inhibition), and in the estimation of the kinetic constants (parameters) values. It was possible to observe by the analysis of the sum of the square errors values from the different models, and by the application of F-test and AIC test, that acarbose presents a mixed-type inhibition [see Supporting Information, Figures 1(S)–5(S)], and flavonoids B4 [see Supporting Information, Figures 6(S)–10(S)], C5 (see Supporting Information, Figures 11(S)–15(S)) and D11 [see Supporting Information, Figures 16(S)–20(S)] present a competitive inhibition. Accordingly, Kim et al.Citation55 and Yoon and Robyt,Citation52 also described acarbose as a mixed type inhibitor of α-amylase. To the best of our knowledge, the inhibition type of flavonoids B4, C5 and D11 were studied here for the first time.
Once defined the best kinetic model for acarbose and for flavonoids B4, C5 and D11, the kinetic constants were estimated: Vmax, Km, Kic and Kiu. The calculation of the Ki value is considered a useful tool to compare the potency of inhibitors. The Kic values of the selected compounds demonstrated the following order: B4>C5>D11>acarbose. This means that, as expected, acarbose has the highest binding affinity to α-amylase, followed by flavonoids D11, C5 and B4.
The prediction of the binding mode of the most active flavonoids towards α-amylase in each group showed that one of the most important aspects shared by them is a motif bearing a carbonyl group next to a –OH group. This allows the carbonyl group to accept a hydrogen bond from Glu233 and the –OH group to donate a hydrogen bond to Asp300 in the region between the –1 subpocket and +1 subpocket. The interactions with these groups can be further enhanced with a hydrogen bond between His299 and the -OH group.
The relative positioning of the -OH and carbonyl groups determine, to a great extent, the orientation of the flavonoid. Thus, the flavonoid part on the side of the carbonyl will be preferably oriented towards the +1 subpocket, while the part on the -OH side will be preferably oriented towards the –1 subpocket.
Hydrophobic groups seem to be more tolerated on the –1/–2 subpockets than on the +1/+2 subpockets, where they can interact with Tyr62, Leu162, Val163 and Leu165. Hydrophobic groups on the +1/+2 subpockets, on the other hand, can establish hydrogen bonds with Tyr151 and His201.
Halogen substitutions or hydrophobic groups might be used next to the carbonyl to explore the interaction with Ile235 and Phe256, as the best flavonoid tested herein shows.
Conclusion
In the present study, the inhibition of pancreatic α-amylase by a panel of 40 flavonoids (A–E groups) was tested, in order to establish an accurate structure-activity relationship. For that purpose, the kinetic analysis, the inhibitory mechanism determination and the corresponding molecular docking calculations were applied, to explain the binding model of the most effective flavonoid of each group to the three-dimensional structure of α-amylase catalytic site. Thus, from the obtained results, it may be concluded that α-amylase inhibition by flavonoids is strongly dependent on the nature and position of the substituents. The most effective flavonoid was the chlorinated flavone D11. As such, the presence of an –OH group at 5- and 7-positions of A-ring and in the 3′- and 4′-positions of B-ring, and the presence of a –Cl ion at 3-position of C-ring, as well as the C2 = C3 double bond, have shown to be favourable for the intended effect (). This flavonoid presented a competitive type of inhibition. The promising α-amylase inhibitory activity of some of the studied flavonoids should be deeply explored allowing these compounds to be considered as possible alternatives for the management of PPHG and consequently T2DM.
Supplemental Material
Download PDF (1,002.5 KB)Disclosure statement
No potential conflict of interest was reported by the authors.
Additional information
Funding
References
- Butterworth PJ, Warren FJ, Ellis PR. Human α-amylase and starch digestion: an interesting marriage. Starch–Stärke 2011;63:395–405.
- Patel H, Royall PG, Gaisford S, et al. Structural and enzyme kinetic studies of retrograded starch: inhibition of α-amylase and consequences for intestinal digestion of starch. Carbohydr Polym 2017;164:154–61.
- Brayer GD, Sidhu G, Maurus R, et al. Subsite mapping of the human pancreatic α-amylase active site through structural, kinetic, and mutagenesis techniques. Biochemistry 2000;39:4778–91.
- Lo Piparo E, Scheib H, Frei N, et al. Flavonoids for controlling starch digestion: structural requirements for inhibiting human α-amylase. J Med Chem 2008;51:3555–61.
- Sales PM, Souza PM, Simeoni LA, et al. Α-amylase inhibitors: a review of raw material and isolated compounds from plant source. J Pharm Pharm Sci 2012;15:141–83.
- Liu D, Gao H, Tang W, Nie S. Plant non-starch polysaccharides that inhibit key enzymes linked to type 2 diabetes mellitus. Ann N Y Acad Sci 2017;1401:28–36.
- Martinez-Gonzalez AI, Diaz-Sanchez AG, Rosa LA, et al. Polyphenolic compounds and digestive enzymes: in vitro non-covalent interactions. Molecules 2017;22:1–24.
- Janecek S, Svensson B, MacGregor EA. α-Amylase: an enzyme specificity found in various families of glycoside hydrolases. Cell Mol Life Sci 2014;71:1149–70.
- International Diabetes Federation. IDF Diabetes Atlas. In IDF Diabetes Atlas. 8th ed. 2017. Available from: http://diabetesatlas.org/IDF_Diabetes_Atlas_8e_interactive_EN/
- American Diabetes Association. Classification and diagnosis of diabetes: standards of medical care in diabetes–2018. Diabetes Care 2018;41:S13–S27.
- Etxeberria U, de la Garza AL, Campion J, et al. Antidiabetic effects of natural plant extracts via inhibition of carbohydrate hydrolysis enzymes with emphasis on pancreatic alpha amylase. Expert Opin Ther Targets 2012;16:269–97.
- Kahn SE, Cooper ME, Del Prato S. Pathophysiology and treatment of type 2 diabetes: perspectives on the past, present, and future. Lancet 2014;383:1068–83.
- Wedick NM, Pan A, Cassidy A, et al. Dietary flavonoid intakes and risk of type 2 diabetes in US men and women. Am J Clin Nutr 2012;95:925–33.
- Liao X, Wang X, Li H, et al. Sodium-glucose cotransporter 2 (SGLT2) inhibitor increases circulating zinc-α2-glycoprotein levels in patients with type 2 diabetes. Sci Rep 2016;6:1–12.
- Proenca C, Freitas M, Ribeiro D, et al. α-Glucosidase inhibition by flavonoids: an in vitro and in silico structure–activity relationship study. J Enzyme Inhib Med Chem 2017;32:1216–28.
- Proenca C, Freitas M, Ribeiro D, et al. Inhibition of protein tyrosine phosphatase 1B by flavonoids: a structure–activity relationship study. Food Chem Toxicol 2018;111:474–81.
- Nicolle E, Souard F, Faure P, Boumendjel A. Flavonoids as promising lead compounds in type 2 diabetes mellitus: molecules of interest and structure–activity relationship. Curr Med Chem 2011;18:2661–72.
- Vinayagam R, Xu B. Antidiabetic properties of dietary flavonoids: a cellular mechanism review. Nutr Metab 2015;12:1–20.
- Xiao JB, Hogger P. Dietary polyphenols and type 2 diabetes: current insights and future perspectives. Curr Med Chem 2015;22:23–38.
- Kawser HM, Abdal DA, Han J, et al. Molecular mechanisms of the anti-obesity and anti-diabetic properties of flavonoids. Int J Mol Sci 2016;17:569–32.
- Tadera K, Minami Y, Takamatsu K, Matsuoka T. Inhibition of alpha-glucosidase and alpha-amylase by flavonoids. J Nutr Sci Vitaminol 2006;52:149–53.
- Gu C, Zhang H, Putri CY, Ng K. Evaluation of α-amylase and α-glucosidase inhibitory activity of flavonoids. Int J Food Nutr Sci 2015;2:1–6.
- Kim JS, Kwon CS, Son KH. Inhibition of alpha-glucosidase and amylase by luteolin, a flavonoid. Biosci Biotechnol Biochem 2000;64:2458–61.
- Li Y, Gao F, Gao F, et al. Study on the interaction between 3 flavonoid compounds and α-amylase by fluorescence spectroscopy and enzymatic kinetics. J Food Sci 2009;74:C199–203.
- Meng Y, Su A, Yuan S, et al. Evaluation of total flavonoids, myricetin, and quercetin from Hovenia dulcis thunb. as inhibitors of α-amylase and α-glucosidase. Plant Foods Hum Nutr 2016;71:444–9.
- Ribeiro D, Freitas M, Tome SM, et al. Modulation of human neutrophils' oxidative burst by flavonoids. Eur J Med Chem 2013;67:280–92.
- Freitas M, Ribeiro D, Tome SM, et al. Synthesis of chlorinated flavonoids with anti-inflammatory and pro-apoptotic activities in human neutrophils. Eur J Med Chem 2014;86:153–64.
- Trinh BTD, Staerk D, Jäger AK. Screening for potential α-glucosidase and α-amylase inhibitory constituents from selected Vietnamese plants used to treat type 2 diabetes. J Ethnopharmacol 2016;186:189–95.
- Bezerra RMF, Fraga I, Dias AA. Utilization of integrated Michaelis–Menten equations for enzyme inhibition diagnosis and determination of kinetic constants using Solver supplement of Microsoft Office Excel. Comput Methods Programs Biomed 2013;109:26–31.
- Dias AA, Pinto PA, Fraga I, Bezerra RMF. Diagnosis of enzyme inhibition using Excel Solver: a combined dry and wet laboratory exercise. J Chem Educ 2014;91:1017–21.
- Motulsky H, Christopoulos A, Fitting models to biological data using linear and nonlinear regression: a pratical guide to curve fitting. New York: Oxford University Press; 2004.
- Burguillo FJ, Wright AJ, Bardsley WG. Use of the F test for determining the degree of enzyme-kinetic and ligand-binding data. A Monte Carlo simulation study. Biochem J 1983;211:23–34.
- Yamaoka K, Nakagawa T, Uno T. Application of Akaike's information criterion (AIC) in the evaluation of linear pharmacokinetic equations. J Pharmacokinet Biopharm 1978;6:165–75.
- Naes T, Isakson T, Fearn T, Davies T, A user-friendly guide to multivariate calibration and classification. Chichester: NIR Publications; 2002.
- Qian M, Nahoum V, Bonicel J, et al. Enzyme-catalyzed condensation reaction in a mammalian alpha-amylase. High-resolution structural analysis of an enzyme-inhibitor complex. Biochemistry 2001;40:7700–9.
- Bietz S, Urbaczek S, Schulz B, Rarey M. Protoss: a holistic approach to predict tautomers and protonation states in protein-ligand complexes. J Cheminform 2014;6:1–12.
- Case DA, Darden TA, Cheatham TE, et al. Amber 12. In. Amber 12. San Francisco: University of California; 2012.
- Hornak V, Abel R, Okur A, et al. Comparison of multiple amber force fields and development of improved protein backbone parameters. Proteins 2006;65:712–25.
- Kirschner KN, Yongye AB, Tschampel SM, et al. Glycam06: a generalizable biomolecular force field. J Comput Chem 2008;29:622–55.
- Trott O, Olson AJ. Autodock vina: improving the speed and accuracy of docking with a new scoring function, efficient optimization, and multithreading. J Comput Chem 2010;31:455–61.
- O'Boyle NM, Morley C, Hutchison GR. Pybel: a python wrapper for the openbabel cheminformatics toolkit. Chem Cent J 2008;2:5–7.
- Gasteiger J, Marsili M. Iterative partial equalization of orbital electronegativity–a rapid access to atomic charges. Tetrahedron 1980;36:3219–28.
- Jones G, Willett P, Glen RC, et al. Development and validation of a genetic algorithm for flexible docking. J Mol Biol 1997;267:727–48.
- Qian M, Spinelli S, Driguez H, Payan F. Structure of a pancreatic α-amylase bound to a substrate analogue at 2.03 a resolution. Protein Sci 2008;6:2285–96.
- Cao H, Chen X. Structures required of flavonoids for inhibiting digestive enzymes. Anticancer Agents Med Chem 2012;12:929–39.
- Xiao J, Ni X, Kai G, Chen X. A review on structure–activity relationship of dietary polyphenols inhibiting α-amylase. Crit Rev Food Sci Nutr 2013;53:497–506.
- Gonzales GB, Smagghe G, Grootaert C, et al. Flavonoid interactions during digestion, absorption, distribution and metabolism: a sequential structure–activity/property relationship-based approach in the study of bioavailability and bioactivity. Drug Metab Rev 2015;47:175–90.
- Xu W, Shao R, Xiao J. Is there consistency between the binding affinity and inhibitory potential of natural polyphenols as α-amylase inhibitors? Crit Rev Food Sci Nutr 2016;56:1630–9.
- Proença C, Ribeiro D, Soares T, et al. Chlorinated flavonoids modulate the inflammatory process in human blood. Inflammation 2017;40:1155–65.
- Pitocco D, Tesauro M, Alessandro R, et al. Oxidative stress in diabetes: implications for vascular and other complications. Int J Mol Sci 2013;14:21525–50.
- Asghari B, Salehi P, Sonboli A, Nejad Ebrahimi S. Flavonoids from salvia chloroleuca with α-amylase and α-glucosidase inhibitory effect. Iran J Pharm Res 2015;14:609–15.
- Yoon S-H, Robyt JF. Study of the inhibition of four alpha amylases by acarbose and its 4iv-α-maltohexaosyl and 4iv-α-maltododecaosyl analogues. Carbohydr Res 2003;338:1969–80.
- Rosak C, Mertes G. Critical evaluation of the role of acarbose in the treatment of diabetes: patient considerations. Diabetes Metab Syndr Obes 2012;5:357–67.
- Zhang BW, Li X, Sun WL, et al. Dietary flavonoids and acarbose synergistically inhibit α-glucosidase and lower postprandial blood glucose. J Agric Food Chem 2017;65:8319–30.
- Kim M, Lee S, Lee H, et al. Comparative study of the inhibition of α-glucosidase, α-amylase, and cyclomaltodextrin glucanosyltransferase by acarbose, isoacarbose, and acarviosine–glucose. Arch Biochem Biophys 1999;371:277–83.