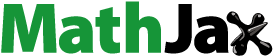
Abstract
We synthesised and screened 18 aromatic derivatives of guanylhydrazones and oximes aromatic for their capacity to bind to dengue virus capsid protein (DENVC). The intended therapeutic target was the hydrophobic cleft of DENVC, which is a region responsible for its anchoring in lipid droplets in the infected cells. The inhibition of this process completely suppresses virus infectivity. Using NMR, we describe five compounds able to bind to the α1-α2 interface in the hydrophobic cleft. Saturation transfer difference experiments showed that the aromatic protons of the ligands are important for the interaction with DENVC. Fluorescence binding isotherms indicated that the selected compounds bind at micromolar affinities, possibly leading to binding-induced conformational changes. NMR-derived docking calculations of ligands showed that they position similarly in the hydrophobic cleft. Cytotoxicity experiments and calculations of in silico drug properties suggest that these compounds may be promising candidates in the search for antivirals targeting DENVC.
1. Introduction
The dengue virus (DENV) is a mosquito-borne virus that belongs to the Flavivirus genus, together with other important human pathogens, such as Zika, yellow fever, West Nile, and Japanese encephalitis viruses. The incidence of DENV is increasing over the years by the mosquito vector spreading, which can be associated with urbanisation, global warming, population growth, and an increasing number of international travels, together with a decrease in effective means for mosquito controlCitation1. The disease caused by DENV may vary from a mild fever to life-threatening severe diseases, known as dengue haemorrhagic fever (DHF) and dengue shock syndrome (DSS), which are characterised by an increase in vascular endothelial permeability that leads to plasma leakage, and may evolve to a fatal hypovolemic shockCitation2.
DENV capsid protein (DENVC) forms a symmetric dimer in solution, presenting 8 intertwined α-helices (4 per subunit)Citation3. DENVC has an essential role in the viral assembly. It is involved with the packaging of the viral genome forming the nucleocapsid (NC) coreCitation4. The protein is dominated by quaternary contacts involving two pairs of antiparallel helices (α2-α2' and α4-α4') that form most of the dimer interface. The α4-α4' exposed surface has the highest density of positive charges and it is the putative RNA binding site. The α2-α2' is nonpolar and along with α1 and α1' form a concave-shaped hydrophobic cleft, that interacts with the viral membrane. The dynamics, size, and orientation of α1 and α1' regulate the exposure of the hydrophobic surfaceCitation5,Citation6. Among flaviviruses, α2-α2' is the most conserved region of protein C, helping in the formation of a conserved hydrophobic surface (π-stacked Phe53/Phe53’, Phe47, Leu54, and Leu57) and a conserved aromatic backbone (π-stacked Phe56/Phe84’ and Phe56’/Phe84)Citation6. Samsa and colsCitation7 showed that DENVC associates with lipid droplets (LD) during viral replication, being this event essential to the virus assembly and infectivity. The interaction to LD involves the hydrophobic cleft of the DENVC and the conserved segment 14–23 of the intrinsically disordered N-terminalCitation8,Citation9. Mutations in hydrophobic amino acid residues at the hydrophobic cleft completely abolished the virus infectivity, which makes it an important unexplored therapeutical targetCitation7.
Some antiviral compounds were identified as being able of inhibiting in vitro and in vivo DENV replication. Different targets were used in those studies, such as viral envelope (E) protein, inhibiting viral-induced membrane fusionCitation10; NS2B-NS3, inhibiting viral protease activityCitation11; NS4B, possibly inhibiting its interaction with the viral NS3 helicase domainCitation12; NS5 methyltransferase activityCitation13 or RNA polymerase activityCitation14,Citation15, inhibiting viral RNA synthesis; and virus-specific RNA translationCitation16. Regarding DENVC, the small compound ST-148 was identified as a ligand of C proteinCitation17,Citation18, with a proposed mechanism of capsid assembly inhibition through its binding to the protein hydrophobic cleft, leading to the formation of a kissing tetramer (dimer of dimers)Citation19. Despite some of these ligands having shown valid antiviral activity against DENV replication, until now, to our knowledge, there are no interaction studies by NMR and no approved antiviral drug for the treatment of DENV infections.
In this work, we screened by NMR 18 aromatic compounds (, derivatives of guanylhydrazones and oximes) for their capacity to bind DENVC. The intended target was the hydrophobic cleft. We selected 5 leads that bind to the hydrophobic cleft at the interface between α1/α1’ and α2/α2’. We used ligand-based NMR methods (saturation transfer difference, STD, and transverse relaxation, T2) and protein-based 15 N-HSQC for screenings. In silico calculations were also performed to rationalise the results. Structural models of the protein-ligand complexes generated by NMR-derived molecular docking showed that the selected compounds could bind to the two symmetric pockets in the hydrophobic cleft. Pharmacokinetics analyses showed the tested compounds are promising drugs, with low cytotoxic effects on two different cell lines (Huh7 and A549). We successfully addressed the hydrophobic cleft of DENVC as a structural target for the development of potential antiviral compounds.
Figure 1. Organic compounds used in this work: (1) 5-chloro-salicylaldehyde-guanylhydrazone, (2) 5-bromo-pyridine-2-guanylhydrazone, (3) thiophene-2-guanylhydrazone, (4) 5-bromo-thiophene-2-guanylhydrazone, (5) 5-nitro-thiophene-2-guanylhydrazone, (6) 1-methyl-imidazole-5-guanylhydrazone, (7) 4-methyl-imidazole-5-guanylhydrazone, (8) 4–(4-morpholinyl)benzyl-guanylhydrazone, (9) 2,4-dinitro-benzyloxime, (10) 2-chloro-3,6-difluoro-benzyloxime, (11) 2-chloro-5-nitro-benzyloxime, (12) 2-hydroxy-5-chloro-benzyloxime, (13) 6-chloro-pyridine-3-oxime, (14) 5-bromo-pyridine-2-oxime, (15) 5-bromo-thiophene-2-oxime, (16) 5-nitro-thiophene-2-oxime, (17) 5-nitro-furfural-2-oxime, and (18) 4–(4-morphonylyl)benzyloxime.

2. Materials and methods
2.1. Compounds selection
The selection of the compounds used in this work () was based on the fact that aromatic and amphipathic compounds are expected to interact with the nonpolar exposed residues and the aromatic backbone in DENVC hydrophobic cleft. In addition, it has been taken into account fact that derivatives of guanylhydrazones and oximes are widely studied due to their broad biological activity, as antitumorCitation20,Citation21, antibacterialCitation22,Citation23, antifungalCitation24,Citation25, antiviralCitation26,Citation27, antiprotozoalCitation28,Citation29, and anti-inflammatoryCitation30,Citation31 drugs.
2.2. Synthesis and characterisation of the organic compounds
All reagents were purchased from Sigma-Aldrich (Brazil) and the solvents from VETEC (Brazil), used without further purification. Reactions were monitored by TLC using DC Alufolien Kieselgel 60 F254 (Merck, Darmstadt, Germany). Melting points (MP) were determined on a Melt-Temp II with a previously calibrated thermometer. Infra-red spectra (IR) were obtained on a Shimadzu Prestige 21. For the characterisation of compounds, all NMR experiments (1H NMR, 13 C NMR, ATP, gHSQC, gHMBC) were performed at 298 K on a 14.1 T Premium COMPACT™ (600 MHz for proton, software VNMRJ version 3.2) spectrometer using a 5 mm NMR probe and dimethylsulfoxide-d6 (DMSO-d6) as solvent and reference. The data of yield, melting point, IR and NMR spectra (Figures S1–S54), and spectral assignment are available in the Supplementary Information.
2.2.1. General procedure for the synthesis of guanilhydrazones (1–8):
Aminoguanidine hydrochloride (1.2 mmols) dissolved in 20 ml of 95% ethanol, the corresponding aldehyde (1 mmol) and 2 drops of HCl (0.6 M) were placed in a round bottom flask. The solution was kept under reflux and stirring. The solid obtained after eliminating the solvent under vacuum was solubilised in distilled water and extracted with dichloromethane (5 × 20 ml). The product was recrystallized from ethanolCitation32.
2.2.2. General procedure for the synthesis of oximes (9–18):
Hydroxylamine hydrochloride (4 mmols) dissolved in a mixture of 10 ml of ethanol and 3 ml of water was placed in a round bottom flask. The corresponding aldehyde (2 mmols) was added to the solution, which was kept under stirring. The solid obtained after eliminating the solvent under vacuum was washed with distilled cool water. The oximes were recrystallized from ethanol or methanolCitation32. The compounds 1, 3, 12, 15, 16, and 17 were synthesised as previously reportedCitation33–38. The synthesis routes were the same as published, optimising the quantities of the reagents and time of reaction.
2.3. DENEC expression and purification
DENVC from serotype 2, strain New Guinea, was expressed in Escherichia coli BL21(DE3)plysS cells with pET3a (Novagen) expression plasmid in M9 minimal medium, as previously describedCitation3,Citation39. For expression of the 15 N-labelled protein, the minimal medium containing 15NH4Cl 1 g.L−1 (Sigma Aldrich) was used. The DENVC was purified using a heparin column (GE Healthcare LifeSciences) at 5 ml/min flow rate and the bound protein was eluted with an increasing NaCl concentration gradient (0.5–2.0 M). The fractions containing the DENVC were confirmed by 15% SDS-PAGE. The DENVC was dialysed against phosphate-buffered saline (PBS) with a Centricon instrument (Millipore) and stored at −20 °C.
2.4. Ligand-protein interaction studies by NMR
Stock solutions of compounds 1–8 were prepared in H2O. Due to the low solubility in water, stock solutions of compounds 9–18 were prepared in DMSO-d6. For the NMR experiments, samples were prepared using the necessary amount of stock solution in 55 mM PBS, 200 mM NaCl, 2 mM EDTA, 10% (v/v) D2O (Cambridge Isotope Laboratories) as buffer solution at pH 6.0.
All experiments were acquired using the samples in the presence and absence of DENVC, with the final concentration of 10 μM for the protein and 1 mM for ligands (100-fold excess). For compounds 9–18, the final concentration of DMSO-d6 was 5% (v/v). All experiments were performed at 308 K, using a 5 mm NMR probe. Spectral data were processed with Topspin 2.1 (Biospin; Bruker).
Initially, the saturation-transfer difference (STD) experiments were performed in pools of 4 or 5 compounds, using STDdiff pulse sequence, 8 scans, 4 dummy scans, off-resonance irradiation at −6000 Hz, and on-resonance irradiation at 124.7, 746, and 2688 Hz, on a Bruker Avance DRX 400 MHz (Bruker Biospin, Germany). For those compounds that showed interaction, new STD spectra were acquired for each compound separately under the same previously described conditions on a Bruker Ascend 500 MHz spectrometer (Bruker Biospin, Germany).
The 1H NMR chemical shift variations were recorded using zgesgp pulse sequence (excitation sculpting for water suppression)Citation40 with 32 scans and 8 dummy scans. DOSY experiments were acquired using bipolar gradients (stebpgp pulse sequence) with 128 scans, 4 dummy scans, 20 ms of diffusion time (big delta), and 10 ms of gradient pulse (little delta). Both experiments were performed on a Bruker Avance DRX 400 MHz (Bruker Biospin, Germany).
The diffusion coefficients calculations were calculated from the adjustment of curves using the following fitting equation, where A and A0 are the areas of the NMR signal in the presence and absence of external gradient pulses, respectively; D is the diffusion coefficient; γ is the gyromagnetic ratio of the observed nucleus; g is the gradient strength; Δ is the diffusion time; δ is the length of the gradient.
(1)
(1)
Relaxation times were acquired on a Bruker Ascend 500 MHz spectrometer (Bruker Biospin, Germany) and performed in triplicate with a recycle delay of 10 s. T1 relaxation time was measured using the inversion recovery pulse sequence and T2 relaxation time by Carr-Purcell-Meiboom-Gill (CPMG) pulse sequence.
In addition, protein-ligand studies for determining the binding sites of the protein were performed only for those ligands that showed interaction by STD. HSQC 1H-15N spectra were acquired using the uniformly labelled 15 N DENVC. The experiments were performed in the presence and absence of the ligands. For the first titration point, a 200 µM DENVC solution was used. For the next four titration points, it was used a molar excess of the ligands: 1, 3, 6, and 9 relatives to the protein. Samples were prepared in PBS buffer (55 mM NaH2PO4/Na2HPO4, 200 mM NaCl, 5 mM EDTA, pH 6.0) with 10% (v/v) D2O (Cambridge Isotope Laboratories), and 5% (v/v) DMSO-d6 (Cambridge Isotope Laboratories). Spectra were acquired on a Bruker Avance III 18.6 Tesla (800 MHz for hydrogen) spectrometer with a 5 mm probe at 308 K using the hsqcetf3gpsi pulse sequence, water suppression by water flip back and gradientsCitation41–44, 8 scans, 16 dummy scans, 1024 × 256 dot spectral window. The experiments were processed using the CcpNmr Analysis softwareCitation45 and were assigned according to NMR data (PDB ID code 1R6R)Citation46 obtained from the Biological Magnetic Resonance Data Bank (BMRB)Citation47.
2.5. Fluorescence analysis
Fluorescence measurements were taken in a Varian Cary Eclipse Fluorescence Spectrophotometer at 308 K. One scan was performed on a 10 µM DENVC sample in PBS buffer using an excitation wavelength of 280 nm and emission detected from 300 to 420 nm with slits set to 5 nm (excitation) and 10 nm (emission). Stock solutions of compounds 1 and 4 were prepared in H2O and for compounds 12, 15, and 16, due to their low solubility in water, in DMSO-d6. The protein sample was titrated by adding aliquots of the ligand stock solution in different concentrations, according to each protocol, as described in .
Figure 2. Effect of intrinsic fluorescence on the relative intensity of the signal of DENVC (10 μM) obtained by titration of compounds with excitation at 280 nm and scanning emission between 305 and 425 nm in the absence and presence of compound: (A) 1, (B) 4, (C) 12, (D) 15, and (E) 16. The insets show the change in the maximum emission wavelength ( open circle) as a function of the selected compound concentration. The black line in the insets denotes the better fitting to the experimental data.

A total of 10 titration points was acquired for each inhibitor, compound 1: apo, 3.33, 6.67, 10, 20, 30, 40, 60, 80, and 100 µM; compound 4: apo, 10, 20, 30, 60, 90, 120, 150, 180, and 210 µM; compound 12: apo, 40, 80, 120, 160, 200, 280, 360, 520, and 680 µM; compound 15: apo, 10, 30, 50, 70, 90, 110, 130, 150, and 190 µM; and compound 16: apo, 3, 9, 15, 21, 27, 36, 45, 54, and 63 µM.
Before each measurement, the samples were placed to reach thermal equilibrium for 10 min. Each experiment was performed in triplicate and the solvent (blank) with each compound was subtracted from the mean of three means of three replicate samples. The values of maximum emission wavelength () were used to build the binding isotherms for the DENVC/selected compound interactions. The program Origin 2021 was employed to fit the following equation to the experimental dataCitation48:
(2)
(2)
where
and
are the initial and saturation maximum emission wavelength, respectively,
is the dissociation constant,
is the total concentration of the selected compound, and
is the total concentration of the DENVC. For compound 12, it was added a linear contribution to EquationEquation (2)
(2)
(2) to perform the fitting.
2.6. Pharmacokinetic and toxicological properties
In silico pharmacokinetic and toxicological properties (molecular weight (g/mol), relative polar surface area (PSA) (Å), lipophilicity (c-LogP), water solubility (c-LogS), donor sites (nOHNH), hydrogen bonding acceptors (nOH), number of rotatable bonds, and toxicity), using DataWarrior softwareCitation49, were calculated for the five compounds that showed interaction by STD-NMR experiment.
2.7. Molecular docking
The DENVC structure used for the computational simulations was downloaded from Protein Data Bank (PDB) under access code 1R6RCitation46. The molecular structures of the compounds were obtained by structural optimisation calculations from the semi-empirical PM6 method using the Gaussian 09 programCitation50. DENVC and compounds were prepared using AutoDockTools programCitation51 for molecular docking simulations, merging non-polar hydrogen atoms, and adding atom types. The rigid root of the compounds was generated automatically, setting all possible rotatable bonds defined as active by torsions. The molecular docking calculations were performed in triplicate by using AutoDock VinaCitation52, applying a total of 8 exhaustiveness. The coordinates of the centre of the conformational search box were defined to enrol the whole hydrophobic cleft (α1/α1’ and α2/α2’) in DENVC since 1H-15N HSQC experiments indicate that all compounds bind to this protein region. The box dimensions were 40 × 30 × 20 Å on the three coordinate axes. Following docking calculations, the lowest energy structural models of DENVC/compound complexes were analysed by PLIP webserverCitation53 for characterising the protein/ligand non-covalent interactions, such as hydrophobic contacts, hydrogen bond, π-cation interaction, and halogen bonds. Structural conformation of the constructed models was displayed using PyMOLCitation54.
2.8. Cytotoxicity assay
Stock solutions (200 mM) of the lyophilised form of the compounds 1, 4, 12, 15, and 16 were prepared in dimethyl sulfoxide (DMSO) and then stored at −20 °C. For the experimental procedures, the stock solutions were diluted in PBS keeping a final concentration of 5% DMSO. Each solution was used to treat the cells at the established concentrations. Human hepatocarcinoma cell line (Huh7) and human epithelial lung cells (A549) were cultured in Dulbecco’s modified Eagle’s medium (DMEM), supplemented with 10% foetal bovine serum (FBS) (Invitrogen, USA), 100 U/mL penicillin, 100 g/mL streptomycin, 0.22% sodium bicarbonate, and 0.2% HEPES (pH 7.4), in a CO2 humid incubation chamber, at 37 °C. The cytotoxicity of compounds was evaluated in vitro using a 3–(4,5-dimethylthiazol-2-yl)-2,5-diphenyl tetrazolium bromide (MTT) (USB, Ohio, USA) assay. Monolayers with 6 × 104 cells per well of the Huh7 and A549 cell lines were prepared in, 48-well, cell culture plates. The cells were treated with increasing concentrations of compounds and the times of 24 and 48 h after treatment were analysed. After this time, the cells were incubated for 40 min with 0.5 mg/mL of MTT at 37 °C. Next, the solution was removed and precipitated formazan was diluted in isopropyl alcohol with 40 mM HCl. Absorbance was measured for each well at 570 nm (compounds-treated and control) and 650 nm (background). The percentage of cell viability was calculated as follows: 100% x (absorbance of treated cells) – (background)/(absorbance of untreated cells) – (background).
Statistical analyses were performed using GraphPad Prism 8.0.2 (GraphPad Software, Inc.). Results are presented as means ± standard errors (SEM) and were compared by two-way analysis of variance (ANOVA) and Dunnett's multiple comparisons test p values of ≤0.05 were considered significant.
3. Results and discussion
3.1. Synthesis and characterisation of the organic compounds
Eighteen compounds were used in this study, 8 aromatic guanylhydrazones and 10 aromatic oximes (). We chose aromatic and amphipathic compounds to target the interaction with the hydrophobic cleft (α1/α1’ and α2/α2’) in DENVC. We expect the aromatic compounds to interact with the nonpolar exposed surface and with the aromatic backbone at the hydrophobic cleft.
Compounds 1–8 were synthesised in the one-step reaction between the correspondent aldehydes and aminoguanidine hydrochloride, using ethanol (95%) as the solvent, HCl as the catalyst, and heating under reflux for 3 − 23 h, generating monocationic compounds obtained in 70–97% yields. The mechanism for those reactions is based on the nucleophilic attack of the –NH2 group of aminoguanidine to the –C = O group of the aldehyde, followed by the loss of molecule water and the formation of a double bond, leading to the corresponding guanylhydrazone (Supplementary Information, Scheme S1).
The oximes 9–18 were prepared by the reaction of hydroxylamine hydrochloride with the corresponding aldehydes, using ethanol (95%) and distilled water as solventsCitation26,Citation55,Citation56. After stirring, the product was vacuum filtered and washed with cold distilled water, leading to compounds obtained in 50–98% yields. The time of stirring (1–36 h) and the yield for each compound is described in Supplementary Material with the spectral assignment data. The mechanism for forming oximes is similar to that of guanylhydrazones, based on the nucleophilic attack of the –NH2 group of hydroxylamine to the –C=O group of the aldehyde, followed by loss of water and formation of the double bond (Supplementary Information, Scheme S2). Compounds 2, 4, 5–11, 13, 14, and 18 are new unpublished agents. Although compounds 1, 3, 12, 15, 16, and 17 have already been reported in the literature, they have never been tested as DENVC inhibitors. All compounds were characterised by infra-red spectroscopy (IR) and NMR (Supplementary Information, Figures S1–S54).
3.2. Ligand-protein interaction studies
To identify the compounds able to bind DENVC, we performed NMR STD experiments. We made pools of 4–5 compounds. The pools were divided according to the observed proton NMR chemical shifts, avoiding overlaps of the signals of the different compounds (Figures S55–S58). The STD spectra for pools 1–4 (Figures S59–S62) showed that compounds 1, 4, 11, 12, 15, and 16 binds to DENVC. For these selected ligands, we ran STD experiments for each compound. Compound 11 showed an STD weak signal near the noise (Figure S61) and thus it was not selected for further analysis. The analysis of the compound 1 STD spectrum revealed that all hydrogens are involved in the binding site of DENVC (). Similar results were observed for compounds 4, 12, 15, and 16 (Figures S63–S66).
Figure 3. STD spectrum of 1 mM of compound 1 and 10 μM of DENVC in PBS buffer H2O/D2O (90%/10%) at different frequencies of irradiation (746, 124.7, and 2688 Hz). At the bottom, the reference 1H-NMR spectrum of the compound.

To investigate the intermolecular interaction between DENVC and the selected compounds, we also analysed relaxation parametersCitation57. We measured proton relaxation times T1 and T2 for the selected compounds in the presence and absence of DENVC (). Changes in T1 and T2 values for compounds 1, 4, 12, 15, and 16 after the addition of the protein corroborate the STD experiments, showing that all compounds interact with DENVC. As expected for binding, T2 of all compounds increased in the presence of the protein, with the exception of hydrogen 1 of compounds 1 and 16. This change could be a result of the presence of conformational exchange at this site in the free ligand that is reduced upon binding. Interestingly, it occurred in the same site for both compounds. The aromatic hydrogens of all compounds presented major T2 changes, suggesting the aromaticity of these compounds is important for the interaction.
Table 1. T1 and T2 values for 1 mM of compounds 1, 4, 12, 15, and 16 in the absence and the presence of 10 uM of DENVC.
We also measured the translational diffusion coefficient in the presence and absence of DENVC (). We observed a decrease in the diffusion coefficient for all compounds in the presence of the protein. Accordingly, such a decrease is expected when a small molecule binds to a high molecular weight biomolecule like a proteinCitation58.
Table 2. Diffusion coefficient (D) for 1 mM of compounds 1, 4, 12, 15, and 16 in the presence and absence of 10 μM of DENVC.
To identify the DENVC residues involved in the binding, we mapped the changes in the signal intensity and chemical shift perturbation (CSP) for all residues by 1H-15N-HSQC experiments in the presence and absence of the selected compounds (1, 4, 12, 15, and 16) ( and S67). The residues showing the highest changes according to the average plus one standard deviation (M + SD) and the average plus two standard deviations (M + 2SD) (Table S1) were selected and represented along with the three-dimensional structure of the DENVC (). When we mapped the CSP values and changes in intensity in the protein structure, we observed perturbations at α1, α2, and α3 helices, indicating that the compounds interact in the hydrophobic cleft, the targeted region.
Figure 4. DENVC (200 µM) chemical shift perturbations (CSP) mapped by HSQC 1H-15N in the absence and presence of 1.8 mM of (A) compound 1, (B) 4, (C) 12, (D) 15, and (E) 16. The solid line denotes the average CSP value (M) for all residues plus the standard deviation (SD), and the dashed line indicates the average plus twice standard deviation (2SD).

Figure 5. Analysis of the molecular docking results for the structural model of the complex of DENVC with compounds 1 (A), 4 (B), 12 (C), 15 (D), and 16 (E). All compounds are in the symmetric pockets of the hydrophobic cleft in DENVC. The protein is shown as a cartoon model with the monomers coloured in grey for chain A and golden yellow for chain B. The secondary structures of α-helix of the chain B are indicated as α1, α2, α3, and α4. The compounds are denoted as a stick model with carbon, oxygen, nitrogen, sulphur, chlorine, and bromine coloured in green, red, blue, yellow, magenta, and dark red, respectively. The residues with changes in chemical shift (CSP) and intensity higher than M + SD and M + 2SD are denoted as cyan and blue lines/spheres, respectively.

The residues identified by 1H-15N-HSQC experiments were: for compound 1, V23, L29, and R32 in α1, Q39, G42, and L44 in the α1–α2 loop, L50 and L54 in α2, R68 and W69 in α3, I72 in the α3–α4 loop, L95 in α4, and R99 in the C-terminal region; for compound 4, V23, Q28, and L29 in α1, L38, Q39, and L44 in the α1–α2 loop, L50, L54, and F56 in α2, R68 and W69 in α3, and I72 in the α3–α4 loop; for compound 12, E19 in the N-terminal region, V23, Q27, L29, and R32 in α1, M37, Q39, G42, and L44 in the α1–α2 loop, L50, A52, and L54 in α2, T62 in the α2–α3 loop, and R68 and W69 in α3; for compound 15, V23, Q27, Q28, L29, and R32 in α1, L38 and L44 in the loop α1–α2, L50 and V51 in α2, T62 in the α2–α3 loop, R68 and W69 in α3, L95 in α4, and R99 in the C-terminal region; and for compound 16, S24, V26, and Q27 in α1, Q39 and F47 in the α1–α2 loop, L50, A52, and L54 in α2, R68 and W69 in α3, F84 and N96 in α4, and R97, R98, R99, and R100 in the C-terminal region. Residues L50 in α2, the core of the hydrophobic cleft, and R68 and W69 in α3 stand out since they are perturbed in all compounds. Compound 16 promoted singular changes in CSP values of the C-terminal residues. It is worth mentioning that W69, with the side chain in the α2–α3 interface, can be used as a fluorescent probe for investigating the DENVC/selected compound interaction.
We used the intrinsic tryptophan fluorescence of DENVC to measure the binding affinity of each of the selected compounds. All compounds led to fluorescence quenching as a consequence of protein binding. For compounds 1, 4, 12, and 15, the binding resulted in a redshift of the tryptophan fluorescence spectra, indicating the exposure of W69 to a more polar environment. Conversely, for compound 16, the binding led to a blue shift in the spectrum, meaning that, in this case, W69 is buried in a more hydrophobic environmentCitation59 (). Interestingly, compound 16 showed significant CSP values in the C-terminal region (). The results suggest a specific interaction of these compounds with DENVC, inducing a conformational change in the protein. The dissociation constants () were calculated using the change in the maximum emission wavelength (
) as a function of the ligand concentration (inset in ). Compounds 1, 4, and 12 presented a hyperbolic binding isotherm, displaying
of 18 ± 4, 20 ± 7, and 23 ± 8 µM, respectively. Compounds 15 and 16 displayed an almost linear isotherm in the measured concentration ranges, probably because they presented affinities in the millimolar range.
The 1H-15N HSQC experiments indicated that all selected compounds bind to the hydrophobic cleft in DENVC. These experimental results drove the docking calculation of the compounds in the protein binding site. shows the structural models of the DENVC/compounds complexes determined from the docking calculations. The docking poses of the compounds correspond to the lowest energy conformers for the triplicate calculations. It is possible to observe in that the compounds interacted in the two symmetric pockets of the hydrophobic cleft in DENVC. These symmetric pockets are formed by the α1–α2 loop and α3 on both chains of the dimer. An analysis of the non-covalent interactions involved in the structural models revealed the occurrence of hydrophobic contacts and hydrogen bonds in all DENVC/compound complexes, while π-cation interactions appeared exclusively to the compounds 1 and 4 and halogen bonds to the compound 15 (Table S2–S6). In at least two of the three replicas, compound 1 presented hydrophobic interactions with P43 and L46 and formed hydrogen bonds with L29, R41, and L44 (Table S2). The same hydrophobic contacts were observed for compound 4 (Table S3), but the hydrogen bond was only recurrent with L29. Compound 12 showed the highest number of hydrophobic interactions, being formed with L29, R32, F33, L44, L46, and F47, while only one hydrogen bond was formed with R41 (Table S4). Compounds 15 and 16 established hydrogen bonds with R41 and L44 in two of three docking runs (Tables S5 and S6). The hydrophobic contacts were remarkably similar for these compounds, being formed with L29, R32, and L46, except F47 that was observed only for compound 16. It is possible to see that R41 and L46 are important residues for the binding of all compounds in the symmetric pockets of DENVC hydrophobic cleft, the former involved in hydrogen bonds, and the last in hydrophobic interaction. It is worth mentioning that R41 also is the main residue responsible for the formation of π-cation interactions with compounds 1 and 4 (Tables S2 and S3). Interestingly, the halogen bond is significant and a recurring interaction in triplicate docking calculations, which is established between the R68 and bromine atom of compound 15 (Table S5).
Figure 6. Cytotoxicity of the studied compounds in Huh7 and A549 cells by the MTT assay. The schematic figures of each panel illustrate the cell viability after treatment with increasing concentrations of the respective compounds (1, 4, 12, 15, and 16) in Huh7 (A, C, E, G, and I) and A549 (B, D, F, H, and J) cells, after 24 (black column) and 48 h (grey column). The results are presented as a percentage of the cell viability of the treated cells in relation to the untreated cell maintained in DMEM medium with 0.5% DMSO. Data are represented as mean ± SEM of the results of at least three independent experiments. For statistical analysis, each treated condition was compared with the untreated control at the respective times. Asterisks indicate significant differences between untreated cells and treated cells as follows: *P ≤ 0.05; **P ≤ 0.01; ****P ≤ 0.0001.

3.3. Pharmacokinetic and toxicological properties
The calculated pharmacokinetic parameters () show that the 5 selected compounds are candidates as fragment leads. The solubility (cLogS) for all compounds was found in an acceptable range (<4)Citation60. The cLogP parameter, which is directly related to a more favourable drug-likeness profile when ≤5Citation61,Citation62, shows that all compounds meet this rule. They all have molecular weights ≤500 g/mol, which means that transportation and absorption are easier than heavy moleculesCitation62. Regarding the polar surface area criterium (PSA ≤140 Å2 for oral bioavailabilityCitation62 or 90 Å2 for the cellular permeabilityCitation61, all compounds showed acceptable results, with exception of compounds 1, 4, and 16 for cellular permeability. The results for donor sites (nOHNH) and hydrogen bonding acceptors (nOH), below 10 and 5, respectively, show that all compounds meet the rule for oral bioavailabilityCitation62 and for crossing the blood-brain barrier (central nervous system, CNS)Citation61. They all have rotatable bond ≤5, which is related to the binding potency and penetration into the membraneCitation62. Thus, the results showed that none of the studied compounds presented more than three violations to Lipinski’s rule, suggesting they are suitable candidates for oral drugs.
Table 3. In silico pharmacokinetic parameters and toxicological properties of compounds.
The toxicity risk calculations search for substructures within the chemical structure being indicative of specific toxicity according to a reference database (Registry of Toxic Effects of Chemical Substances database – RTECS), which covers compounds of different toxicity classesCitation49,Citation63. The absence of risky fragments suggests a low risk concerning the toxicity class under investigationCitation63. For these studies, the toxicity classes related to mutagenic, tumorigenic, or irritant effects or being associated with reproductive effects are considered. The results for all compounds showed they do not present any toxicological risks for those classes. It is important to emphasise that these results do not eliminate the need for traditional toxicological tests.
We evaluated the cytotoxicity of the compounds on Huh7 and A549 cells. Both cell lines were chosen because they are widely used in studies of DENV infection and replication. The MTT assay was performed after 24 and 48 h of treatment with increasing concentrations of each compound (, AJ). Cell lines maintained in culture medium with 0.5% DMSO were used as controls since this is the final DMSO concentration in the medium after the addition of the compounds. The viability results for cell treatment with each concentration of the compounds are expressed as a percentage related to cells treated with only DMSO, which did not significantly affect the cell viability. In general, the compounds were more toxic to Huh7 cells than to A549 cells. Compound 1 was very toxic to HuH7 cells even at low concentrations, leading to about 50% loss of viability at 30 µM after 48 h (). On the other hand, for A549, the compound was toxic only at much higher concentrations, such as 400 µM (). The most cytotoxic compound for both cells studied was compound 4, with concentrations above 20 µM being highly toxic (). On the other hand, compounds 12, 15, and 16 showed much more promising results (). Compounds 15 were toxic only at concentrations above 100 or 400 µM to HuH7 or A549, respectively (), while compounds 12 and 16 were toxic to HuH7 only at concentrations above 400 µM (), with even better results to A549 (). Compound 16 was non-toxic to A549 cells () at all the concentrations tested and showed low cytotoxicity up to 100 µM in Huh7 cells, with cell viability of 98% and 80% after 24 and 48 h treatment, respectively (). Therefore, among the tested compounds, compound 16 may be seen as the best candidate to explore a possible antiviral activity against DENV in future trials.
4. Conclusions
Here we presented a targeted screen of small aromatic compounds to the hydrophobic cleft (α1-α1' and α2-α2') of DENVC. The strategy was successful, enabling the description of new fragment leads that binds to the hydrophobic cleft, and this new series of compounds may be used as promising leads for dengue therapy. The advantages of these compounds include physical properties compatible with desirable pharmacokinetic parameters, such as low toxicity, simple synthetic procedure, and evidence of binding at a micromolar concentration to DENVC. Remarkably, STD-selected compounds elicited conformational changes upon DENVC binding. For compounds 1, 4, 12, and 15, the tryptophan residue (W69) is more solvent-exposed, possibly making the hydrophobic core looser, and for compound 16, W69 is less solvent-exposed, hidden in a more rigid hydrophobic core. NMR-derived docking calculations suggest that the selected compounds are located in asymmetric binding sites formed by α1, α2, and α3 in the hydrophobic cleft, where W69 is at the centre. These studies should allow the development of antiviral analogs targeting the hydrophobic cleft of the DENVC and possibly blocking its interaction with lipid droplets.
Supplemental Material
Download PDF (4.2 MB)Acknowledgements
The author IPC gratefully acknowledges the financial support by a postdoctoral fellowship from FAPERJ and the PROPe UNESP. The author LOO was a fellow of CAPES.
Disclosure statement
No potential conflict of interest was reported by the authors.
Additional information
Funding
References
- Guzman MG, Gubler DJ, Izquierdo A, et al. Dengue infection. Nat Rev Dis Prim 2016;2:1–25.
- Diamond MS, Pierson TC. Molecular insight into dengue virus pathogenesis and its implications for disease control. Cell. Cell Press 2015;162:488–92.
- Jones CT, Ma L, Burgner JW, et al. Flavivirus capsid is a dimeric alpha-helical protein. J Virol 2003;77:7143–9.
- Mukhopadhyay S, Kuhn RJ, Rossmann MG. A structural perspective of the flavivirus life cycle. Nat Rev Microbiol 2005;3:13–22.
- Neves-Martins TC, Mebus-Antunes NC, Caruso IP, et al. Unique structural features of flaviviruses' capsid proteins: new insights on structure-function relationship. Curr Opin Virol 2021;47:106–12.
- Morando MA, Barbosa GM, Cruz-Oliveira C, et al. Dynamics of zika virus capsid protein in solution: the properties and exposure of the hydrophobic cleft are controlled by the α-Helix 1 sequence. Biochemistry 2019;58:2488–98.
- Samsa MM, Mondotte JA, Iglesias NG, et al. Dengue virus capsid protein usurps lipid droplets for viral particle formation. Diamond MS, editor. PLoS Pathog 2009;5:e1000632.
- Martins IC, Gomes-Neto F, Faustino AF, et al. The disordered N-terminal region of dengue virus capsid protein contains a lipid-droplet-binding motif. Biochem J 2012;444:405–15.
- Faustino AF, Guerra GM, Huber RG, et al. Understanding dengue virus capsid protein disordered N-terminus and pep14-23-based inhibition. ACS Chem Biol 2015;10:517–26.
- Poh MK, Yip A, Zhang S, et al. A small molecule fusion inhibitor of dengue virus. Antiviral Res 2009;84:260–6.
- Steuer C, Gege C, Fischl W, et al. Synthesis and biological evaluation of α-ketoamides as inhibitors of the Dengue virus protease with antiviral activity in cell-culture. Bioorg Med Chem 2011;19:4067–74.
- Xie X, Wang Q-Y, Xu HY, et al. Inhibition of dengue virus by targeting viral NS4B protein. J Virol 2011;85:11183–95.
- Lim SP, Sonntag LS, Noble C, et al. Small molecule inhibitors that selectively block dengue virus methyltransferase. J Biol Chem 2011;286:6233–40.
- Chen Y-L, Yin Z, Duraiswamy J, et al. Inhibition of dengue virus RNA synthesis by an adenosine nucleoside. Antimicrob Agents Chemother 2010;54:2932–9.
- Niyomrattanakit P, Chen YL, Dong H, et al. Inhibition of dengue virus polymerase by blocking of the RNA tunnel. J Virol 2010;84:5678–86.
- Wang Q-Y, Kondreddi RR, Xie X, et al. A translation inhibitor that suppresses dengue virus in vitro and in vivo. Antimicrob Agents Chemother 2011;55:4072–80.
- Scaturro P, Trist IML, Paul D, et al. Characterization of the mode of action of a potent dengue virus capsid inhibitor. J Virol 2014;88:11540–55.
- Byrd CM, Dai D, Grosenbach DW, et al. A novel inhibitor of dengue virus replication that targets the capsid protein. Antimicrob Agents Chemother 2013;57:15–25.
- Xia H, Xie X, Zou J, et al. A cocrystal structure of dengue capsid protein in complex of inhibitor. Proc Natl Acad Sci USA 2020;117:17992–8001.
- Andreani A, Burnelli S, Granaiola M, et al. New antitumor Imidazo[2,1-b]Thiazole guanylhydrazones and analogues. J Med Chem 2008;51:809–16.
- Banday AH, Akram SMM, Shameem SA. Benzylidine pregnenolones and their oximes as potential anticancer agents: synthesis and biological evaluation. Steroids 2014;84:64–9.
- Harini ST, Kumar HV, Rangaswamy J, et al. Synthesis, antioxidant and antimicrobial activity of novel vanillin derived piperidin-4-one oxime esters: preponderant role of the phenyl ester substituents on the piperidin-4-one oxime core. Bioorg Med Chem Lett 2012;22:7588–92.
- Singh B, Maheshwari A, Dak G, et al. Studies of antimicrobial activities of some 4-thiazolidinone fused pyrimidines, [1,5]-benzodiazepines and their oxygen substituted hydroxylamine derivatives. Indian J Pharm Sci 2010;72:607–12.
- Lazić J, Ajdačić V, Vojnovic S, et al. Bis-guanylhydrazones as efficient anti-Candida compounds through DNA interaction. Appl Microbiol Biotechnol 2018;102:1889–901.
- Rossello A, Bertini S, Lapucci A, et al. Synthesis, antifungal activity, and molecular modeling studies of new inverted oxime ethers of oxiconazole. J Med Chem 2002;45:4903–12.
- Zhang L, Jiang CS, Gao LX, et al. Design, synthesis and in vitro activity of phidianidine B derivatives as novel PTP1B inhibitors with specific selectivity. Bioorg Med Chem Lett 2016;26:778–81.
- Shamroukh AH, Zaki MEA, Morsy EMH, et al. Synthesis of pyrazolo[4',3':5,6]pyrano[2,3-d]pyrimidine derivatives for antiviral evaluation. Arch Pharm 2007;340:236–43.
- Degardin M, Wein S, Duckert J-F, et al. Development of the first oral bioprecursors of bis-alkylguanidine antimalarial drugs. ChemMedChem 2014;9:300–4.
- Papanastasiou I, Tsotinis A, Zoidis G, et al. Design and synthesis of Trypanosoma brucei active 1-alkyloxy and 1-benzyloxyadamantano 2-guanylhydrazones. ChemMedChem 2009;4:1059–62.
- Mohassab AM, Hassan HA, Abdelhamid D, et al. Novel quinoline incorporating 1,2,4-triazole/oxime hybrids: synthesis, molecular docking, anti-inflammatory, COX inhibition, ulceroginicity and histopathological investigations. Bioorg Chem 2017;75:242–59.
- Ottanà R, Maccari R, Barreca ML, et al. 5-Arylidene-2-imino-4-thiazolidinones: design and synthesis of novel anti-inflammatory agents. Bioorg Med Chem 2005;13:4243–52.
- Petronilho E da C, Rennó M do N, Castro NG, et al. Design, synthesis, and evaluation of guanylhydrazones as potential inhibitors or reactivators of acetylcholinesterase. J Enzyme Inhib Med Chem 2016;31:1069–78.
- Prasad RN, McKay AF. Acylation of guanidines and guanylhydrazones. Can J Chem 1967;45:2247–52.
- Amidi S, Esfahanizadeh M, Tabib K, et al. Rational design and synthesis of 1-(arylideneamino)-4-aryl-1H-imidazole-2-amine derivatives as antiplatelet agents. ChemMedChem 2017;12:962–71.
- Bonomi P, Servant A, Resmini M. Modulation of imprinting efficiency in nanogels with catalytic activity in the Kemp elimination. J Mol Recognit 2012;25:352–60.
- Jiménez-Juárez R, Cruz-Chávez W, de Jesús-Ramírez N, et al. Synthesis and antimycobacterial activity of 2,5-disubstituted and 1,2,5-trisubstituted benzimidazoles. Front Chem 2020;8: 433.
- Irfan I, Sawangjaroen N, Bhat AR, et al. New dioxazole derivatives: synthesis and effects on the growth of Entamoeba histolytica and Giardia intestinalis. Eur J Med Chem 2010;45:1648–53.
- Trefzger OS, Barbosa NV, Scapolatempo RL, et al. Design, synthesis, antileishmanial, and antifungal biological evaluation of novel 3,5‐disubstituted isoxazole compounds based on 5‐nitrofuran scaffolds. Arch Pharm 2020;353:1900241.
- Carvalho FAA, Carneiro FAA, Martins ICC, et al. Dengue virus capsid protein binding to hepatic lipid droplets (LD) is potassium ion dependent and is mediated by LD surface proteins. J Virol 2012;86:2096–108.
- Hwang TL, Shaka AJ. Water suppression that works. Excitation sculpting using arbitrary wave-forms and pulsed-field gradients. J Magn Reson Ser A 1995;112:275–9.
- Grzesiek S, Bax A. The importance of not saturating water in protein NMR. Application to sensitivity enhancement and NOE measurements. J Am Chem Soc 1993;115:12593–4.
- Kay L, Keifer P, Saarinen T. Pure absorption gradient enhanced heteronuclear single quantum correlation spectroscopy with improved sensitivity. J Am Chem Soc 1992;114:10663–5.
- Palmer AG, Cavanagh J, Wright PE, et al. Sensitivity improvement in proton-detected two-dimensional heteronuclear correlation NMR spectroscopy. J Magn Reson 1991;93:151–70.
- Schleucher J, Schwendinger M, Sattler M, et al. A general enhancement scheme in heteronuclear multidimensional NMR employing pulsed field gradients. J Biomol NMR 1994;4:301–6.
- Vranken WF, Boucher W, Stevens TJ, et al. The CCPN data model for NMR spectroscopy: development of a software pipeline. Proteins Struct Funct Genet 2005;59:687–96.
- Ma L, Jones CT, Groesch TD, et al. Solution structure of dengue virus capsid protein reveals another fold. Proc Natl Acad Sci USA 2004;101:3414–9.
- Markley JL, Ulrich AEEL, Berman AEHM, et al. BioMagResBank (BMRB) as a partner in the Worldwide Protein Data Bank (wwPDB): new policies affecting biomolecular NMR depositions. J Biomol NMR 2008;40:153–5.
- Caruso IP, Guimarães GC, Machado VB, et al. Biophysical and dynamic characterization of fine-tuned binding of the human respiratory syncytial virus M2-1 core domain to long RNAs. J Virol 2020;94(23):e01505-20.
- Sander T, Freyss J, von Korff M, et al. DataWarrior: an open-source program for chemistry aware data visualization and analysis. J Chem Inf Model 2015;55:460–73.
- Frisch MJ, Trucks GW, Schlegel HB, et al. Gaussian 09. Wallingford, CT: Gaussian, Inc.; 2016.
- Sanner MF. Python: a programming language for software integration and development. J Mol Graph Model 1999;17:57–61.
- Trott O, Olson AJ. AutoDock Vina: improving the speed and accuracy of docking with a new scoring function, efficient optimization, and multithreading. J Comput Chem 2009;31:NA.
- Salentin S, Schreiber S, Haupt VJ, et al. PLIP: fully automated protein-ligand interaction profiler. Nucleic Acids Res 2015;43:W443–W447.
- Delano WL, The PyMOL molecular graphics system. San Carlos (CA): DeLano Scientific; 2002.
- Hunan Huateng Pharmaceutical Co. L. A kind of preparation method of bridged piperazine derivatives. Republic of China Patent. CN106810513A, filed October 1, 2017, and issued September 6, 2017.
- Dengke L, Xiaoli F, Ying L, et al. Preparation of 6,7-dihydro-4H-thieno[3,2-c]pyridine derivatives for treatment of depression. Republic of China Patent. CN 102503953, filed October 20, 2011, and issued June 20, 2012
- Figueroa-Villar J, Tinoco L. Spin-lattice relaxation time in drug discovery and design. Curr Top Med Chem 2009;9:811–823.
- Figueiredo IM, Marsaioli AJ. Mapeamento das interações proteína-ligante através de técnicas de RMN de 1H utilizando detecção do ligante. Quim Nova 2007;30:1597–1605.
- Weber G. Fluorescence-polarization spectrum and electronic-energy transfer in tyrosine, tryptophan and related compounds. Biochem J 1960;75:335–345.
- Meanwell NA. Improving drug candidates by design: a focus on physicochemical properties as a means of improving compound disposition and safety. Chem Res Toxicol 2011;24:1420–1456.
- Pajouhesh H, Lenz GR. Medicinal chemical properties of successful central nervous system drugs. NeuroRX 2005;2:541–553.
- Lipinski CA. Lead- and drug-like compounds: the rule-of-five revolution. Drug Discov Today Technol 2004;1:337–341.
- von Korff M, Sander T. Toxicity-indicating structural patterns. J Chem Inf Model 2006;46:536–544.