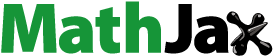
Abstract
Neisseria gonorrhoeae is a high-priority pathogen of concern due to the growing prevalence of resistance development against approved antibiotics. Herein, we report the anti-gonococcal activity of ethoxzolamide, the FDA-approved human carbonic anhydrase inhibitor. Ethoxzolamide displayed an MIC50, against a panel of N. gonorrhoeae isolates, of 0.125 µg/mL, 16-fold more potent than acetazolamide, although both molecules exhibited almost similar potency against the gonococcal carbonic anhydrase enzyme (NgCA) in vitro. Acetazolamide displayed an inhibition constant (Ki) versus NgCA of 74 nM, while Ethoxzolamide’s Ki was estimated to 94 nM. Therefore, the increased anti-gonococcal potency of ethoxzolamide was attributed to its increased permeability in N. gonorrhoeae as compared to that of acetazolamide. Both drugs demonstrated bacteriostatic activity against N. gonorrhoeae, exhibited post-antibiotic effects up to 10 hours, and resistance was not observed against both. Taken together, these results indicate that acetazolamide and ethoxzolamide warrant further investigation for translation into effective anti-N. gonorrhoeae agents.
1. Introduction
Neisseria gonorrhoeae, a Gram-negative pathogen, and the causative agent of gonorrhoea, is an emerging super-pathogen that has seen a rapid rise in new cases worldwide over the past decade. The World Health Organisation (WHO) reported a surge of new cases of gonorrhoea from 78 millionCitation1 in 2012 to as many of 87 million new infections worldwide in 2016Citation2. Moreover, the Centres for Disease Control and Prevention (CDC) reported a record of 583,000 new cases of drug-resistant gonorrhoea in the United States in 2018Citation3.
The growing number of infections is only part of the problem. Equally concerning is the pathogen has demonstrated a keen ability to develop resistance to several classes of FDA-approved antibiotics. Global surveillance programs have identified alarming resistance rates to the available antibiotics such a β-lactams, tetracyclines, and quinolinesCitation4. As recently as 2017, 6–30% of N. gonorrhoeae isolates in the United States were resistant to ciprofloxacin and that number increased to 71–100% of isolates in other regions of the worldCitation5. In addition, resistance to the macrolide drug, azithromycin, has recently grown to greater than 33% in some regions of the worldCitation5–7. This emerging resistance was cited as one of the reasons the CDC removed azithromycin from the standard treatment guidelines for uncomplicated gonorrhoea, in 2020, leaving only ceftriaxone injection as the drug of choiceCitation8. However, although rare for the time being, isolated cases of treatment failure with ceftriaxone have been reportedCitation9–11. Due to the combination of increased rates of infection and prevalence of drug-resistant strains worldwide, both the WHO and CDC have classified drug-resistant N. gonorrhoeae within the highest threat level to public healthCitation5,Citation12 and highlighted an urgent need to identify new molecular targets and chemical scaffolds to combat this pathogen. Otherwise, the world faces the real possibility of an untreatable gonococcal infectionCitation13.
One method that has the potential to "fast-track" discovery of new antibiotics is through a drug repurposing strategyCitation14 because it accelerates the process of drug discovery and reduces the time to marketCitation15,Citation16. To this end, there have been reports of FDA-approved carbonic anhydrase inhibitors (CAIs) with potency against several pathogens such as Helicobacter pyloriCitation17–19, Neisseria spp. Citation20,Citation21, and Mycobacterium tuberculosisCitation22. Other groups have reported the potential of human CAIs, or analogs thereof, against recombinant bacterial carbonic anhydrasesCitation23–25 including Helicobacter pyloriCitation26,Citation27, Vibrio choleraeCitation28, Burkholderia sppCitation29,Citation30, and Streptococcus pneumoniaeCitation31 to name a few. However, while many of these studies demonstrate activity against the bacterial carbonic anhydrases in vitro, they stop short of demonstrating antimicrobial efficacy against the pathogens. Also of note, while humans encode for only the α-carbonic anhydrase subfamily, bacteria have been found to encode for α-, β-, γ-, ι-subfamily carbonic anhydrasesCitation29,Citation32,Citation33. However, there is limited information about inhibition or ligand-bound structural data for inhibitors against non-α-class carbonic anhydrases.
As part of a drug repurposing effort, our team has reported that the human CAI, acetazolamide (AZM), and the newly developed CAI scaffold displayed improved efficacy against vancomycin-resistant Enterococcus (VRE)Citation34–36 and N. gonorrhoeaeCitation37 and are likely inhibiting essential bacterial α-carbonic anhydrases in each species. As an extension of that work, we report herein further studies comparing the human CAIs, acetazolamide (AZM) and ethoxzolamide (EZM) () for in vitro efficacy against N. gonorrhoeae.
Figure 1. Structures of FDA-approved human carbonic anhydrase inhibitors acetazolamide and ethoxzolamide.

Both AZM and EZM are approved for use as diuretics and used for treating glaucomaCitation38–41. AZM is also prescribed as a chronic treatment for epilepsyCitation42,Citation43, symptoms associated with congestive heart failureCitation44, and altitude sicknessCitation45,Citation46. Additionally, AZM is investigated in various clinical trials (clinicaltrials.gov) that are active or in the recruitment phases, for the treatment of conditions such as ataxia (NCT04679389), sleep apnoea (NCT04726982), chronic obstructive pulmonary disorder (NCT04915365), and cancer (NCT03011671). Moreover, AZM is included in the WHO’s list of essential medicines due to its low cost, safe pharmacokinetic and toxicity profiles and overall efficacyCitation47. Further, AZM is generally safe as a phase 1 clinical trial showed 89.5% of patients were able to tolerate >1 g/day dose for 6 months with 45% of patients tolerating up to 4 g/day over the same time periodCitation48,Citation49. The drug is often dosed chronically to patients at >1 g/dayCitation49–51, is orally bioavailable with a plasma elimination half-life of 4–6 h in adults after 250 mg oral doseCitation45, and 100% of the drug is excreted in the urine with no metabolitesCitation52–54. It has also been shown that due to the expression of human carbonic anhydrase isoforms I and II in erythrocytes, AZM partitions readily into the red blood cells portion of bloodCitation55,Citation56. This effectively forms a sink that sequesters the drug and doubles the elimination half-life (∼12 h) when the whole blood concentration is quantifiedCitation45.
Concerning EZM, there is considerably less publicly available information regarding safety, tolerability, and pharmacokinetics. If dosed orally, it is commonly prescribed at 125 mg every 6 h and has a plasma half-life of 6 h in humansCitation57. Unlike AZM, only 40% of EZM appears unchanged in the urine with the rest of the molecule being O-dealkylated followed by subsequent glucuronidation at the free phenolCitation39.
While previous literature has demonstrated that AZM and EZM have activity against N. gonorrhoeaeCitation20,Citation21, it has stopped short of further elucidating the antimicrobial properties for these molecules against the pathogen. In the case of EZM, while Sanders and Maren reported anti-Neisseria activityCitation20, they only provided ranges of MICs and not a full study on the drug's antimicrobial properties. Our recent work further investigated AZM alongside new CAI analogsCitation37, but there is still more to be learned from how AZM and EZM affect N. gonorrhoeae beyond standard MIC measurements. To this end, we present additional data for the antimicrobial properties of AZM and EZM including in vitro activity versus the proposed intracellular target, MICs versus a panel of clinical N. gonorrhoeae strains, antibiotic efficacy with regards to killing kinetics, post-antibiotic effects, the frequency of resistance development and bacterial accumulation in N. gonorrhoeae are all described.
2. Materials and methods
2.1. Bacterial strains, media and chemicals
N. gonorrhoeae strains (Table S1) used in the study were clinical isolates obtained from the CDC and the American Type Culture Collection (ATCC) (Manassas, VA, USA). Drugs used in this study were purchased from chemical vendors: AZM (Alfa Aesar, Tewksbury, MA, USA), EZM and tetracycline (Sigma-Aldrich, Saint Louis, MO, USA), azithromycin and ceftriaxone (TCI America, Portland, OR, USA). Media and reagents were purchased commercially: brucella broth, GC medium base, IsoVitaleX, bovine haemoglobin, and chocolate II agar plates (Becton, Dickinson and Company, Cockeysville, MD, USA), yeast extract and dextrose (Fisher Bioreagents, Fairlawn, NJ, USA), protease peptone (Oxoid, Lenexa, KS, USA), haematin, pyridoxal, and nicotinamide adenine dinucleotide (NAD) (Chem-Impex International, Wood Dale, IL, USA), corn starch (Spectrum Chemical MFG, Gardena, CA, USA), monobasic potassium phosphate and dibasic potassium phosphate (Macron chemicals, Centre Valley, PA, USA), and sodium chloride (Fisher Scientific, Fail Lawn, NJ, USA), and phosphate-buffered saline (PBS) (Corning, Manassas, VA, USA).
Table 1. MICs for AZM and EZM against a panel of N. gonorrhoeae clinical isolates.
2.2. Antibacterial activity of AZM and EZM against N. gonorrhoeae strains
The minimum inhibitory concentrations (MICs) of compounds were carried out as described previousyCitation37,Citation58–60. Briefly, N. gonorrhoeae strains were grown overnight on chocolate II agar plates. Bacterial cells were then suspended in PBS to achieve turbidity equivalent to a 1.0 McFarland standard which was diluted in Brucella broth supplemented with yeast extract, dextrose, protease-peptone, NAD, pyridoxal, haematin and IsoVitaleX to reach a bacterial count of about 1 × 106 CFU/mL. Drugs were added and serially diluted along with the plates. Media containing bacteria (without test agents) were included in the assays as a control. Plates were then incubated in the ambient air, and in presence of 5% CO2 for 24 h at 37 °C before recording the MICs as observed visually. MICs reported are the minimum concentrations of drugs that completely inhibited the visual growth of bacteria.
2.3. Carbonic anhydrase CO2 hydration catalytic assay and Ki determination
The assay was performed according to previously published protocolsCitation37,Citation61–65. Recombinant N. gonorrhoeae carbonic anhydrase (NgCA) was produced as previously describedCitation37. Recombinant human carbonic anhydrase (hCA) I and hCA II were purchased from Millipore Sigma (hCA I Catalog# C4396-5MG; hCA II Catalog # C6624-500UG). Ki values were determined from inputting the IC50 values into the Cheng-Prusoff equationCitation66 for Ki from catalytic inhibition constants.
2.4. Killing kinetics assay
To determine the mode of the killing of AZM and EZM, a standard time-kill kinetics assay was performed against N. gonorrhoeae ATCC 700825 as described previouslyCitation67–69. N. gonorrhoeae was grown in GC broth supplemented with IsoVitaleX to logarithmic phase and further diluted to reach an initial inoculum of ∼106 CFU/mL. AZM, EZM, azithromycin, and ceftriaxone were then added (at 10× MIC in triplicates), and further incubated in the ambient air at 37 °C for 24 h. Bacteria exposed to DMSO (solvent of drugs) served as a negative control. An aliquot from each treatment was collected after the corresponding times of incubation and subsequently serially diluted and plated onto chocolate II agar plates. Plates were incubated for 24 h at 37 °C before viable CFU/mL was determined.
2.5. Accumulation of AZM and EZM inside N. gonorrhoeae cells
2.5.1. Sample preparation
The accumulation assay was performed as described previouslyCitation70,Citation71. An overnight culture of N. gonorrhoeae ATCC 700825 was diluted 1:100 in GC broth supplemented with IsoVitaleX, and grown at 37 °C with shaking for 12 h. The bacteria were pelleted, washed once in PBS and then resuspended in fresh PBS, aliquoted into sixteen 1.7 ml Eppendorf tubes (4 tubes for each test agent), diluted, and counted. AZM, EZM, and tetracycline (positive control) were added at a concentration of 50 μM. DMSO was included as a negative control. Samples were then incubated at 37 °C with shaking for either 10 min., 30 min., 1 h, or 2 h. After incubation, 800 μL of the cultures were carefully layered on 700 μL of silicone oil mixture (AR20/High Temperature (9:1)). Bacteria were pelleted through the oil by centrifuging at 13,000 r.c.f. for 2 min. at room temperature. The supernatant and oil were then removed by pipetting. Afterwards, samples were lysed by resuspending the pellet in 200 μL of sterile distilled water, and then they were subjected to four freeze-thaw cycles (three minutes in liquid nitrogen followed by three minutes in a water bath at 65 °C). The lysates were pelleted and the supernatant was collected. The debris was re-suspended in 100 μL of methanol and pelleted as before. The supernatants were removed and combined with the previous supernatants collected. The remaining debris was removed by centrifuging. Samples (50 μL) from each replicate were diluted 10-fold in a solution of 25% water and 75% methanol. Sample solutions were sonicated for 5 min, centrifuged at 16,000 g for 8 min, then the supernatants were transferred to HPLC vials.
2.5.2. HPLC/MS-MS analysis
Samples were quantitated for AZM, EZM, and tetracycline by HPLC/MS-MS. Separations were performed on an Agilent Rapid Res 1200 HPLC system using an Agilent Zorbax XDB-C18 (2.1 × 50 mm, 3.5 μm) column. Mobile phase A was water with 0.1% formic acid and mobile phase B was acetonitrile with 0.1% formic acid. Initial conditions were 90:10 A:B, followed by a linear gradient to 10:95 at 10 min. Column re-equilibration was performed by returning to 90:10 A:B at 11 min and held until 16 min. The column flow rate was 0.3 ml/min. Retention times for AZM, EZM, and tetracycline were 1.5, 7.0, and 4.0 min, respectively.
Analytes were quantified by MS/MS utilising an Agilent 6460 triple quadrupole mass spectrometer with positive electrospray ionisation (ESI). Quantitation was based on Multiple Reaction Monitoring (MRM). AZM was detected with a transition of 223.0–181.0 (quantifier) and 163.9 (qualifier), with collision energies (CE) of 10 V and 20 V, respectively. EZM was detected with a transition of 258.9–178.0 (quantifier) and 150.0 (qualifier), with CE of 15 V and 20 V, respectively. Tetracycline was detected with a transition of 445.1–410.1 (quantifier) and 154.0 (qualifier), with CE of 20 V and 25 V, respectively. Fragment energy of 145 V and a dwell time of 200 ms were used. All data were collected and analysed with Agilent MassHunter B0.03 software. Quantitation was based on 5-point standard curves, with concentration ranges from 3 to 1,700 ng/mL. Correlation coefficients of >0.9999 were obtained.
2.6. Post-antibiotic effect of AZM and EZM
The post-antibiotic effect (PAE) of AZM and EZM was tested against two N. gonorrhoeae strains (CDC 181, and CDC 186) following the procedure previously describedCitation72–74. Briefly, N. gonorrhoeae strains were grown in brucella supplemented broth to logarithmic phase and further diluted to reach an initial inoculum of about 1 × 106 CFU/mL. AZM, EZM, or azithromycin (10 × MIC, in triplicates) were added to bacteria and incubated at 37 °C with agitation under ambient air conditions for one hour. DMSO was included as growth control. Thereafter, drugs were removed by diluting each tube 1:1000 in fresh brucella supplemented broth, and tubes were incubated as previously described for 12 h. Samples were collected from each tube every two hours, serially diluted, and plated on GC II agar plates. The PAE was calculated using this equation: PAE = T − C, where T is the time taken by the bacterial culture treated with the drug to increase by one log10, while C is the time required for the negative control (DMSO) to increase by one log10.
2.7. Frequency of spontaneous mutation
N. gonorrhoeae CDC 202 was tested against AZM, EZM, or rifampin for a single-step mutation assay as previously describedCitation75–77. Briefly, drugs were mixed with GC agar supplemented with IsoVitaleX at the concentrations of 5× and 10× MIC. Plates were then prepared and allowed to dry at room temperature. A bacterial suspension (∼109 CFU/mL) was prepared and spread over the plates containing the drugs tested. Plates were incubated at 37 °C under ambient air conditions for 48 h before determining the bacterial CFU.
3. Results
3.1. Anti-gonococcal activity of AZM and EZM
As depicted in , both AZM and EZM were tested for antimicrobial activity against a panel of 22 N. gonorrhoeae clinical isolates including WHO reference isolates. In general, EZM outperformed AZM across the panel tested with MIC values ranging from 0.06 to 0.5 µg/mL. EZM inhibited 50% of the isolates tested (MIC50) at the concentration of 0.125 µg/mL and 90% of the tested isolates (MIC90) at 0.25 µg/mL. This equated to 16-fold better potency as compared to AZM, which exhibited MIC50 and MIC90 values of 2 µg/mL and 4 µg/mL, respectively. Azithromycin (AZI) inhibited strains tested at concentrations ranging from 0.125 µg/mL to 4 µg/mL (with exception of two strains that exhibited high-level resistance to AZI with MIC values of >64 µg/mL), with MIC50 and MIC90 values of 1 µg/mL and 16 µg/mL, respectively. Interestingly, EZM was superior to AZI against the majority of the tested strains at approximately 4- to 64-fold greater potency in MIC50 over AZI. Additionally, AZM and EZM maintained their activity against azithromycin-resistant N. gonorrhoeae isolates (CDC 181, CDC 202, and WHO-V), indicating no cross-resistance between the CAIs AZM and EZM, and AZI.
However, EZM was not as potent against the panel tested as compared to the current standard drug of choice for gonorrhoea, ceftriaxone (CEF), which displayed an MIC50 value of 0.03 µg/mL and MIC90 value of 0.125 µg/mL, equating to 4- and 2-fold less potency for the two metrics. Nonetheless, these results indicate that EZM maintains sub-1 µg/mL antimicrobial activity, outperforms AZI, and is comparable to CEF although slightly less potent.
3.2. Intracellular target identification
Our group and others have reported previously on CAIs potential in targeting N. gonorrhoeae and suggest that the molecules could be targeting the intracellular N. gonorrhoeae α-carbonic anhydrase (NgCA)Citation20,Citation37. EZM is also a potent CAI of human carbonic anhydrases and likely maintains similar activity against NgCA. Thus, we performed an assay to test for N. gonorrhoeae susceptibility in CO2 saturating conditions. Since CO2 is the natural substrate of carbonic anhydrases, at higher levels in the bacterial culture, it will outcompete with the inhibitor for the substrate-binding siteCitation20,Citation78. Consequently, in these conditions, if the inhibitor is targeting the NgCA intracellularly, the bacteria should display reduced susceptibility. Similar to AZM, it was observed that N. gonorrhoeae strains displayed reduced susceptibility to EZM in presence of 5% CO2 (representative strains shown in , and data for all strains tested shown in Table S2). As a control the same assay was performed with azithromycin and ceftriaxone, two molecules with mechanisms of action not linked to CO2 metabolism, to ensure no unintended resistance was observed that may confound interpretation of the results observed for the CAIs. For both molecules, there was no observed change in susceptibility to N. gonorrhoeae between the normal and CO2 conditions providing confidence that the reduced susceptibility in the case of the CAIs could indeed be due to NgCA CO2 saturation. While these results suggest a high probability that NgCA is the intracellular target for CAIs further work is being performed to definitively link CAI engagement with NgCA.
Table 2. MICs of molecules under normal and CO2 conditions.
3.3. In vitro inhibition of carbonic anhydrases
EZM exhibited 16-fold improved MIC50 and MIC90 than AZM towards N. gonorrhoeae. Thus, we sought to assess whether the increased potency for EZM was a function of differing in vitro potencies against NgCA. Therefore, we collected inhibition constant (Ki) data in a CO2 hydration assay as previously describedCitation37. Interestingly, AZM displayed greater potency in terms of Ki versus NgCA (Ki = 74 nM) compared to EZM (Ki = 94 nM) by approximately 1.2-fold (). Consequently, the large difference in anti-gonococcal potency between EZM and AZM cannot be attributed to the in vitro inhibition of NgCA for AZM and EZM. For comparison, the inhibitory constants are also provided for both molecules against two highly expressed human carbonic anhydrase isoforms, hCA I and hCA II. These isoforms, like NgCA, are also α-carbonic anhydrase family enzymes and are relevant for the distribution and pharmacokinetics of carbonic anhydrase inhibitors as they are prevalent in the red blood cells of humansCitation55,Citation56. EZM potency favoured the human isoforms over NgCA, by more than 10-fold for hCA II and about 4-fold for hCA I, while AZM was more potent towards NgCA over hCA I but not hCA II.
Table 3. Inhibitory constants for AZM and EZM against NgCA and hCAs
3.4. Molecule accumulation in N. gonorrhoeae
Since NgCA inhibition did not provide further details about superiority in the anti-gonococcal activity for EZM over AZM, we sought to investigate whether the molecules exhibited differences in accumulation within this Gram-negative pathogen and if this factor could account for EZM’s superior anti-microbial activity. Therefore, we set out to quantify the accumulation levels of both AZM and EZM as well as tetracycline (positive control) using the procedure described by Richter et al.Citation70.
Quantitation of the molecules indicated that EZM rapidly accumulated and peaked at 21.1 2.2 nmol/109 CFU at the 30-min time-point then maintained a concentration around 20 nmol/109 CFU for the remainder of the 120-min duration. This accumulation equated to 3.6-fold greater accumulation compared to AZM (5.8
0.1 nmol/109 CFU) at 30 min, while the concentration for AZM steadily increased and peaked at 7.7 nmol/109 CFU at the 120-min time point ( and tabular data in Table S3). Given that the total dose of molecule equated to 50 nmol/109 CFUs in each sample the amount of EZM detected at the 30-min time point indicated approximately 40% of the total EZM in solution accumulated within the bacterial cell compared to approximately 11% of the total AZM in solution at 30 min. Tetracycline was used as a positive control and exhibited rapid accumulation followed by a steady decline in molecule retention over the course of 120 min. Given that EZM ranged from approximately 2.5 to 3.5-fold greater accumulation within N. gonorrhoeae ATCC700825, it could be reasonably deduced that the improved accumulation of EZM is a primary factor for its increased potency over AZM. Future studies will evaluate the difference in accumulation in strains where the MIC values exhibited a wider gap in potency between the two compounds to assess if there are greater differences in permeability, and retention, among these strains. Nonetheless, there appear to be physicochemical properties intrinsic to EZM that provide this molecule with favourable accumulation over AZM.
Figure 2. Drug accumulation in N. gonorrhoeae ATCC700825 over 120 min at pH 7.4. (A) Plot of accumulation in nmol/109 CFU over 120 min after treatment with AZM, EZM, and tetracycline. (B) Accumulation of each drug at each time point in ng/109 CFUs. Each drug was dosed at a concentration of 50 µM into 1 ml tubes containing 109 CFU of N. gonorrhoeae.

3.5. Time-kill and post-antibiotic effects for carbonic anhydrase inhibitors
The CAIs were assessed in killing kinetics assay and evaluated for post-antibiotic effects to further understand their antimicrobial properties. Recently we showed that AZM and analogs exhibit bacteriostatic effects against N. gonorrhoeaeCitation37. Unsurprisingly, the same bacteriostatic effect was observed for EZM (). Yet, both AZM and EZM were found to significantly reduce N. gonorrhoeae burden as compared to DMSO (negative control). After 24 h, EZM reduced N. gonorrhoeae burden by 0.4-log10 units. In addition, where at the 24-h time point the molecule provided approximately 2.5-log10-reduction in N. gonorrhoeae load compared to the DMSO-treated control. Azithromycin and ceftriaxone each exhibited bactericidal activity consistent with previous reportsCitation58,Citation79.
Figure 3. Time-kill assay of CAIs, azithromycin (AZI), and ceftriaxone (CEF) (n = 4, at 10 × MIC) against N. gonorrhoeae ATCC 700825. DMSO (vehicle) served as a negative control. The error bars represent standard deviation values for each test agent studied. The data were analysed via a two-way ANOVA with post-hoc Dunnett's test for multiple comparisons. An asterisk (*) indicates a statistically significant difference (P < 0.05) between treatment with drugs compared to DMSO treatment (negative control).

Next, the post-antibiotic effect (PAE), the period after complete removal of the drug in which an antibiotic effect is still observed, was determined for the CAIs and azithromycin. The PAE is important data that, in combination with in vivo pharmacokinetics, can inform the proper dosing regimenCitation80,Citation81. PAE was determined as previously describedCitation72,Citation73,Citation82. AZM displayed an observed PAE ranging from 2 to 4 h against N. gonorrhoeae strains tested while EZM exhibited a long PAE of at least 10 h against all the strains tested (). EZM also slightly outperformed azithromycin (PAE = 8 h) in its PAE. These results are consistent with the permeability data that suggests EZM maintains a high intracellular concentration for at least 2 h with little decline over that period. Although the accumulation assay currently has not been extended out to the 10-h timepoint tested in the PAE assay, the PAE data suggests EZM maintains appreciable levels of molecule inside the cell to maintain the antimicrobial effect for this period.
Table 4. Post-antibiotic effect of molecules against N. gonorrhoeae.
3.6. Frequency of spontaneous mutation
Given the promising results of CAIs, AZM and EZM, we sought to investigate the likelihood that N. gonorrhoeae will develop resistance to CAIs using the single-step spontaneous mutation assay and the results are presented in . No resistant mutants were isolated at concentrations of 5× and 10 × MIC in the presence of a high inoculum (5.42 × 109 CFU/mL) of N. gonorrhoeae CDC 202, indicating a frequency of mutation of < 5.42 × 1 0 −9. This result is comparable to that of azithromycin that exhibits low mutation frequency, as reported earlierCitation83. The frequency of mutation of rifampin (positive control) was higher (1.1–1.5 × 1.0−7), as previously reportedCitation58–60.
Table 5. Spontaneous mutation frequencies of AZM, EZM, and rifampicin against N. gonorrhoeae 202.
4. Discussion
In previous work, our group has reported the efficacy of AZM and AZM-based analogs against N. gonorrhoeae. In the present work, we have extended the effort to investigate another FDA-approved CAI, EZM, that displays antimicrobial activity against N. gonorrhoeae. While both AZM and EZM have been reported previously to possess some level of anti-gonococcal activityCitation20, the exact MIC values had not been reported. Thus, we set out to characterise the EZM’s antimicrobial activity against N. gonorrhoeae clinical isolates and compare it to AZM. The results presented herein show that EZM possessed superior antimicrobial potency towards N. gonorrhoeae compared to both AZM and azithromycin. For example, across a panel of 22 N. gonorrhoeae isolates, EZM’s MIC50 and MIC90 were 16-fold more potent than AZM and 8–64-fold more potent than AZI. The MIC50 for EZM was roughly 4-fold weaker than the current therapeutic option for gonorrhoea, ceftriaxone. Accordingly, this data suggests that EZM is comparable or better in terms of antimicrobial activity compared to the current, or former, treatments for the pathogen.
Since EZM was significantly more potent than AZM, even though they are hypothesised to be inhibiting at the same intracellular target, we sought to investigate the reason for this divergence in antimicrobial potency. We first evaluated both CAIs in vitro against NgCA to determine if a difference in biochemical inhibition may provide clues to EZM’s superior antimicrobial activity. Both CAIs are generally regarded to be essentially equipotent against human α-CA isoforms, with Ki values ranging from 0.8 to 250 nM across a panel of 12 human isoformsCitation84. We found that AZM is slightly more potent against NgCA compared to EZM (74 and 94 nM, respectively). Therefore, we conclude the increase in antimicrobial activity against N. gonorrhoeae strains for EZM could not be driven by binding to the proposed target, NgCA. We are continuing to pursue target engagement studies in the bacterium, beyond the culture in CO2 conditions reported here, to definitively identify NgCA as the intracellular target. However, at this point, we cannot rule out the possibility of a second intracellular target that may be more susceptible to EZM inhibition than AZM, which in turn, may increase the susceptibility of the pathogen to the drug. Additionally, it should be noted that the CAI sulphonamides (AZM and EZM) are structurally related to sulfa-drugs that inhibit dihydropteroate synthase (DHPS), which previously were effective anti-gonorrheal agentsCitation85. However, as noted previously by our group, these sulfa-drugs do not have activity against the N. gonorrhoeae strains testedCitation37. Therefore, neither AZM nor EZM are likely inhibiting DHPS as part of their antimicrobial mechanism of action. Nevertheless, further work is scheduled to further elucidate the mechanism of action of CAIs against N. gonorrhoeae.
Gram-negative pathogens are notoriously difficult to treat due to the presence of the outer membrane that provides a barrier to small molecules, and the presence of efflux pumps that expel molecules from the cell. Thus, much effort has been applied recently to elucidate the physicochemical properties of molecules that influence permeability, and retention, within Gram-negative bacteriaCitation70,Citation86–88. Richter et al. have established eNTRy rules for accumulation within Escherichia coli that suggest molecules with globularity < 0.25 (generally flat in nature), low flexibility (reduced number of rotatable bonds; < 5), and presence of a primary amine (positive charge in nature) all can increase accumulation within E. coliCitation70. However, these rules were defined using E. coli as the model system and may not be fully applicable to N. gonorrhoeae accumulation as we described in our prior publicationCitation37. Nonetheless, AZM and EZM do satisfy two of the criteria suggested by the eNTRy rules, those being of low globularity and low flexibility. Conversely, the CAIs do not possess a primary amine, or any positively charged group, a structural component that had a significant positive influence on accumulation within E. coli. Yet, they still exhibit antimicrobial potency against the bacterium suggesting intracellular accumulation is achieved. Therefore, we turned to investigate the role bacterial accumulation may have on both AZM and EZM concerning the anti-gonococcal activity. Previous analysis by O’Shea and Moser on the physicochemical properties of antimicrobial compounds available on the market suggests that Gram-negative active molecules generally possess lower polar surface area and fewer hydrogen bond donors and acceptorsCitation89. They also suggest that overall charge, or lack thereof, maybe a key element that influences their anti-Gram-negative activity. This was taken a step further by Richter et al. when they proposed the aforementioned eNTRy rules for Gram-negative accumulation and note the importance of a primary amine in the driving accumulation within the E. coli cellCitation70. These rules were developed and rationalised in E. coli that expresses the non-specific porin OmpF. N. gonorrhoeae contain two porins, PorA and PorB, that are structurally similar to OmpF (, overlay of OmpF; PDB: 1OPFCitation90 and PorB; PDB: 4AUICitation91) yet possess some key differences. For example, the general pore size for PorB compared to OmpF is relatively similar ()Citation91, with a measured distance at the constriction point for OmpF of 11.2 Å and 11.9 Å for PorB. Thus, we expect the globularity and rigidity metrics from the eNTRy rule to still apply to N. gonorrhoeae.
Figure 4. Comparison of E. coli porin OmpF and N. gonorrhoeae porin PorB. (A) Overlay of E. coli porin OmpF (yellow, PDB: 1OPF) and N. gonorrhoeae (cyan, PDB: 4AUI). (B) Side view cross-section of OmpF, porin constriction point depicted by a double-headed arrow with diameter of 11.2 Å. (C) Side view cross-section of PorB, porin constriction point depicted by the double-headed arrow with a diameter of 11.9 Å. (D) Surface electrostatic potential of OmpF, blue = positive charge and red = negative charge, arrow points to anionic surface patch. (E) Surface electrostatic potential of PorB, blue = positive charge and red = negative charge, arrow points to anionic surface patch. Overlays, surface representations, and electrostatic potentials are calculated in PyMol version 2.3.3 (Schrödinger, LLC). This figure was created on BioRender.com.

However, the charge distributions at the constriction points are quite different. As shown for OmpF, the pore contains a large cationic surface balanced by a large anionic surface (, black arrow pointing to anionic surface). Conversely, PorB also possesses a cationic surface but an anionic surface is reduced in size and polarity (, black arrow pointing to anionic surface). It has been previously proposed that the anionic surface in OmpF drives the cation selectivity through the porinCitation92 and this was rationalised as the basis for why primary amines on molecules enhance accumulation in E. coliCitation70,Citation88,Citation93. We observed that the anionic surface patch is reduced in PorB and this is in agreement with previous reports suggesting the charge distribution is less pronounced than that for OmpFCitation91. Thus, it stands to reason that the addition of a primary amine, or maintaining a net positive charge, may not be critical to molecule accumulation within N. gonorrhoeae. Conversely, the presence of a negative charge may also not be as detrimental to accumulation in N. gonorrhoeae as it is for E. coli. In fact, it has been reported previously that negatively charged antibiotics, such as penicillin G or cefotaxime, are capable of traversing PorBCitation94. Moreover, the current drug of choice for treating N. gonorrhoeae, ceftriaxone, is marketed as a di-sodium salt possessing a net charge of −2, yet is still capable of reaching its intracellular target.
Both AZM and EZM are negatively ionised at pH 7.4, although to different degrees. The pKa of the sulphonamides vary due to the makeup of the heterocyclic ring. AZM possesses two acidic protons, the amide proton with a predicted pKa of 6.5 and the sulphonamide that has an experimentally determined pKa of 7.2Citation95. These combined pKa’s indicate that AZM would be approximately 95% negatively ionised at pH 7.4 (MarvinSketch prediction, Ver 21.8, ChemAxon). Conversely, EZM possesses only one acidic proton on the sulphonamide with a pKa of 8.0Citation96 that would yield 20% negatively ionised molecule in solution at pH 7.4. Additionally, EZM is more lipophilic with a predicted LogD7.4 of 1.4 compared to a LogD7.4 of −2.1 for AZM (MarvinSketch prediction, Ver 21.8, ChemAxon). In terms of molecular weight, globularity, and rigidity, both AZM and EZM are comparable (). Thus, given that the interior of PorB is more hydrophobic in nature compared to the OmpF core, we hypothesise that the lower percentage of ionised molecule and increased lipophilicity of EZM may facilitate its improved accumulation within the N. gonorrhoeae bacterial cell. This analysis does not account for the effect that these physicochemical properties may have on drug efflux and future studies are planned to investigate the effect of physicochemical properties of molecules on accumulation in N. gonorrhoeae.
Figure 5. Physicochemical and eNTRy rules properties for AZM and EZM. Green text indicates favourable properties for E. coli accumulation defined by Richter et al.Citation70 and calculated by entry-way.org.

Extending the analysis, the combination of improved intracellular accumulation and tight binding affinity is likely contributing to the prolonged post-antibiotic effects of EZM. Once EZM is within the cell, the double-digit nanomolar Ki value for EZM to NgCA would contribute to keeping the molecule inside the bacterial cell as the drug would spend more time in a bound state as opposed to unbound that is subject to diffusion or expulsion out of the cell. Consequently, these properties of EZM could lead to providing the antimicrobial effect for extended periods even after the drug has been washed away, resulting in prolonged PAE. However, the accumulation study was only performed throughout 2 h while the PAE was observed up to 10 h. Future experiments will investigate bacterial accumulation at time points beyond 2 h to determine if and when intracellular EZM levels begin to regress towards baseline as well as evaluate physicochemical properties that may improve accumulation in N. gonorrhoeae and how those might differ from E. coli.
It is also important to assess the frequency of development of resistance against novel antibacterial agents as an important step in the drug development process. Thus, we attempted to generate N. gonorrhoeae mutants that are resistant to either AZM or EZM using a single-step spontaneous mutation assay. At a high inoculum size (∼109 CFU/mL), N. gonorrhoeae mutants exhibiting resistance to either AZM or EZM could not be isolated. This indicates a low likelihood of the emergence of rapid resistance to CAIs although further work is being performed on this front. On the other hand, resistance was developed against rifampicin, which is consistent with previous reportsCitation58–60,Citation77.
The possibility of targeting the bacterial carbonic anhydrase for anti-gonococcal activity is further bolstered by this new data. Although EZM is FDA-approved, much less is reported in terms of the drugs in vivo pharmacokinetics and safety. These attributes would need to be further explored to realise the potential of EZM-based molecules as new antibiotics. Nonetheless, the combined potent antimicrobial activity, extended post-antibiotic effect, and low potential of resistance development towards EZM suggest the scaffold is worthy of additional evaluation as a potential new class of inhibitors to treat drug-resistant N. gonorrhoeae.
5. Conclusion
In this study, our group has characterised the anti-gonococcal activity for two FDA-approved carbonic anhydrase inhibitors, AZM and EZM. Building from previous reports and work from our team, we have shown that EZM displays a potent antimicrobial activity against N. gonorrhoeae clinical isolates with an MIC50 value of 0.125 µg/mL and MIC90 value of 0.25 µg/mL, which was 16-fold more potent than AZM. EZM also outperformed azithromycin in most strains and was about 4-fold less active than the current drug of choice, ceftriaxone. Consistent with our previous report with AZM-based analogs, we observed that EZM was bacteriostatic against the strains tested and likely inhibits the intracellular N. gonorrhoeae carbonic anhydrase as the mode of action. Both AZM and EZM displayed comparable sub-100 nM Ki values against NgCA. Additionally, it was shown that EZM accumulated within N. gonorrhoeae bacteria up to 3.5-fold better than AZM and was hypothesised that reduced ionisation, combined with higher lipophilicity, may enhance accumulation in this particular Gram-negative pathogen. The significant increase in intracellular accumulation likely explains both the superior activity and the extended post-antibiotic effect observed for EZM. Furthermore, AZM and EZM exhibited low potential for the development of bacterial resistance against them. Taken together, the data presented suggests the potential for EZM-based bacterial CAIs that warrant further investigation for translation into effective anti-N. gonorrhoeae agents.
Author contributions
The manuscript was written through contributions of all authors. All authors have given approval to the final version of the manuscript.
Supplemental Material
Download PDF (128 KB)Acknowledgement
The research program was partially funded by a Purdue Institute for Drug Discovery Programmatic Grant (M.N.S and D.P.F.), NIH/NIAID 1R01AI148523 (M.N.S and D.P.F.).
Disclosure statement
No potential conflict of interest was reported by the author(s).
Additional information
Funding
References
- Newman L, Rowley J, Vander HS, et al. Global estimates of the prevalence and incidence of four curable sexually transmitted infections in 2012 based on systematic review and global reporting. PLOS One 2015;10:e0143304.
- World Health Organization. Multi-drug resistant gonorrhoea Fact Sheet. World Health Organization Fact Sheets. Geneva, Switzerland 2020. https://www.who.int/news-room/fact-sheets/detail/multi-drug-resistant-gonorrhoea
- Bowen VB, Braxton J, Davis DW, et al. Sexually transmitted disease surveillance 2018. Atlanta (GA): Center for Disease Control and Prevention; 2019.
- Rice PA, Shafer WM, Ram S, Jerse AE. Neisseria gonorrhoeae: drug resistance, mouse models, and vaccine development. Annual Rev Microbiol 2017;71:665–86.
- Wi T, Lahra MM, Ndowa F, et al. Antimicrobial resistance in Neisseria gonorrhoeae: Global surveillance and a call for international collaborative action. PLOS Med 2017;14:e1002344–16.
- Derbie A, Mekonnen D, Woldeamanuel Y, Abebe T. Azithromycin resistant gonococci: a literature review. Antimicrob Resist Infect Control 2020;9:1–7.
- Gernert KM, Seby S, Schmerer MW, et al. Azithromycin susceptibility of Neisseria gonorrhoeae in the USA in 2017: a genomic analysis of surveillance data. Lancet Microbe 2020;1:e154–e164.
- Cyr SS, Barbee L, Workowski KA, et al. Update to CDC's treatment guidelines for gonococcal infection, 2020. MMWR Morb Mortal Wkly Rep 2020;69:1911–6.
- Fifer H, Natarajan U, Jones L, et al. Failure of dual antimicrobial therapy in treatment of gonorrhea. New Engl J Med 2016;374:2504–6.
- Cámara J, Serra J, Ayats J, et al. Molecular characterization of two high-level ceftriaxone-resistant Neisseria gonorrhoeae isolates detected in Catalonia, Spain. J Antimicrob Chemother 2012;67:1858–60.
- Golparian D, Ohlsson AK, Janson H, et al. Four treatment failures of pharyngeal gonorrhoea with ceftriaxone (500 mg) or cefotaxime (500 mg), Sweden, 2013 and 2014. Eurosurveillance 2014;19:20862.
- Centers for Disease Control and Prevention. Antibiotic resistance threats in the United States, 2019. Atlanta, GA: U.S. Department of Health and Human Services 2019. https://www.cdc.gov/drugresistance/pdf/threats-report/2019-ar-threats-report-508.pdf
- Bolan G, Sparling PF, Wasserheit JN. The emerging threat of untreatable gonococcal infection. N Engl J Med 2012;366:485–7.
- Farha MA, Brown ED. Drug repurposing for antimicrobial discovery. Nat Microbiol 2019;4:565–77.
- Ashburn TT, Thor KB. Drug repositioning: identifying and developing new uses for existing drugs. Nat Rev Drug Discov 2004;3:673–83.
- Chong CR, Sullivan DJ Jr. New uses for old drugs. Nature 2007;448:645–6.
- Takeuchi H, Supuran C, Onishi S, Nishimori I. The alpha and beta classes carbonic anhydrases from Helicobacter pylori as novel drug targets. Current Pharmaceutical Design 2008;14:622–30.
- Rahman MM, Tikhomirova A, Modak JK, et al. Antibacterial activity of ethoxzolamide against Helicobacter pylori strains SS1 and 26695. Gut Pathogens 2020;12:20–7.
- Modak JK, Tikhomirova A, Gorrell RJ, et al. Anti-Helicobacter pylori activity of ethoxzolamide. J Enzyme Inhib Med Chem 2019;34:1660–7.
- Sanders E, Maren TH. Inhibition of carbonic anhydrase in Neisseria: effects on enzyme activity and growth. Mol Pharmacol 1967;3:204–15.
- Nafi BM, Miles RJ, Butler LO, et al. Expression of carbonic anhydrase in neisseriae and other heterotrophic bacteria. J Med Microbiol 1990;32:1–7.
- Johnson BK, Colvin CJ, Needle DB, et al. The carbonic anhydrase inhibitor ethoxzolamide inhibits the Mycobacterium tuberculosis PhoPR regulon and Esx-1 secretion and attenuates virulence. Antimicrobial Agents Chemother 2015;59:4436–45.
- Supuran CT, Capasso C. Antibacterial carbonic anhydrase inhibitors: an update on the recent literature. Expert Opin Ther Pat 2020;30:963–82.
- Supuran CT. Bacterial carbonic anhydrases as drug targets: toward novel antibiotics? Front Pharmacol 2011;2:34–6.
- Capasso C, Supuran CT. An overview of the alpha-, beta- and gamma-carbonic anhydrases from Bacteria: Can bacterial carbonic anhydrases shed new light on evolution of bacteria? J Enzyme Inhib Med Chem 2015;30:325–32.
- Modak JK, Modakh JK, Liu YC, et al. Structural basis for the inhibition of Helicobacter pylori α-carbonic anhydrase by sulfonamides. PLOS ONE 2015;10:e0127149.
- Modak JK, Liu YC, Supuran CT, Roujeinikova A. Structure-activity relationship for sulfonamide inhibition of Helicobacter pylori α-carbonic anhydrase. J Med Chem 2016;59:11098–109.
- Mancuso F, De Luca L, Angeli A, et al. In silico-guided identification of new potent inhibitors of carbonic anhydrases expressed in Vibrio cholerae. ACS Med Chem Letters 2020;11:2294–9.
- Del Prete S, Nocentini A, Supuran CT, Capasso C. Bacterial ι-carbonic anhydrase: a new active class of carbonic anhydrase identified in the genome of the Gram-negative bacterium Burkholderia territorii. J Enzyme Inhib Med Chem 2020;35:1060–8.
- Del Prete S, Vullo D, Di P, et al. Sulfonamide inhibition profile of the γ-carbonic anhydrase identified in the genome of the pathogenic bacterium Burkholderia pseudomallei the etiological agent responsible of melioidosis. Bioorganic Med Chem Letters 2017;27:490–5.
- Burghout P, Cron LE, Gradstedt H, et al. Carbonic anhydrase is essential for Streptococcus pneumoniae growth in environmental ambient air. J Bacteriol 2010;192:4054–62.
- Supuran CT, Capasso C. An overview of the bacterial carbonic anhydrases. Metabolites 2017;7:56–2139.
- Capasso C, Supuran CT. Bacterial, fungal and protozoan carbonic anhydrases as drug targets. Expert Opin Ther Targets 2015;19:1689–704.
- Kaur J, Cao X, Abutaleb NS, et al. Optimization of acetazolamide-based scaffold as potent inhibitors of vancomycin-resistant enterococcus. J Med Chem 2020;63:9540–62.
- Abutaleb NS, Elkashif A, Flaherty DP, Seleem MN. In vivo antibacterial activity of acetazolamide. Antimicrob Agents Chemother 2021;65:e01715–e01720.
- Abutaleb NS, Elhassanny AEM, Flaherty DP, Seleem MN. In vitro and in vivo activities of the carbonic anhydrase inhibitor, dorzolamide, against vancomycin-resistant enterococci. PeerJ 2021;9:e11059.
- Hewitt CS, Abutaleb NS, Elhassanny AEM, et al. Structure-activity relationship studies of acetazolamide-based carbonic anhydrase inhibitors with activity against Neisseria gonorrhoeae. ACS Infect Dis 2021;7:1969–84.
- Drance SM. Ethoxzolamide (cardrase) in the management of chronic simple glaucoma. Arch Ophthalmol 1960;64:433–7.
- Maren TH, Brechue WF, Bar-Ilan A. Relations among IOP reduction, ocular disposition and pharmacology of the carbonic anhydrase inhibitor ethoxzolamide. Exp Eye Res 1992;55:73–9.
- Supuran CT, Altamimi ASA, Carta F. Carbonic anhydrase inhibition and the management of glaucoma: a literature and patent review 2013-2019. Expert Opin Ther Pat 2019;29:781–92.
- Gordon DM. Ethoxzolamide; a new carbonic anhydrase inhibitor. Am J Ophthalmol 1958;46:41–4.
- Lim L, Foldvary N, Mascha E, Lee J. Acetazolamide in women with catamenial epilepsy. Epilepsia 2001;42:746–9.
- Patsalos PN. The epilepsy prescriber’s guide to antiepileptic drugs. Cambridge (UK): Cambridge University Press; 2018.
- Van Berkel MA, Elefritz JL. Evaluating off-label uses of acetazolamide. Bull Am Soc Hosp Pharm 2018;75:524–31.
- Ritschel WA, Paulos C, Arancibia A, et al. Pharmacokinetics of acetazolamide in healthy volunteers after short- and long-term exposure to high altitude. J Clin Pharmacol 1998;38:533–9.
- Low EV, Avery AJ, Gupta V, et al. Identifying the lowest effective dose of acetazolamide for the prophylaxis of acute mountain sickness: systematic review and meta-analysis. BMJ 2012;345:e6779.
- World Health Organization. WHO Model List of Essential Medicines. Essential medicines and health products. 2017; (August):1–39.
- Dollery CT, Boobis AR. Therapeutic drugs, 2nd Ed. London (UK): Churchill Livingstone; 1999.
- Ten Hove MW, Friedman DI, Patel AD, Irrcher I, et al. Safety and tolerability of acetazolamide in the idiopathic intracranial hypertension treatment trial. J Neuro-Ophthalmol 2016;36:13–9.
- Lubow M, Kuhr L. Pseudotumor cerebri: comments on practical management. Neuro-ophthalmology 1976;9:199–206.
- Tomsak RL, Niffenegger AS, Remler BF. Treatment of pseudotumor cerebri with Diamox (acetazolamide). J Neuro-Ophthalmol 1988;8:93–8.
- Hampson AJ, Babalonis S, Lofwall MR, et al. A pharmacokinetic study examining acetazolamide as a novel adherence marker for clinical trials. J Clin Psychopharmacol 2016;36:324–32.
- Wistrand PJ. The use of carbonic anhydrase inhibitors in ophthalmology and clinical medicine. Annals NY Acad Sci 1984;429:609–19.
- Wistrand PJ, Rawls JA Jr, Maren TH. Sulphonamide carbonic anhydrase inhibitors and intra-ocular pressure in rabbits. A comparison between in vitro and in vivo activities based on tissue distributions and physical and chemical properties of nine compounds. Acta Pharmacol Toxicol 1961;17:337–55.
- Wallace SM, Reigelman S. Uptake of acetazolamide by human erythrocytes in vitro. J Pharm Sci 1977;66:729–31.
- Wallace SM, Shah VP, Riegelman S. GLC analysis of acetazolamide in blood, plasma, and saliva following oral administration to normal subjects. J Pharm Sci 1977;66:527–30.
- Friedland BR, Maren TH, Carbonic anhydrase: pharmacology of inhibitors and treatment of glaucoma. In: Pharmacology of the Eye. Handbook of experimental pharmacology, vol. 69. Berlin, Heidelberg: Springer; 1984. p. 279–309.
- Alhashimi M, Mayhoub A, Seleem MN. Repurposing salicylamide for combating multidrug-resistant Neisseria gonorrhoeae. Antimicrob Agents Chemother 2019;63:e01225–19.
- Seong YJ, Alhashimi M, Mayhoub A, et al. Repurposing fenamic acids drugs to combat multidrug-resistant Neisseria gonorrhoeae. Antimicrob Agents Chemother 2020;64:e02206–19.
- Elkashif A, Seleem MN. Investigation of auranofin and gold-containing analogues antibacterial activity against multidrug-resistant Neisseria gonorrhoeae. Sci Rep 2020;10:5602–10.
- Petreni A, De Luca V, Scaloni A, et al. Anion inhibition studies of the Zn(II)-bound ι-carbonic anhydrase from the gram-negative bacterium Burkholderia territorii. J Enzyme Inhib Med Chem 2021;36:372–6..
- Vullo D, Del Prete S, Osman SM, et al. Sulfonamide inhibition studies of the γ-carbonic anhydrase from the oral pathogen Porphyromonas gingivalis. Bioorganic Med Chem Lett 2014;24:240–4.
- Turkmen H, Durgun M, Yilmaztekin S, et al. Carbonic anhydrase inhibitors. Novel sulfanilamide/acetazolamide derivatives obtained by the tail approach and their interaction with the cytosolic isozymes I and II, and the tumor-associated isozyme IX. Bioorganic Med Chem Lett 2005;15:367–72.
- Di Cesare Mannelli L, Micheli L, Carta F, et al. Carbonic anhydrase inhibition for the management of cerebral ischemia: in vivo evaluation of sulfonamide and coumarin inhibitors. J Enzyme Inhib Med Chem 2016;31:894–9.
- Nocentini A, Hewitt CS, Mastrolorenzo MD, et al. Anion inhibition studies of the α-carbonic anhydrases from Neisseria gonorrhoeae. J Enzyme Inhib Med Chem 2021;36:1061–6.
- Yung-Chi C, Prusoff WH. Relationship between the inhibition constant (KI) and the concentration of inhibitor which causes 50 per cent inhibition (I50) of an enzymatic reaction. Biochem Pharmacol 1973;22:3099–108.
- Hamann HJ, Abutaleb NS, Pal R, et al. β,γ-Diaryl α-methylene-γ-butyrolactones as potent antibacterials against methicillin-resistant Staphylococcus aureus. Bioorganic Chem 2020;104:104183–90.
- Elsebaei MM, Abutaleb NS, Mahgoub AA, et al. Phenylthiazoles with nitrogenous side chain: an approach to overcome molecular obesity. Eur J Med Chem 2019;182:111593–603.
- Abutaleb NS, Seleem MN. Repurposing the antiamoebic drug diiodohydroxyquinoline for treatment of Clostridioides difficile infections. Antimicrob Agents Chemother 2020;64:e02115–19.
- Richter MF, Drown BS, Riley AP, et al. Predictive compound accumulation rules yield a broad-spectrum antibiotic. Nature 2017;545:299–304.
- Parker EN, Drown BS, Geddes EJ, et al. Implementation of permeation rules leads to a FabI inhibitor with activity against gram-negative pathogens. Nat Microbiol 2020;5:67–75.
- Mohammad H, Abutaleb NS, Dieterly AM, et al. Evaluation of ebselen in resolving a methicillin-resistant Staphylococcus aureus infection of pressure ulcers in obese and diabetic mice. PLOS One 2021;16:e0247508.
- Shahin IG, Abutaleb NS, Alhashimi M, et al. Evaluation of N-phenyl-2-aminothiazoles for treatment of multi-drug resistant and intracellular Staphylococcus aureus infections. Eur J Med Chem 2020;202:112497.
- Pankuch GA, Jacobs MR, Appelbaum PC. Postantibiotic effects of garenoxacin (BMS-284756) against 12 gram-positive or -negative organisms. Antimicrob Agents Chemother 2003;47:1140–2.
- Butler MM, Waidyarachchi SL, Connolly KL, et al. Aminomethyl spectinomycins as therapeutics for drug-resistant gonorrhea and chlamydia coinfections. Antimicrob Agents Chemother 2018;62:e00325–18.
- Naclerio GA, Abutaleb NS, Li D, et al. Ultrapotent inhibitor of clostridioides difficile growth, which suppresses recurrence in vivo. J Med Chem 2020;63:11934–44.
- Thangamani S, Mohammad H, Abushahba MFN, et al. Antibacterial activity and mechanism of action of auranofin against multi-drug resistant bacterial pathogens. Sci Rep 2016;6:22571.
- Chirică LC, Elleby B, Jonsson BH, Lindskog S. The complete sequence, expression in Escherichia coli, purification and some properties of carbonic anhydrase from Neisseria gonorrhoeae. Eur J Biochem 1997;244:755–60.
- Perry TR, Schentag JJ. Clinical use of ceftriaxone. Clin Pharmacokinet 2001;40:685–94.
- Spivey JM. The postantibiotic effect. Clin Pharm 1992;11:865–75.
- Cars O, Odenholt-Tornqvist I. The post-antibiotic sub-MIC effect in vitro and in vivo. J Antimicrob Chemother 1993;31:159–66.
- Mohammad H, Abutaleb NS, Seleem MN. Auranofin rapidly eradicates methicillin-resistant Staphylococcus aureus (MRSA) in an infected pressure ulcer mouse model. Sci Rep 2020;10:7251–8.
- Binet R, Maurelli AT. Frequency of development and associated physiological cost of azithromycin resistance in Chlamydia psittaci 6BC and C. trachomatis L2. Antimicrob Agents Chemother 2007;51:4267–75.
- Supuran CT. Carbonic anhydrases: novel therapeutic applications for inhibitors and activators. Nat Rev Drug Discovery 2008;7:168–81.
- Woods DD. The relation of p-aminobenzoic acid to the mechanism of the action of sulphanilamide. Br J Exp Pathol 1940;21:74–90.
- Davis TD, Gerry CJ, Tan DS. General platform for systematic quantitative evaluation of small-molecule permeability in bacteria. ACS Chem Biol 2014;9:2535–44.
- Zhao S, Adamiak JW, Bonifay V, et al. Defining new chemical space for drug penetration into gram-negative bacteria. Nat Chem Biol 2020;16:1293–302.
- Richter MF, Hergenrother PJ. The challenge of converting gram-positive-only compounds into broad-spectrum antibiotics. Annals NY Acad Sci 2019;1435:18–38.
- O'Shea R, Moser HE. Physicochemical properties of antibacterial compounds: implications for drug discovery. J Med Chem 2008;51:2871–8.
- Cowan SW, Garavito RM, Jansonius JN, et al. The structure of OmpF porin in a tetragonal crystal form. Structure 1995;3:1041–50.
- Zeth K, Kozjak-Pavlovic V, Faulstich M, et al. Structure and function of the PorB porin from disseminating Neisseria gonorrhoeae. Biochem J 2013;449:631–42.
- Danelon C, Suenaga A, Winterhalter M, Yamato I. Molecular origin of the cation selectivity in OmpF porin: single channel conductances vs. free energy calculation. Biophys Chem 2003;104:591–603.
- Acosta-Gutiérrez S, Ferrara L, Pathania M, et al. Getting drugs into gram-negative bacteria: rational rules for permeation through general porins. ACS Infect Dis 2018;4:1487–98.
- Olesky M, Zhao S, Rosenberg RL, Nicholas RA. Porin-mediated antibiotic resistance in Neisseria gonorrhoeae: ion, solute, and antibiotic permeation through PIB proteins with penB mutations. J Bacteriol 2006;188:2300–8.
- Maynard RL. The Merck index: 1996. Occup Environ Med 1997;54:288.
- Remko M, Von Der Lieth CW. Theoretical study of gas-phase acidity, pKa, lipophilicity, and solubility of some biologically active sulfonamides. Bioorg Med Chem 2004;12:5395–5403.