Abstract
Natural products and analogues are a source of antibacterial drug discovery. Considering drug resistance levels emerging for antibiotics, identification of bacterial metalloenzymes and the synthesis of selective inhibitors are interesting for antibacterial agent development. Peptide nucleic acids are attractive antisense and antigene agents representing a novel strategy to target pathogens due to their unique mechanism of action. Antisense inhibition and development of antisense peptide nucleic acids is a new approach to antibacterial agents. Due to the increased resistance of biofilms to antibiotics, alternative therapeutic options are necessary. To develop antimicrobial strategies, optimised in vitro and in vivo models are needed. In vivo models to study biofilm-related respiratory infections, device-related infections: ventilator-associated pneumonia, tissue-related infections: chronic infection models based on alginate or agar beads, methods to battle biofilm-related infections are discussed. Drug delivery in case of antibacterials often is a serious issue therefore this review includes overview of drug delivery nanosystems.
Introduction
Widespread resistance to antibiotics is now a global threat to society. Despite this the number of new antibacterials that have been brought to the market has declined considerably in recent decades. Additionally, many of the antibiotics that are in clinical development belong to existing families of compounds and their antibacterial activity and thus usefulness is likely to be decreased by rapid development of resistance in clinical strains. Therefore there is an urgent need for the development of new classes of antibacterials, preferably with new molecular targets and/or mechanism of action.
As said WHO’s Assistant Director-General for Health Security Dr. Keiji Fukuda “Without urgent, coordinated action by many stakeholders, the world is headed for a post-antibiotic era, in which common infections and minor injuries which have been treatable for decades can once again kill”. In other words, still in nearest 10–15 years there is a high risk that going to a hospital with a minor injury to die from an antibiotic resistant form of hospital infection.
Antimicrobial drug-resistant bacteria are responsible for about 33 000 human deaths annually in the European Union only. It is important to highlight that drug-resistance is growing frighteningly fast.
In this view we summarise here selected strategies for dealing with pathogenic bacteria. Since drug delivery especially in case of antibacterials often is a serious issue therefore this review also includes overview on drug delivery nanosystems.
Application of natural products and their synthetic analogues in antibacterial drug discovery
The majority of clinically used antibiotics are natural products or derivatives thereof. The history began with the discovery of penicillin in 1928. The pool of natural products constitute a very rich source of chemical diversity, and it remains to have an untapped potential for discovery of novel antibacterial agents. According to a recent review by Newman & CraggCitation1, ca. 75% of all approved drugs in the timeframe 1981–2019 originated from or were inspired by natural products. Thus, the importance of natural products as leads for novel antibacterial agents remain evident: of FDA-approved antibacterials, 69% originate from natural products and 97% of these are isolated or derived from microbesCitation2. Regardless of the success in discovery of new biologically active compounds from natural products, their use in pharmaceutical research has declined during the past decades. The main reasons for this trend stem from the lengthy process required for natural product discovery, and from the incompatibility of natural product libraries with most high-throughput screening operations. Furthermore, in many cases the originally isolated bioactive natural product is not necessarily the lead compound taken further in drug development. For example, it may not be optimal in terms of physico-chemical properties or not be available in required amounts from the original source. Alternatively, natural products may be utilised in various ways as inspiration to create biologically relevant compounds and compound collections, for example by using natural product-derived or -inspired scaffolds or fragments as starting points for synthetic librariesCitation3.
Three recently developed antibiotics (i.e. eravacycline, vaborbactam and doripenem) serve as examples of utilising natural products as sources or inspiration of new antibiotics to combat antimicrobial resistance. In addition, a brief account of the discovery of anti-biofilm agents based on coniferous diterpenes (i.e. abietic acid and dehydroabietic acid) is presented.
A large number of antibacterial tetracyclines, produced as secondary metabolites by the genus Streptomyces, have been isolated and characterised since the discovery of chlortetracycline 80 years agoCitation4. The widely used tetracycline class of antibiotics has led to a reduced efficacy against pathogenic bacteria due to development of drug resistanceCitation5. Eravacycline (Scheme 1) is a synthetic, tetracycline-class antibiotic that has been developed for the treatment of infections caused by multidrug-resistant bacteria, e.g. methicillin-resistant Staphylococcus aureus (MRSA) and carbapenem-resistant Enterobacteriaceae speciesCitation6. Eravacycline was approved by FDA in 2018. The four-step synthesis route of this 7-fluorotetracycline involves a tandem Michael addition and Dieckmann cyclisation as key steps (Scheme 1). This facile synthesis route also enables preparation of tetracycline libraries for further structure-activity studies.
Scheme 1. Synthesis of eravacycline. (i) LDA, TEA•HCl, THF -70 °C; LiHMDS, -70 °C to -10 °C, 94%; 48% HF, MeCN, rt; (ii) H2, 5% Pd-C, HCl, MeOH-H2O, rt, 89%; (iii) 2-(pyrrolidin-1-yl)acetyl chloride hydrochloride, MeCN-H2O, 10 °C, 89%; (iv) HCl, MeOH, EtOH; EtOAc, quant.
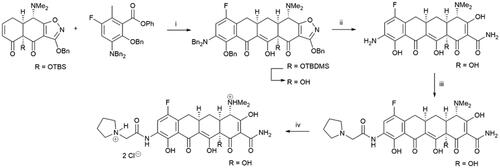
Vaborbactam (Scheme 2) is an example of a synthetic β-lactamase inhibitor that is used in combination with meropenem, an intravenous β-lactam antibiotic susceptible to degradation by metallo-β-lactamases. The FDA-approved meropenem-vaborbactam combination complements other β-lactamase inhibitors, such as clavulanic acid, a secondary metabolite of Streptomyces clavuligerus. The Klebsiella pneumoniae β-lactamase is only weakly inhibited by clavulanic acid, which therefore has no clinically relevant use against infections caused by K. pneumoniaeCitation7. Vaporbactam is a potent inhibitor of K. pneumonia carbapenemase with no observable concomitant off-target inhibition of mammalian serine proteases. However, vaborbactam does not inhibit class B metallo-β-lactamases, thus, further search for compounds with even wider β-lactamase spectrum is warranted. The key steps in the vaborbactam synthesis involve iridium (I)-catalyzed regioselective hydroboration of an allyl precursor, stereoselective chloromethylation of pinanediol boronate, and a stereospecific substitution of the chloro substituent with lithium hexamethylsilazideCitation8.
Scheme 2. Synthesis of vaborbactam. (i) TBDMSCl, imidazole, CH2Cl2, 94%; (ii) [Ir(COD)Cl]2, dppb, pinacolborane, CH2Cl2, 96%; (iii) (+)-pinanediol, THF; (iv) n-BuLi, CH2Cl2, -95 °C, THF; (v) LiHMDS, THF, -78 °C to rt; 2-thiopheneacetic acid, EDCI, HOBT, NMM, CH2Cl2, 70%; (vi) 3 M HCl, 1,4-dioxane, Δ, 64%.
![Scheme 2. Synthesis of vaborbactam. (i) TBDMSCl, imidazole, CH2Cl2, 94%; (ii) [Ir(COD)Cl]2, dppb, pinacolborane, CH2Cl2, 96%; (iii) (+)-pinanediol, THF; (iv) n-BuLi, CH2Cl2, -95 °C, THF; (v) LiHMDS, THF, -78 °C to rt; 2-thiopheneacetic acid, EDCI, HOBT, NMM, CH2Cl2, 70%; (vi) 3 M HCl, 1,4-dioxane, Δ, 64%.](/cms/asset/aabfad61-4ebe-43d0-aebd-de45bbd09579/ienz_a_2155816_sch0002_b.jpg)
Doripenem is a new thienamycin-inspired antibiotic, which can be used parenterally. Thienamycin, isolated from Streptomyces cattleya in 1976, was found highly activity against both Gram-positive and Gram-negative bacteria and is resistant to bacterial β-lactamase, however, this compound was too metabolically unstable to be further developedCitation9. The thienamycin analogue doripenem has an enhanced metabolic stability to renal dehydropeptidase-1 due to the 1β-methyl substituent in the carbapenem skeletonCitation10. Doripenem is a broad-spectrum antibiotic against many pathogenic bacteria, including the Gram-negative Pseudomonas aeruginosa. The key synthetic step (Scheme 3) is a coupling between the 4-nitrobenzyl-protected enol phosphate and substituted 3-mercaptopyrrolidineCitation11.
Scheme 3. Synthesis of doripenem hydrate. (i) DIPEA, DMF, EtOAc, rt, 88%; (ii) H2, 10% Pd/C, MgCl2•6H2O; crystallisation; sterilisation; crystallisation, 64%.

An interesting source of antimicrobial agents is the components from resins obtained from the pines Pinus sylvestris L. and Norway spruce (Piceaabies (L.) H. Karst.). The coniferous resins have antibacterial activity against Gram-positive bacteriaCitation12 due to a combination of constituents, such as abietic acid and dehydroabietic acid (). For example, various D- and L-amino acid derivatives of dehydroabietic acid disrupt Staphylococcus aureus biofilms and interact with the bacterial cell envelope causing efficient bacterial kill of S. aureus (ATCC 25923 and Newman) at low minimum inhibitory concentration (MIC 15 and 20 µM respectively)Citation13.
Recently, nanocellulose-based novel biomaterials with antibacterial properties Hassan et al. have been prepared. Thus, antimicrobial nanocellulose films have been covalently bound to (+)-dehydroabietylamineCitation14. These immobilised contact-active surfaces showed good antibacterial activity against methicillin-resistant S. aureus MRSA14TK301 and S. aureus ATCC12528 and were found to display excellent biocompatibility and a low risk of spreading resistance.
Metalloenzymes as antibacterial drug targets
Around one third of all known enzymes in prokaryotes and eukaryotes are metalloenzymesCitation15,Citation16. Bacteria contain metalloenzymes, which strongly differ from those found in mammals; often metalloenzymes produced by bacteria play a crucial role for their survivalCitation17–21. Therefore, the identification of unique bacterial metalloenzymes and the synthesis of selective inhibitors are of great interest for the development of novel antibacterial agentsCitation15–21.
Among the metalloenzymes that have been investigated as potential antibacterial drug targets in some detail during the last decade are the carbonic anhydrases (CAs, EC 4.2.1.1)Citation22–30. These enzymes act as highly effective catalysts for the hydration of CO2 to yield bicarbonate and protons, and they are involved in a multitude of physiological processes connected to pH regulation, metabolism, etc.Citation22–27. In Bacteria, four of the currently eight known CA genetic familiesCitation31,Citation32 have been described so far, namely the α-, β-, γ- and ι-CAsCitation33–35. In many species, there are CAs from more than one genetic family and frequently, more than one isoform being present. The elucidation of the role played by these enzymes during the life cycle and involvement in virulence of many pathogenic bacteria have only started recently, e.g. their inhibition proved to be bacteriostatic in many species, such as Helicobater pyloriCitation36–38, Escherichia coliCitation39, Mycobacterium tuberculosisCitation40–43 and more recently, also in the vancomycin-resistant enterococci (VRE)Citation44–46 and Neisseria gonorrhoeaeCitation47. Indeed, for the last pathogens mentioned above, sulphonamide CA inhibitors (CAIs) such as acetazolamide (clinically used for more than 70 years) together with some recently synthesised derivatives, as well as other clinically used CAIs (e.g. dorzolamide) were recently shown to outperform linezolid, which is the drug of choice for treating VRE infectionsCitation44–46. These promising results offer the long-awaitedCitation44–47 proof-of-concept for inhibition of bacterial CAs as a novel mode action for antibacterials, which are less prone to induce development of resistance. This was demonstrated for H. pylori and ethoxzolamide, in which case, a certain level of mutations was observed in several bacterial genes, including the α-CA one, but nevertheless the pathogen remained susceptible to the drug at sufficiently low, clinically relevant concentrationsCitation36–38.
Many other pathogenic bacteria besides those mentioned above encode for CAs, which may be considered as druggable targetsCitation25–30 – .
Table 1. Pathogenic bacteria and their CAs, as well as infectious disease they can cause.
Considering the worrying levels of drug resistance emerging for the classical antibiotics by many of these and other pathogensCitation17,Citation18, targeting of these enzymes with specific and selective CAIs may lead to antibacterials with an innovative mechanism.
Biopharmaceuticals; antisense peptide nucleic acid antibiotics
Peptide nucleic acids (PNAs) constitute attractive antisense agents that also represent a novel strategy to target bacterial pathogens due to their unique mechanism of action. PNA antisense antibiotics can in principle be developed against any protein target at the translational level by strong interactions with the corresponding mRNA (or directly against any functional RNA) due to the extremely strong PNA-RNA inter-oligomer binding observed when base pairing is optimal. Generally, PNA-based antibiotics will inherently exhibit a very narrow antibacterial spectrum, since their activity depends on the expression of a specific bacterial genetic sequence. Moreover, their activity is highly dependent on transport to the intracellular genetic target, and thus carrier efficiency is variable when targeting different species (or even strains).
Due to their inherent lack of a positive overall charge delivery across biological barriers in higher organisms is a severe challenge, which so far mainly has been overcome via conjugation to cell-penetrating peptides (CPPs). Likewise, low penetration through the outer lipopolysaccharide (LPS) layer of Gram-negative bacteria constitutes a barrier preventing efficient bacterial uptake of PNA oligomers. Thus, in order to achieve bacterial delivery of these large hydrophilic nucleic acid mimics conjugation to bacteria-penetrating peptides (BPPs) has proved to be a requisite for high potency, albeit the BBP may not necessarily contribute to the direct killing mechanism of the conjugate.
Brief historical introduction
In most early studies focus was on targeting bacterial genes essential for maintaining metabolism (and thus survival) or bacterial replication (e.g. acpP involved in fatty acid biosynthesis and ftsZ involved in cell division). More recent work also concern genes involved in virulence, including resistance mechanisms, as recently reviewedCitation48–50. Here, we will give a brief historical introduction followed by a review of selected highlights reported during the last 5 years.
The concept of antisense PNA-based antibiotics was demonstrated 15 years agoCitation51,Citation52, however, the approach remained severely limited by poor bacterial uptake of PNA oligomers due to their low propensity for translocation across membranes. The enhancer peptide (KFF)3K, exhibiting synergy with other antibioticsCitation53 constitutes the first example of a functional BPP capable of internalising PNA as antisense PNA-BPP conjugatesCitation52. Later, the arginine-aminohexanoyl type eukaryotic CPPs (typically based on the RXR motif; where R = Arg and X = Ahx = 6-aminohexanoic acid) were introduced for bacterial delivery of antisense PNA oligomers as well. Studies of such conjugates, targeting essential bacterium-specific genes, provided evidence that antibacterial PNA oligomers can be designed to possess low micromolar potency against Gram-negative pathogensCitation54,Citation55. Regardless of the positive clearing effects observed in mouse infection models, these KFF-based peptides neither possess sufficient stability nor favourable toxicological properties to warrant actual drug development.
Studies of factors influencing uptake efficiency
Optimisation of PNA-peptide conjugates targeting essential bacterial genes has largely focussed on the proper selection of target genes as well as the localisation of the antisense oligomer close to the ribosome binding site of the mRNA. Somewhat surprisingly, the PNA oligomer length optimum has been identified to be 10–12 residues, which is significantly shorter than seen for uptake by mammalian cells. Thus, the relationship between oligomer size, PNA-RNA duplex stability and antimicrobial activity in E. coli was recently studied in more detailCitation56. Here, a direct correlation between increased oligomer length and PNA-RNA duplex stability was evident. Nevertheless, 10-mer PNAs (within a series comprising 6- to 18-mers with either (KFF)3K or (RXR)4 as delivery moieties) proved most active as antisense antimicrobials in E. coli, inferring an upper size constraint limiting the bacterial uptake of PNA-peptide conjugates. This was corroborated by flow cytometry experiments with fluorophore-labeled conjugates. Interestingly, the size-limited uptake appeared independent of outer-membrane integrity (which is diminished in the AS19 strain), and thus the inner membrane determines the limits in molecular size for peptide-PNA internalisation into the cytosolCitation56.
Recently, the stability and uptake mechanisms as well as activity of (KFF)3K-eg1-PNA (and a series of analogues with shortened peptide parts) and the corresponding D-peptide analoguesCitation57. The SbmA inner-membrane transporter protein is known to be quite promiscuous with respect to recognition of substratesCitation58, which include proline-rich AMPs and PNA-peptide conjugates. Expectedly, the L-peptide part proved to be degraded rapidly by peptidases excreted into the culture medium as well as present within the periplasm and cytoplasm. The resulting main degradation products were devoid of antibacterial activity when applied directly to the bacterial culture. Also, for both the L- and D-peptide series a gradually reduced activity was seen with decreasing length of the peptide part (i.e. MIC values changed from >16–32 µM for the naked PNA to 2–4 µM for the full-length PNA-peptide). Expectedly, the full-length D-form (kff)3k-eg1-PNA conjugate retained antibacterial activity against the SbmA-knockout strain, however, a surprising SbmA dependence was seen for conjugates shortened by only a few amino acidsCitation57. Thus, only the full-length (kff)3k peptide is capable of transporting PNA across both the outer and inner membrane, whereas PNA conjugates with shorter peptides (both L- and D-forms) exhibit poor spontaneous translocation across the inner membrane, and hence require SbmA-mediated transport for efficient cytoplasmic uptake, and thus antibacterial activity.
One of the latest studies on peptide conjugates with PNA (H-CTCATACTCT-NH2), targeting the acpP gene expression in E. coli, concerns how the composition of LPS affects their bacterial uptake, and thus their antibacterial activityCitation59. In Gram-negative bacteria the outer membrane mainly consists of O-antigens attached to outer-core sugars (comprising 5 different monosaccharides in E. coli) linked to inner-core sugars, which are linked to lipid A. The inner core sugars comprise at least two Kdo (3-deoxy-D-manno-oct-2-ulosonic acid) residues and one or more L-glycero-D-manno-heptose (Hep) residues, and is highly conserved within a species. Firstly, E. coli MG1655 (which is devoid of O-antigen) was compared with derived strains having partially or fully restored O-antigen. Interestingly, the O-antigen part appeared to exert limited effects on the activity of (KFF)3K-eg1-PNA, (RXR)4X-(β-Ala)-PNA, and (RX)6-(β-Ala)-PNA (X = aminohexanoic acid; eg1 = ethylene glycol linker) as similar MIC values were obtained for both strainsCitation59. Intriguingly, the O-antigen-restored strain exhibited faster time-kill kinetics than the wild type (MG1655) when treated with either (KFF)3K-eg1-PNA or (RXR)4X-(β-Ala)-PNA, and hence the O-antigen part in this case may act as an apparent binding site, while for other compounds (e.g. AMPs) it is believed to prevent efficient penetration of the envelope. In addition, four inner-core mutants with increasingly shortened LPS core were found to be significantly (8- to 32-fold) more susceptible to the (KFF)3K-eg1-PNA (MICs in the range 0.03–0.12 µM) than the parent strain (MIC of 1 µM). By contrast, these mutants displayed similar susceptibility to the two Arg-rich conjugates as that of the parent strain, inferring that the outer membrane is not the rate-limiting barrier for the uptake of such conjugates. Incomplete LPS inner core enables improved access to the periplasm, and thus conjugates may more readily reach the inner membrane containing the SbmA transporter essential for the (KFF)3K-PNAsCitation59. Thus, these findings corroborate the hypothesis that uptake and activity of SbmA-transported (KFF)3K-PNA mainly is limited by translocation across the outer membrane, whereas uptake and activity of the SbmA-independent RXR-PNA type conjugates mainly is limited by inner membrane translocation.
PNA-peptide antimicrobials against Pseudomonas aeruginosa
Discovery of novel antimicrobials against P. aeruginosa, capable of inhibiting bacterial growth as well as the ensuing inflammatory response, constitutes a key goal in research aimed at alleviating progression of cystic fibrosis. Interestingly, targeting the translation initiation region of the essential acpP gene in P. aeruginosa by a known growth-inhibiting (RX)6B-PNA conjugateCitation54 was found to exert a concomitant strong inhibition of expression of pro-inflammatory chemokines and cytokines (i.e. IL-8, IL-6, G-CSF, IFN-γ, IP-10, MCP-1 and TNF-α)in IB3-1 cystic fibrosis cells infected by P. aeruginosa PAO1. In particular, the attenuation of IL-8 induction is significant due to its key role in the exacerbated pro-inflammatory cellular response induced by P. aeruginosa infection in cystic fibrosis patientsCitation60.
AMPs as delivery vehicles in PNA conjugates
Rapid resistance development against PNA-peptide conjugates has been found to be associated with mutations in the non-essential gene sbmACitation55, encoding an inner-membrane ABC transporter, for which proline-rich peptides previously has been identified as typical substratesCitation54,Citation55. Hence, novel carriers should preferably act via an SbmA-independent uptake mechanism, and such peptide carriers have been identifiedCitation55,Citation61. A recent alternative approach for achieving efficient bacterial delivery of PNA-peptide conjugates that has been explored is the use of antimicrobial peptides (AMPs) attached to the antisense PNA oligomer to provide dual-action antibioticsCitation61,Citation62.
In particular AMPs with an intracellular mode of action were found to constitute promising vehicles for bacterial delivery of an antibacterial PNA oligomer targeting the essential acpP gene in E. coli. Thus, it was demonstrated that buforin2-A (BF2-A), drosocin, oncocin, Pep-1-K, and KLW-9,13-a as well as the end-modified BF-2A-RXR and drosocin-RXR (X = aminohexanoic acid) indeed are capable of transporting PNA effectively into E. coli (with resulting MICs of 1–4 µM). Importantly, the presence of the inner-membrane peptide transporter SbmA was not required (cf. test in ΔsbmA versus wild-type strains) for antibacterial activity of PNA-AMP conjugates containing Pep-1-K, KLW-9,13-a, or drosocin-RXR. Consequently, certain AMPs (and modified analogues thereof) provide efficient transport of PNA into the bacterial cytoplasm with the AMP moiety appearing to act simultaneously at a separate target (e.g. the ribosome)Citation61]. Similarly, N-terminally modified drosocin (i.e. RXR-PRPYSPRPTSHPRPIRV; X = aminohexanoic acid, Ahx) and a truncated Pip1 peptide (i.e. [RXR]2-IKILFQNRRMKWKK; X = Ahx) were subsequently studied. The drosocin-derived compound without a linker moiety exhibited the highest antibacterial activity against both wild-type E. coli and K. pneumoniae (MICs in the range 0.3–0.7 µM), while analogues displaying an ethylene glycol (eg1) moiety or a polar maleimide linker were found also to exhibit activity towards wild-type K. pneumoniae (MICs of 0.6–1.3 µM). Against two colistin-resistant E. coli strains the linker-deficient compound proved most potent (with MICs in the range 0.3–0.7 µM). Importantly, the antisense PNA-drosocin conjugates had potent antibacterial activity against colistin- and tigecycline-resistant E. coli and K. pneumoniae without exhibiting concomitant haemolytic propertiesCitation62.
Cationic dendrons for delivery of PNA oligomers
Besides Arg-based modification of AMPs to obtain more efficient delivery vehicles for PNA, a recent work reports on guanidinylated dendrimeric delivery moieties for PNA targeting the translation of the acpP mRNA essential for fatty acid synthesisCitation63. Based on a series of 2,4-diaminobutanoic acid (Dab)-based dendrons, the structure-activity relationships (SARs) were explored with respect to the effects of terminal guanidinylation as well as the length (and thus hydrophobicity) of the terminal linear cationic carbon linker (C4 to C8) moieties. Expectedly, the compounds with terminal amino groups had low activity towards E. coli (i.e. MICs above 16 µM). Conversely, the corresponding guanidinylated derivatives exhibited MIC values in the ranges 0.25–2 µM and 0.125–8 µM against E. coli and K. pneumonia, respectively. The C8 linker conferred highest activity, however, the mismatch PNA conjugate also possessed substantial activity (MICs of 4–8 µM) that appeared to arise from membrane-disruptive effectsCitation63. To assess the influence of the size of the core dendritic structure on antibacterial activity, two similar third-generation dendrons with a core of 2,3-diaminopropanopic (Dap) residues were examined as well. However, only slight differences in activity between the Dap- and Dab-based conjugates were observed, implying that the outer shell of the dendron is most important in determining the antibacterial properties of dendron-PNA conjugates. The guanidinobutanoyl-modified Dab-based dendron with a charge of +8 was subjected to further studies, which showed: (i) SbmA-independent activity, (ii) low cytotoxicity against the liver cell line HepG2 (full viability at 90 µM), (iii) MICs increased in the presence of high concentrations of divalent cations (Ca2+ and Mg2+), (iv) bactericidal mode of action, (v) high stability in mouse and human serum, with t1/2 ≫24 h, (vi) in vivo activity against a multidrug-resistant E. coli in a murine peritonitis modelCitation63.
PNA-peptide antimicrobials against Klebsiella and Acinetobacter species
In another recent study, a PNA conjugate with the (KFF)3K CPP targeting gyrA in KPC-producing K. pneumoniae was found to inhibit bacterial growth in vitro. The suppressive effect on the expression of the gyrA gene was evaluated along with the haemolytic properties towards mouse erythrocytes. The conjugate was capable of inhibiting bacterial growth at 50 µM, with a concomitant reduction of the 16S gene amplification by ca. 97%, while 21% haemolysis was observed at this concentrationCitation64.
Nosocomial infections caused by carbapenem-resistant Acinetobacter baumannii (CRAB) are emerging as increasingly difficult-to-prevent and -treat diseases. A series of BPP-PNA oligomers targeting the translation initiation region of the ftsZ, acpP, and rne genes of CRAB strains, comprising the antisense conjugates (KFF)3K-eg1-(acpP)PNA, (KFF)3K-eg1-(ftsZ)PNA, and (KFF)3K-eg1-(rne)PNA, were found to exhibit complete growth inhibition against several CRAB strains and isolates at concentrations in the range 1–2 µM, while the compounds were bactericidal either at the MIC or at 2-fold higher concentrations. Expectedly, the mode of killing did not involve a concomitant membrane disruption. In addition, the conjugates exhibited low cellular toxicity in the HepG2 cell line (up to 20 µM) and did not show significant antibacterial activity against other Gram-negative pathogens (i.e. E. coli and P. aeruginosa), indicating a potential for specific treatment of CRAB infectionsCitation65.
PNA-peptide antimicrobials against other gram-negative pathogens
Also, other Gram-negative pathogens have been considered as potential targets for PNA-based conjugates. For example, six PNA-peptide conjugates, displaying (RFF)3R at one of the PNA termini, were designed to target the expression of the gene for the acyl carrier protein (acpP) in non-typeable Haemophilus influenzae (NTHi), and these displayed MICs in the range 0.6–2.5 µM against 20 clinical isolates. Biofilm eradication (as measured by biofilm-eradicating concentrations = MBECs) was only seen at significantly higher concentrations (i.e. MBECs up to 40 µM). Resistant strains could be selected by serial passages upon initial exposure to sub-MIC concentrations that were gradually increased. A resistant strain had an SNP predicted to affect an ATP-binding protein of a conserved ABC transporter. Interestingly, insertion of an ethylene glycol linker (eg1) between PNA and delivery peptide affected the propensity for resistance development, since the wild-type strain remained susceptible to this conjugate even after 30 serial passagesCitation66.
In addition, PNA conjugates targeting two Gram-negative pathogens, critically associated with periodontitis, have been designed and evaluated as antisense antibiotics for selective growth inhibition of Porphyromonas gingivalis and Aggregatibacter actinomycetemcomitans. Antisense PNAs targeting groEL or acpP were conjugated to the traditional delivery peptide (KFF)3K. In P. gingivalis the anti-groEL PNA conjugate inhibited growth for 5 h at a concentration of 3 µM. Anti-groEL PNA against A. actinomycetemcomitans inhibited growth for 2 h at a concentration of 3 µM, with an ensuing reduced GroEL protein expression. In contrast, anti-acpP PNA exhibited no marked growth-inhibitory effect on these species. Thus, anti-groEL PNA-peptides appear to constitute potential species-specific antibacterial tools against oral pathogensCitation67.
In a comparative study of KFF-PNA, RXR-PNA and Tat-PNA anti-acpP conjugates [i.e. (KFF)3K-, (RXR)4XB-, and GRKKKRRQRRRYK-ctcatactct], global RNA-seq analysis was used for the first time to investigate how the transcriptome in Salmonella enterica is affected upon exposure to the same PNA oligomer when delivered by different carrier peptidesCitation68. Most previous studies have focussed on MIC determinations to assess activity of peptide conjugates with PNA oligomers designed to prevent ribosomal interaction with the target mRNA, however, the peptide part may trigger pathways protecting the integrity of the bacterial envelope. Indeed, besides inhibition of protein synthesis, a rapid acpP mRNA decay occurred, the exact mechanism of which remains to be elucidated. Intriguingly, conjugates with different carrier peptides did not only exhibit different bactericidal activity (MICs in the range 1.25–5 µM, with KFF-PNA being the most potent), they also triggered acpP-independent stress pathways. In particular, KFF-, RXR- and Tat-PNA conjugates all induced the PhoP/Q response, while the last two also induced upregulation of gene expression related to several membrane proteins and transporters.
Alternative targets for PNA-based antimicrobials
Intriguingly, alternative targets for PNA-based antimicrobials have been explored with a successful outcome. Thus, the bacterial signal recognition particle (SRP) was considered as a potential antibacterial target, for which proof of principle was achieved by using an antisense (KFF)3K-PNA conjugate to target a key SRP-related RNA-protein interaction. In liquid culture, one PNA conjugate inhibited the growth of E. coli AS19 cells in a dose-dependent manner with complete growth inhibition at a concentration of 2.5 µM. Transmission electron microscopy showed that deformed cell structures were seen for PNA-treated bacteria. Depending on the targeted RNA site, this strategy may be tailored either to generate broad-spectrum PNA-based antibacterial compounds, or to identify sequence-specific inhibitors for drug-resistant pathogensCitation69.
Bacterial toxin–antitoxin (TA) systems comprise genetic modules that encode a growth-arresting protein toxin (acting by interference with essential cellular processes), and a cognate antitoxin, which neutralises the activity of the toxin. TA systems have no human homologs, but they are abundant in bacterial genomes, and they thus constitute attractive alternative antibacterial targets. Artificial activation of E. coli mazEF and hipBA toxin–antitoxin systems was explored as an innovative approach, in which arrest of growth in E. coli was induced via inhibition of translation of the antitoxins by antisense (KFF)3K-PNA oligomers. The MIC of the anti-mazE PNA conjugate was 16 µM in three different E. coli strains, while the anti-hipB PNA inhibited growth of E. coli K-12 and WR3551/98 with MICs of 8 and 16 µM, respectively. Furthermore, the combination of anti-thyA PNA with trimethoprim resulted in a highly synergistic interaction (FICI = 0.31; for the combination 4 µM PNA + 0.04 µM antibiotic). These findings infer that TA systems constitute interesting, but so far poorly explored targets for antisense agentsCitation70.
Plasmid-mediated drug resistance is accelerating the spreading of polymyxin resistance, resulting in only a few or no remaining therapeutic options for treatment of infections caused by certain Gram-negative MDR bacteria (e.g. carbapenemase-producing strains). In order to restore susceptibility to colistin in Enterobacteriaceae, PNAs designed to specifically target mcr-1 expression were exploredCitation71. In particular, a 12-mer PNA-peptide conjugate [AACTACTCAAAA-O-(KFF)3K] targeting a mRNA sequence (at the ribosome-binding site that is conserved in nine mcr-1 variants) proved capable of complete inhibition of the expression of mcr-1 at a concentration of 4 µM, thereby conferring an increased susceptibility to colistin (with a MIC decreasing from 8 to 2 µg/mL). Importantly, 4 µM of this PNA-peptide conjugate improved colistin susceptibility in several mcr-1-positive E. coli strains (35 out of 37 tested) with MICs decreasing from 4–16 µg/mL to 1–2 µg/mL. Encouragingly, this strategy may increase the lifespan of the critical polymyxin antibiotics, albeit the toxicity and other potential side effects of such PNA conjugates require further in vivo studies to assert their safety as preclinical drug candidates.
Ribonucleotide reductases (RNRs) constitute the rate-limiting enzymes involved in de novo synthesis of DNA precursors via reduction of ribonucleotide di-/tri-phosphates to deoxyribonucleotides di-/tri-phosphates. Escherichia coli possesses genes encoding an iron-dependent class Ia RNR, which is essential for viability during aerobic growth (i.e. nrdAB; with nrdA and nrdB transcribed as a polycistronic mRNA with the nrdA gene located upstream of the nrdB gene). A PNA-peptide conjugate ([KFF]3K-eg1-ATGTATGTCG-NH2) was designed to target E. coli nrdA, and this anti-NrdA-PNA indeed inhibited bacterial growth with MIC value of 4 µMCitation72. In addition, during treatment DNA synthesis rate was found to decrease, resulting in incomplete chromosome replication. It is proposed that reduced DNA replication is caused by dNTP depletion, which eventually causes an accumulation of double-stranded DNA breaks. Importantly, anti-NrdA-PNA treatment did not influence protein synthesis, corroborating the intended mode of actionCitation72.
PNA-peptide antimicrobials against gram-positive pathogens
Recent examples of a PNA-based antibacterial strategy against Gram-positive pathogens have also been reported. Streptococcus pyogenes are a human pathogen causing a wide range of infections that usually can be treated with penicillins, albeit cases of therapeutic failure have emerged. Also, streptococcal resistance to alternative antibiotics (e.g. macrolides) is common. Previously, the HIV-1 Tat peptide coupled to anti-gyrA PNA was shown to inhibit growth of S. pyogenes, but later an investigation of 18 CPP-coupled anti-gyrA PNAs on S. pyogenes was undertakenCitation73. Here, HIV-1 Tat, oligolysine (K8), and (RXR)4XB (X = Ahx; B = β-Ala) conjugates with anti-gyrA PNAs were found to inhibit bacterial growth in vitro. Treatment with these three PNA-CPPs led to increased survival of larvae in a Galleria mellonella infection modelCitation73.
Nosocomial infections caused by antibiotic-resistant Enterococcus faecalis have increased prevalence, and thus novel strategies are needed. The efaA gene (presumed to be involved in biofilm formation) was targeted by an antisense (RXR)4-PNA conjugate, which was designed to interact with the start codon section of the efaA gene. Biofilm inhibitory effect of the conjugate was seen at 10 µM, where expression of the efaA gene was essentially abolished, while biofilm formation was reduced by 60% after 24 h treatment. In addition, in an MTT assay the conjugate was devoid of toxicity on MCF7 cells at the active concentrationCitation74.
A novel transport mode for PNA-peptide antimicrobials
A novel transport mode for antibacterial antisense PNA oligomers was recently explored. Besides CPPs and BPPs new types of bacterial delivery vehicles for antibacterial PNAs should be considered, and the vitamin B12-PNA conjugates indeed constitutes a novel approach for enhancing bacterial uptake of PNA oligomers. Thus, vitamin B12 was covalently linked to a PNA oligomer targeting the mRNA of the acpP gene in E. coli either via a stable linkage or via a cleavable disulfide linkage. The MICs of vitamin B12 − PNA conjugates against E. coli were 5 µM, similar to that of the corresponding (KFF)3K-PNA control. Moreover, vitamin B12-PNA conjugates proved to be stable in the presence of biological matricesCitation75.
Combination therapies: synergy and sensitisation
A combination of antibacterial agents is considered to make the emergence of resistance in bacteria less probable. Thus, potential synergy between antibacterial antisense PNA-peptide conjugates and conventional antibiotics was tested in the E. coli AS19 (lipopolysaccharide defective) strain and in a modified pathogenic strain E. coli O157:H7. Herein, PNAs were designed to target acpP (i.e. anti-acpP PNA) and conjugated to (KFF)3K. Antibiotics studied included aminoglycosides, aminopenicillins, polymyxins, rifamycins, sulphonamides and trimethoprim. Two novel synergistic combinations were identified, namely (KFF)3K-PNA and polymyxin B as well as (KFF)3K-PNA and trimethoprim - both with a fractional inhibitory concentration index (FICI) of 0.38. Synergy with polymyxin B indicates that antibiotics impairing the integrity of the bacterial envelope may be attractive agents for improving uptake of PNA conjugates, whereas trimethoprim/PNA-peptide synergy may arise via a hitherto unknown mechanismCitation76.
Likewise, the lpxB gene in A. baumannii was evaluated as a potential therapeutic target for antisense PNAs either alone or in combination with known antimicrobial therapies. RNA-seq analysis of A. baumannii ATCC 17978 showed that the lpxB gene was overexpressed in a murine pneumonia model. Expression in vivo of LpxB during treatment with anti-lpxB (KFF)3K-PNA was inhibited in vitro, and gave rise to decreased bacterial survival while increasing the survival rate of infected A549 cells. Synergy was observed between this conjugate and colistin in colistin-susceptible strains. Test via an in vivo Galleria mellonella infection model confirmed that combination treatment with anti-lpxB (KFF)3K-PNA and colistin was more effective than colistin monotherapyCitation77.
Most recently, a larger study on the potential of PNA-peptide conjugates as sensitisers for antibiotics in wild-type E. coli and P. aeruginosa was reportedCitation78. Here, PNA oligomers were designed to silence gene expression of bamB and tolC, coding for a component of the outer membrane protein assembly complex (β-barrel-assembly machinery) and TolC (part of the substrate-promiscuous AcrAB-TolC efflux pump complex), respectively. Firstly, KFF-bamB [(KFF)3K-eg1-catcgggtcc] and KFF-tolC [(KFF)3K-eg1-tgcattcctt] at low concentrations (i.e. 5 µM) were shown not to exert significant growth inhibition alone (but at high concentrations they caused a slight growth delay). Interestingly, KFF-bamB decreased the MICs in E. coli of novobiocin, and fusidic acid by 16-, and 4-fold, respectively, while the combination of KFF-bamB and KFF-tolC decreased the MIC of these antibiotics even more pronounced (>1000-fold to below 0.4 µg/mL). To expand this sensitisation approach to P. aeruginosa, conjugates targeting bamB and oprM (a tolC homolog) were designed: RXR-bamB [(RXR)4-XB-catatcattg] and RXR-oprM [(RXR)4-XB-tcaggcctct]. These were applied at a concentration of 3 µM together with antibiotics that were inactive alone (vancomycin, erythromycin and carbenicillin). In the presence of RXR-bamB carbenicillin MIC was reduced from 64 to 8 µg/mL), while the combination (1:1) of RXR-bamB and RXR-oprM reduced the MICs of carbenicillin and erythromycin from 64 and 128 µg/mL to below 4 and 16 µg/mL, respectively. This constitutes the first report on the potential of bamB as a target of antisense PNA-based conjugates, and the increased effect when applied in combination with conjugates targeting oprM indicates that it will be possible to design sensitiser cocktails to combat various pathogens with different resistance mechanisms.
Another approach for enhancing activity of antibiotics is the combination therapy involving membrane-permeabilizing compounds. In this context the polymyxin-derived NAB741Citation79 (displaying a neutral Ac-Thr-D-Ser tail instead of the C8/C9 fatty acyl-Dab-Thr-Dab with two cationic charges; Dab = 2,4-(S)-diaminobutanoic acid), was at a sub-MIC concentration (1.6 µg/mL) found to provide >10-fold improved antibacterial activity of (KFF)3K-eg1-PNA in wild-type E. coli (MG 1655 strain)Citation57. Interestingly, the effect was even more pronounced for conjugates with shortened peptide parts, e.g. the activity was enhanced 32-fold for the KFFK-eg1-PNA (i.e. a reduction in MIC from 16 to 0.5 µM). This large enhancement of antibacterial activity by NAB741 infers that transport across the outer membrane constitutes the main barrier when SbmA is present as an efficient inner-membrane transporter. In contrast, only a 2- to 4-fold enhancing effect of NAB741 was found for the D-form KFF-based conjugates, indicating a different mode of outer-membrane passage, a less efficient inner-membrane translocation by SbmA, or an alternative mechanism for crossing the inner membraneCitation57.
Conclusive remarks
Conclusively, antisense PNA-peptide conjugates constitute a unique compound class amenable to rapid drug discovery as medicinal chemistry on the active principle can be performed without significantly affecting its affinity for the therapeutic target (i.e. typically a species-specific mRNA involved in a process essential for bacterial survival). We and others have characterised the drug potential of PNA-peptide conjugates, of which several have been tested in mouse infection models. However, further optimisation towards lower toxicity and increased efficacy in in vivo settings is clearly warranted, and for these reasons no antibacterial agents belonging to this compound class have as yet entered clinical trials.
In vivo models to study biofilm-related respiratory infections
Bacteria can grow as single cells (planktonic growth state) or as a sessile community, also known as a biofilm. According to the National Institutes of Health (NIH), biofilms are involved in over 80% of all bacterial infectious diseases. Due to the increased resistance of biofilms to antibiotics, alternative therapeutic options are urgently needed.
To develop novel antimicrobial strategies, standardised and optimised in vitro and in vivo models are needed. To bridge the gap between in vitro findings and clinical relevance, animal models are requiredCitation80. These models differ in genetics, physiology and biochemistry, but are used under controlled and ideal lab circumstances, thereby minimising variables such as sex, age and environmentCitation81,Citation82.
While it is clear abandoning all animal experiments for the sake of animal welfare is impossible, as both computational and experimental in vitro and ex vivo models cannot consider all variables and individual variations essential to an in vivo situation, efforts to reduce their need are being madeCitation83. Alternative in vivo models are gaining attention as these models are often more simplistic compared to complex vertebrate models. They are not able to fully replace animal experimentation but could be an extra step in- between in vitro and in vivo experiments, leading to a reduction of laboratory animalsCitation84–86. Invertebrate models such as insects and fish are commonly used in the academic area, especially in the larval stageCitation87.
Although important information has been generated using in vitro and invertebrate models, the use of mammalian models that are more closely related to humans is required for going from bench to bedside. It is challenging to mimic BRI in higher organisms, but this challenge has to be tackled in order to address diagnostic, preventive or therapeutic solutionsCitation88.
In vivo biofilm models
Non-human mammalians, i.e. mice and rats, are mostly chosen to unravel the in vivo pathogenesis of bacterial lung infections or to evaluate the in vivo efficacy of new antibacterial therapies or prophylactic agents. The use of mice as a laboratory animal species has many advantages, including the availability of a wide arrange of molecular and immunology laboratory techniques specific for mice, the easy housing and care and the requirement of low quantities of test compounds due to the small size of mice making it a cost-efficient modelCitation89. Extrapolation of results obtained from mouse lung infection models to humans is not straightforward due to significant anatomical and physiological differences between mice and human. Despite the differences in lung anatomy and respiratory parameters between mouse and human, similarities are present when comparing pneumonia in both species. One important similarity is the accumulation of the polymorph exudate in the alveoli of pneumonia patients as wells as in murine lungs infected with bacteria.
Differences between the murine and human lungs are well documented, such as differences in structural anatomy, tracheobronchial epithelium, local phagocytic and chemical defences and immune responseCitation87,Citation90. It is important to consider those differences when extrapolating data from in vivo experiments. The murine lungs exhibit a monopodial branching pattern as opposed to the symmetric branching pattern in human lungs. Murine lungs have therefore fewer respiratory bronchioles than human lungsCitation91. The airways of mice terminate abruptly into alveolar ducts without intervening to respiratory bronchiolesCitation92,Citation93. The cellular composition of the murine tracheobronchial epithelium differs due to the presence of less mucous and serous cells and the absence of submucosal glandsCitation94–96. Some characteristics of the antimicrobial molecules, that are a part of the innate lung defense, differ as well. Differences in local phagocytic defences and cellular expression and ligand binding for some toll like receptors were also observed for both speciesCitation97,Citation98.
Chronic pneumonia models can be used to study biofilm formation of P. aeruginosa as well as the influence of single virulence factors or to study co-infection with other pathogens (e.g. B. cenocepacia or S. aureus)Citation87,Citation99–107. The P. aeruginosa lung infection in these chronic models can be challenged with antibacterial and anti-biofilm therapies to evaluate their efficacyCitation108–114. Disease models such as CF knock-out mouse models were also developed to study P. aeruginosa or other bacterial lung infections. Models of P. aeruginosa lung infection can be divided by their route of infection in intratracheal or intravenous modelsCitation88. Intratracheal models represent the clinical situation better. A relevant model was developed by Cash et al., 1979Citation115 using immobilised bacteria in agar beads. Pedersen et al., 1990Citation116 developed another model by embedding the bacteria in seaweed alginate microspheres.
Device related infections: ventilator-associated pneumonia (VAP)
Hospitalised patients are at high risk of morbidity and even mortality from healthcare- associated infections (HAIs), in addition to their primary illness. According to the ECDC, 8.9 million European patients acquire infections in hospitals and long-term care facilities every yearCitation117. HAIs cause over 90,000 deaths per year in the European countriesCitation118, more than any other infectious disease under surveillance. And hospital-acquired pneumonia is the second most common nosocomial infection and the most common cause of death from HAI in critically ill patientsCitation119. Ventilator-associated pneumonia (VAP) is a serious hospital-acquired pneumonia which is defined as lung infection acquired by patients mechanically ventilated in the intensive care unit for at least 48 hCitation120. Patients with VAP have increased length of hospitalisation and consequently increased health costCitation121,Citation122.
Endotracheal tube microbial colonisation is a major risk factor for VAP and a multispecies biofilm of commensal micro-organisms forms in 90% of the tubes used in mechanical ventilationCitation123. This biofilm presents a clinical challenge since pathogens can attach to the microbial community formed on the inner surface of the endotracheal tube. Carried by airway pressure, they can then bypass normal barriers and reach the pulmonary parenchyma or infect the lungs. In a prospective observational study that included patients from 27 European intensive care units (ICUs), S. aureus is reported as the most common Gram-positive isolate in VAP patients and P. aeruginosa, A. baumannii and E. coli are among the most common Gram-negative isolatesCitation124. Mortality rates can vary in different patient groups and depend on underlying conditions and disease severity. In a meta-analysis using randomised trials and considering a homogeneous group of patients, the overall attributable mortality associated with VAP was 13%Citation125.
To address this high risk of mortality from VAP, several strategies have been investigated such as modifying endotracheal tubes in order to make their surface anti-fouling and/or prevent biofilm formation by incorporating substances with antibacterial properties during the manufacturing processCitation126. The limited number of available solutions is due to the complex process of developing reliable devices, starting from idea up to the market. For a successful development, an interdisciplinary approach is essential, requiring drug development, material science, engineering, clinically relevant animal models and, finally, well-designed clinical studies. Thus, the availability of tools to produce testing samples for each step of characterisation and validation is key to achieve the required results.
Several animal models have been developed to study preventive or curative strategies for VAP, using mice, sheep, pigs or dogs. They all rely on tracheal intubation of the animal with endotracheal tubesCitation88,Citation127. Each animal model has its own advantages and limitations. The benefits of using a mouse model are their small size and relatively rapid reproductive rate. There are also genetic and physiological similarities to humans and genetically engineered mutant mice strains, such as CF mouse modelsCitation128,Citation129. The costs of a mouse model are limited. Disadvantages are that the anatomy and physiology of their respiratory tracts differ from humans. For instance, mice have no respiratory bronchioles and a weak cough reflex. Other limitations are long-term mechanical ventilation is unfeasible, there is limited ability to provide critical care to severely ill animals and, therefore, using mouse experiments to predict the long-term effectiveness and safety in humans remain controversialCitation130.
Murine models of VAP can be divided regarding the necessity or not of surgery (tracheotomy) and the possibility or not of mechanical ventilation. A research group at Nagasaki University has been using endotracheal intubation in mice as the preferred non-invasive procedure to study VAP treatment options against P. aeruginosaCitation131–134. However, this model uses tubes with shorter length and direct infection of the lungs and does not include the possibility of studying the effects of the biofilm formation on the endotracheal tube. Surgery has been used not only in mice but also in rat models, to study the effects of inhalation therapy with or without simultaneous mechanical ventilationCitation135–137. However, the time of the experiment is shortened to 4 h maximum when ventilation is performed. To study this aspect and mimic VAP appropriately other mammal models such as sheep, pigs and dogs must be employed, since only after 48 h ventilation the models would resemble the clinical situationCitation138–141. Most models test a monospecies infection with P. aeruginosa, the most frequent pathogen associated with VAPCitation121,Citation142.
Shaqour et al. developed a test system for evaluating novel antimicrobial compounds in a VAP mouse model. Therefore, the tubes produced with this novel design were loaded with ciprofloxacin (CPX), a well-known antibiotic for treating S. aureus VAP. The advantages of incorporating CPX in the tubes are its thermal stability and low cost. From a clinical perspective, CPX should not be used prophylactically, since it could be used for treatment of VAP caused by Gram-negative bacteriaCitation143. The tubes were not cytotoxic, and their antibacterial properties were demonstrated by zone-of-inhibition (ZOI) and quantification of the planktonic bacteria. Their antibiofilm properties were also confirmed by quantification and visualisation of the attached bacteria.
Animal models of device-related infections can be divided in site-specific and subcutaneous models. The VAP mouse model is a site-specific model and allows the evaluation of the host response to endotracheal tubes located in the same position as in the clinicCitation88. Other animal species have been used to study BRIs in endotracheal tubes such as sheep, pigs and dogsCitation138,Citation140,Citation141. Mice have the advantages of their small size, relatively high reproductive rate and the availability of antibodies, increasing the chance to bring new technologies and drugs from bench to bedside. We used fully mature adult mice to ensure that the tubes fit in the trachea. Outbred SWISS-CD1 are used in all areas of biomedical research and are more resistant to infections than the inbred mice as reported in a P. aeruginosa VAP modelCitation144.
The challenge to establish a VAP mouse model lies not only in the technical, but also in the biological aspects. The selection of a bacterial strain is fundamental for the success of murine modelsCitation88. The benefit of this animal model is the relative ease to establish a chronic infection (≥ 3 days)Citation127,Citation145 with S. aureus ATCC 25923. The limitation is that the delivery of bacteria at implantation of the device is initially different between the CPX and the TPU tubes, since the bacterial viable numbers are reduced during the pre-incubation step due to eluting CPX. However, in the clinical situation an endotracheal tube is placed in the trachea in contact with the fluid lining of the tracheal epithelium. In this humid environment, CPX elution will also start at the same time as the first contaminating bacteria will reach the tube. Thus, it might be argued that the exposure of the bacteria to CPX in the pre-incubation step represents the situation that may occur in vivo. In future studies, it would be interesting to focus on the pathophysiology by inserting non-colonized tubes and subsequently exposing mice to the bacteria. Other bacterial strains and even species could be used for this purpose, such as P. aeruginosa, since chronicity of infection has been previously obtained with the appropriate strain and methodologyCitation107,Citation131.
Tissue-related infections: chronic infection models based on alginate or agar beads
Mice are inherently more resistant to infections caused by human bacterial lung pathogens. Two methods can be used to overcome this problem. Immunosuppression, i.e. suppression of the cell-mediated immunity, mostly by PMNs, makes mice more susceptible to human bacterial lung pathogensCitation146. However, considering the high virulence of the bacteria used in this study immunosuppressing the mice would further compromise their well-being. An alternative for immunosuppression is embedding bacteria in beads followed by pulmonary infectionCitation147. Bacteria are embedded in an immobilising extracellular matrix formed by agar, agarose or seaweed alginateCitation99,Citation101,Citation115,Citation116. This embedding technique results often in a chronic lung infection as bacteria are protected from the host immune system inside the beads instead of being quickly removed from the lungs. Chronic P. aeruginosa lung infections are characterised by the presence of protective biofilm structures. This adaptive strategy of P. aeruginosa contributes to their persistence by avoidance of the immune system or by hindering the activity of antibacterial therapies. Cash and collaborators developed a chronic P. aeruginosa lung infection mouse model by embedding bacteria in agar beads following administration to lungs of ratsCitation115. The use of agar beads bypass the primary host airway defense mechanisms such as the interaction between bacterial adhesion molecules and respiratory epithelial cells. In this way, the initial colonisation phase of P. aeruginosa is avoided. The use of these P. aeruginosa agar beads resulted in retention of bacteria in the airways leading to a persistent stimulation of the host immune system which is typical for CF lungs. A disadvantage of this model is that the extensive neutrophil influx in response to the P. aeruginosa agar beads may cause airway obstruction and consequently lead to ineffective gas-exchange. A small percentage of animals may die after the infection procedureCitation148. An advantage is that with this model lung infection can last more than one month in lungs of infected rats and cause inflammation and tissue damage that is similar to the one observed in CF patientsCitation115. Pedersen and collaborators adapted this chronic lung infection model by using seaweed alginate beads instead of agar beadsCitation116. A major advantage of the use of seaweed alginate is to mimic closely the natural CF lung conditions, in which mucoid P. aeruginosa are present in biofilms and the extracellular matrix of these biofilms consist mainly of pseudomonal alginateCitation116,Citation149. This model was modified by Starke et al., 1987 for Swiss miceCitation101 and optimised to cause chronic infection in BALB/c mice by Moser et al., 2009Citation107.
Methods to battle biofilm-related infections
Quorum sensing inhibitors
It is well known that growth of bacteria in biofilms can lead to increased resistance against antimicrobial therapies, caused by the decreased growth rate of the bacteria, the inaccessibility of the bacteria inside the biofilm and the possible presence of persister cells. For these reasons antibiotic therapy failure is commonly seen in BRI’sCitation150. Therefore, novel strategies need to be developed to battle biofilm-formation.
An important new target is the bacterial communication system, quorum sensing (QS). The QS-system consists of extracellular signal molecules that are produced and secreted by bacterial cells to coordinate their behaviour in a cell-density dependent manner and thereby synchronously altering their gene expression. The main QS-mechanism used in gram-negative bacteria is the production of acyl homoserine lactone (AHL) signalling moleculesCitation151. Although the involvement of QS in biofilm formation is not yet fully elucidated, quorum sensing inhibitors (QSI) have been proposed as promising anti-biofilm agents. Several methods of QS inhibition have been proposed including the inhibition of signal synthesis or degradation of the signal, receptor inhibition or directly interfering with the downstream signalling cascadeCitation152,Citation153.
Compounds interfering with signal synthesis mostly relate to analogs of S-adenosylmethionine (SAM) which is the amino donor for the formation of the homoserine lactone ring moiety. These include S-adenosyl homocysteine, sinefungin and butyryl-SAMCitation154,Citation155. Also, the antibiotic azithromycin has been shown to interact with the C4-homoserine lactone synthesis leading to reduced adherence to polystyrene surfacesCitation129,Citation156.
The main target of the inhibition of the QS-signalling complex is the enzymatic degradation of the signal molecule also called Quorum Quenching (QQ), including AHL-lactonase and -acylase as well as oxidising and reducing agents like paraoxonase and oxidoreductases. These are all responsible for the breakdown of AHL leading to reduced accumulation and biofilm formation by the sessile bacteriaCitation153,Citation157–160.
A third method in inhibiting QS is receptor inhibition of mostly the LuxR-homolog receptor. This was done by using AHL-analogs that match this receptor. They mostly differ from AHL by replacing the lactone ring by a cyclopentyl or cyclohexanone ring. In this way, a signal-receptor complex is generated that is competitive to the native active receptor-complex leading to a disruption of the downstream signalling cascadeCitation161–163. Besides these, also unrelated compounds resembling AHL are used that can be found in nature. This includes bergamottin (component of grapefruit juice), extracts of Penicillium species (patulin) and garlic extracts (ajoene)Citation164–167.
Microbiome-based therapy
Due to increasing treatment failure because of antibiotic resistance and the unfavourable cytotoxic properties of QS-inhibitors different treatment strategies have been investigated in the past decade. One of the strongest candidates is the use of probiotics in the battle against biofilm-infectionsCitation168. Probiotics can be used to reduce the adherence of pathogenic bacteria to surfaces as well as hinder their activityCitation169,Citation170. Besides that, they also interact with QS-mechanisms, biofilm formation and survival of pathogenic bacteria leading to a reduced biofilm-quality and ultimately eradication of the bacterial biofilm. Mechanisms used by the probiotics include the creation of unfavourable conditions (pH alterations, surface and nutrient competition) and the production of antagonists (e.g. surfactants, enzymes,…)Citation171–174. These agonists lead to disruption of the bacterial membrane, altered gene expression in pathogenic biofilms and modulation of the host immune response to the biofilmsCitation175–177. All of the above mechanisms have been proven to be methods employed by different probiotic bacteria including Lactobacillus spp., some Streptococcus species like S. salivarius and S. oralis and Bifidobacterium lactis and B. longumCitation168. Cell free supernatants or membrane vesicles from probiotic strains can be used alone or in combination with the probiotic bacterial cells as an innovative antimicrobial formulationCitation178.
Another bacterium that is associated with the Cystic Fibrosis (CF) respiratory tract is Rothia mucilaginosa. Research has pointed out that Rothia-species exhibit an anti-inflammatory effect in CF-lungs as has been shown in both in vitro and in vivo models by Rigauts et al. Besides that, in a cohort of patients with bronchiectasis, a negative correlation has been observed between the presence of Rothia and the expression of pro-inflammatory markers (IL-8, IL-1β) in the sputum. The proposed mechanism by which Rothia exhibits its function is the inhibition of the NF-κB pathway activation by reducing the phosphorylation of IκB-α leading to a decreased expression of NF-κB target genesCitation179.
Antibiotic drug delivery nanosystems
The inappropriate use of antibiotics and new bacterial defence mechanisms has led to a substantial decrease in the efficacy of traditional antibiotics. The high number of antimicrobial resistance (AMR) cases against key antimicrobial groups represent a serious threat to patient safety in EuropeCitation180. Therefore, it is essential to develop new approaches for killing bacteria and to avoid development of AMRCitation181. Development of new drug delivery systems is a powerful tool to enable alternative mechanisms for systemic administration and for a controlled release of a drugCitation182. These systems comprise vesicular systems, such as liposomes or niosomes. Extracellular vesicles (EV) have also a potential as drug delivery systems due to their role in intercellular communication and in transfer of biological informationCitation183.
Nanotechnology and tissue engineering have been utilised in the elaboration of nanoparticle (NP) based antimicrobial agents. Some of the potential advantages of NPs in the fight against microorganisms are their low propensity to generate resistance, and they constitute a safe alternative to facilitate clinical use of otherwise difficult-to-administer drugsCitation184,Citation185
Encapsulation of an antibiotic in a niosomal formulation may enhance its antibacterial efficiency. Due to the compatibility of the physico-chemical properties between antibiotics and vesicles encapsulation of traditional antibiotics in nanovesicles may be achievableCitation186.
In the last decades development of antibiotic drug delivery systems can be divided into two main directions: (i) evaluation of different kinds of nanovesicular systems and (ii) their applications. There are different types of nanoformulations available for antibacterial applications. Mainly nanoparticulate drug delivery systems formed by either an organic or inorganic core, or hybrid nanosystems consisting of both components are may be used for these applicationsCitation187–190. The NPs classes being explored in antimicrobial chemotherapy are summarised in .
Antibiotic delivery systems on inorganic material core
Inorganic nanosystems represent a class of nanosystems derived from inorganic materials – metals, metaloxides, carbon materials, silica nanoparticles and quantum dotsCitation189,Citation190
Metallic NPs generally composed of Au, Ag, or Cu have been shown to possess strong antimicrobial activityCitation191. Furthermore, because of the small size of the NPs, they facilitate transport of active substances across biological barriers, and facilitate release of the drug in the proper site(s)Citation192. The use of metal NPs as drug carriers is one of the recent rapidly developing approaches in chemotherapyCitation193. Metal NPs as drug carriers appear beneficial due to their ability to solve problems of solubility of hydrophobic drugs, and increase the dissolution efficiency of drug molecules in the bloodCitation194. Because of considerable interactions between the particles and the medium, agglomeration may occur. Moreover, metallic NPs on their own may induce harmful effects, in particular to healthy cellsCitation194. Antimicrobial activities of metals and metaloxides have been widely studied. Highly potent antibacterial effects have been shown by a number of metaloxide NPs, which include silver (Ag), iron oxide (Fe3O4), titanium oxide (TiO2), copper oxide (CuO), and zinc oxide (ZnO). Most metaloxide NPs exhibit bactericidal properties through generation of reactive oxygen species (ROS), while some are effective due to their physical structure and release of metal ionsCitation195.
Silver nanoparticles
Silver exhibits favourable effects because of its chemical stability, excellent conductivity, catalytic, and antibacterial activity. Nowadays, silver nanoparticles (AgNPs) are among of the most widely studiedCitation196,Citation197. It is known that AgNPs are effective antimicrobial agents against bacteria, fungi, and virusesCitation198,Citation199. In medicinal use, Ag-containing compounds are found useful in treatment of burns, wounds, and various infectious diseasesCitation200,Citation201. The antimicrobial potency of AgNPs, like other metals or metaloxide NPs, has been foundCitation202. Despite the fact that the mechanism of action for AgNPs remain unclear, AgNPs with small diameters have been found to possess higher antimicrobial potency than those with larger diametersCitation203. Silver is also known as an efficient bactericidal agent that can kill various pathogens in vitro and in vivoCitation204. Furthermore, bacteria appears to have a lower propensity for development of resistance against Ag than seen for ordinary antibioticsCitation205–207.
Nevertheless, there is numerous aspects that remain to be addressed: silver minimal inhibitory concentration (MIC), minimum bactericidal concentration (MBC) clinical breakpoint, effects on emergence of resistanceCitation208,Citation209, nanoparticle toxicity, and side effects of AgNPsCitation210,Citation211. Importantly, the details in the bactericidal mechanism of AgNPs remains to be elucidatedCitation212.
Usually, particles with diameters below 100 nm are suitable for drug delivery applications, and AgNPs that meet this requirement are readily obtained. Coating of NPs with active substances, may considerably increase the size of the resulting modified particles, and therefore it is crucial that the core itself is small. The accumulation of AgNPs at disease site depends on a facilitated translocation across membranes, which is better for small-size AgNPs. However, smaller particles may increase intracellular cytotoxicity caused by their degradation. For neutral AgNPs cytotoxicity should be lower than for drug-loaded particles. The attractive force among the conducting electrons of AgNPs and bacterial cell membranes facilitate their adhesion to the surface, thereby launching a toxic effect. AgNPs, having a diameter less than 50 nm, change the potential of bacterial cells quite efficiently and prevent their proliferationCitation213. AgNPs also demonstrate synergistic antibacterial effects in combination with different antibiotics on both Gram-positive and Gram-negative bacteriaCitation214,Citation215.
AgNPs are effective antimicrobials, and constitute promising alternatives to traditional antibiotics. The evaluation of potency of differently coated 10 and 50 nm-sized AgNPs against Gram-negative E. coli and Gram-positive S. aureus demonstrated that 10 nm-sized AgNPs released more Ag ions and were more toxic than 50 nm-sized AgNPs, while coating-dependent activity was more prominent for 50 nm-sized AgNPsCitation216. Recently, novel silver ultra-nano clusters were synthesised with an average size less than 5 nmCitation217. These clusters were effective in eradicating H. pylori biofilms and showed only a low toxicity on human gastric adenocarcinoma cells.
The exact mechanism of action of AgNPs is still not clear. Mechanistically, the antimicrobial profile of AgNPs is correlated to their attraction to bacterial surface, destabilisation of bacterial cell wall, change in membrane permeability, induction of toxicity and oxidative stress by generation of reactive oxygen species (ROS) and free radicals as well as modulation of signal transduction pathwaysCitation218,Citation219.
Several new silver‐based antimicrobial products have been commercialised in the last two decadesCitation220 and a recent study investigated surgical sutures coated with titanium dioxide nanoparticles covalently linked with monovalent silver ions for its potential prophylactic activity against surgical site infectionsCitation221.
Gold nanoparticles
Gold nanoparticles (AuNPs) are colloidal or clustered particles comprised of an inert and biocompatible gold coreCitation222. One of the advantages of AuNPs is their synthetic versatility allowing for control of their size, shape and surface properties. To enable conjugation of biologically active molecules the NP surface can be modified with thiols or amines, thereby providing functionalised AuNPsCitation223. Despite the potential applicability of AuNPs, some uncertainty remains about their potential toxicityCitation219. AuNPs are active against Gram-negative and Gram-positive bacteria, namely E. coli, P. aeruginosa, S. typhimurium, Serratia spp., K. pneumoniae, S. aureus, B. subtilis and E. faecalis, among othersCitation224–226 In comparison to Ag, AuNPs show lower antibacterial effect by themselvesCitation212. The adsorption of active drug molecules on the AuNPs surface allows for delivery of active ingredients to targeted sites. Various antimicrobial agents such as antibiotics, antimicrobial peptides, and surfactants can be conjugated onto Au shells to confer bactericidal activityCitation227. Regardless of the scope of application of AuNPs, it is very important to maintain their stability by preventing their aggregationCitation194.
Copper and copper oxide nanoparticles
Copper is a semiconductor material considered to be an excellent candidate for the synthesis of copper based NPs (CuNPs). Along with being highly resistant to heat, it is robust, stable, cheap, and can be readily obtainedCitation228,Citation229. Although copper oxide (Cu2ONPs and CuONPs) are effective against various bacterial pathogens, their antibacterial efficacy is somewhat lower than that of Ag or ZnO (see below). Thus, a relatively higher concentration of NPs is needed to achieve the same level of activityCitation230. In addition, the activity CuONPs varies considerably depending on which bacterial species is to be targeted. Nevertheless, as Cu is less costly than other metal nanomaterials, it can be used as nanocomposite to enhance the efficacy of antibiotics.
CuONPs also possess antimicrobial effects in themselves. The mechanism of antibacterial activity of CuONPs is as yet not well clarified, but it is assumed that it involves bacterial cell wall adhesion caused by electrostatic interactions. Released Cu2+ions induces generation of ROS that interact with cellular membranes. The ROS is also capable of penetrating the cell, causing bacterial cell membrane damage, which is associated with degradation of the internal contents and bacterial cell leakageCitation231. Moreover, their antibacterial activity has been studied in detail against microorganisms such as E. coli, V. cholera, P. aeruginosa, S. typhus, S. aureus, E. faecalis, B. subtilis and S. faecalisCitation219,Citation232,Citation233.
Zinc oxide nanoparticles
Due to photocatalytic properties of zinc oxide nanoparticles (ZnONP) shave the ability to generate ROS quite readily, which gives rise to favourable antibacterial properties. ZnONPs have a positive surface charge, and therefore they will readily interact with negatively charged bacterial membranes, which facilitate penetration into the cells, leading to their destruction. These features allow them to be applied as excellent additives for dentistry bactericides and daily cosmetics products. ZnONPs have an isoelectric point at pH ∼9. At pH values below 9, ZnONPs are surface-protonated to the form of ZnOH2+, which means that they remain positively charged in the pH range corresponding to body fluids, which promotes their applicability as drug carriersCitation234. Photoluminescent features, used in biosensing, together with antibacterial properties, are promising parameters for the putative use of these NPs in theranostics. Use of ZnO in self-lighting photodynamic therapy (SLPDT) against human ovarian cancer (OVCAR-3) cells in culture was described a decade ago. It was proven that under dark conditions, the ZnONPs conjugated with methylthioadenosine phosphorylase (MTAP) anticancer drug to safely reach its intended site, and when exposed to ultraviolet A (UVA) radiation they were able to produce ROS, which significantly reduced the viability of cancer cells. Cells died both by apoptosis and necrosisCitation235. Those studies produced a clear evidence that use of ZnONPs in combination with already known anticancer drugs may enhance the effect of cancer therapyCitation194.
Titanic oxide nanoparticles
Also, titanium dioxide (TiO2) has been extensively studied for its antimicrobial activitiesCitation236. TiO2 is known for its ability to kill both Gram-positive and Gram-negative bacteriaCitation237. More recently it was found that titanic oxide nanoparticles (TiO2NPs) were effective against various viral species and parasitesCitation195,Citation238.
TiO2 are characterised by a refractive index in the UV/visible range. TiO2, like ZnO, is an n-type semiconductor materialCitation239. TiO2NPs are relatively inexpensive, highly chemically stable, non-toxic, biocompatible, and also highly active. Depending on the parameters and the method used, it is possible to control the shape and size of the particles. A number of methods are currently available for the preparation of TiO2NPs. Among them, the most used are sol-gel, hydro, and solvothermal methods along with electrochemical methodsCitation240. The controlled release of daunorubicin (DNR) was observed when using TiO2 NPs as a drug carrier. This release was observed due to the possibility to change pH. It was noted that lowering the pH from 7.4 to 5.0 accelerated the release of DNR from TiO2NPsCitation241.
The combinations of Ti or TiO2 with other NPs such as AgNPs were found to have a synergistic effect and to enhance their activityCitation242–245.
Superparamagnetic iron oxide nanoparticles
Iron oxide NPs have found wide applications in different fields of biomedicineCitation246. The antibacterial properties are described both for NPs based on iron oxides and as free iron ions. However, in contrast to free ions, iron oxides do not exert a significant toxic effect on mammalian cellsCitation247,Citation248. Among iron oxide NPs, superparamagnetic iron oxide nanoparticles (SPIONs) consisting of Fe2O3 or Fe3O4 being the most important ones. They are mainly used as contrast agents in imaging (MRI), but they have also found applications as targeted drug carriers and biosensors. Toxicity studies of SPIONs show that they may be mutagenic and neurotoxic, can induce genotoxicity, and also cause adverse effects when subjected to long-term exposureCitation194,Citation239. Microbiological assays have proven that surfaces modified by Fe3O4 nanoparticles demonstrate anti-adherent properties, and therefore significantly reduce both Gram-negative and Gram-positive bacterial colonisationCitation195,Citation249. Comparative studies of the antibacterial properties of ferrimagnetic and superparamagnetic NPs led to the conclusion that both nanomaterials may be considered as antibacterial candidates for treatment of complicated infections in the upper respiratory tract or in the skinCitation250. SPIONs can be coated with other NPs such as Ag and Au, and their magnetic properties can be utilised to penetrate and destroy biofilmsCitation251,Citation252 or to enable surface modification with other materials, for example oleic acid, alginate or polyethylene glycol to prevent P. aeruginosa biofilm formationCitation253. It has been suggested that the capping agent plays a major role in conferring bactericidal properties to such nanocomposites. Alternatively, several surface modifications of SPIONs were used for optimisation of the activity and increasing their interaction with the bacterial cellsCitation254. These NPs can be modified with chemical groups and metals can be adhered to them. As the example, synthesis of silver-ring-coated SPIONs involves the coating of monodispersed SPIONs with carboxylated dextran via the ligand exchange followed by conjugation with ethanediylbis(isonicotinate)Citation255. Also conventional immobilisation of amphiphilic 1,4-dihydropyridine (1,4-DHP) on aminosilane-coated magnetic NPs markedly enhances their antimicrobial activity as compared to non-immobilised molecules due to the higher affinity of these nanosystems for bacterial cell wall componentsCitation256. Good combination of the antimicrobial activity and biocompatibility of iron nanoparticles makes them particularly attractive study objects as antibiotic carriersCitation257.
Carbon-based nanomaterials
During the past years carbon-based NPs have been used for antiviral applications. Usually, carbon nanomaterials exert low cytotoxicity and exhibit specific antiviral activities. Carbon-based nanomaterials have yielded encouraging results and may provide the necessary level of biocompatibility and antiviral properties. The main groups of carbon nanomaterials explored as antiviral systems comprise e.g. fullerenes, carbon nanotubes (CNTs), and in particular single-walled carbon nanotubes (SWCNTs) as well as graphene oxide (GO) NPsCitation258. Due to a high surface/volume ratio and other unique chemical and physical properties of carbon-based nanostructures the study of their antimicrobial properties is particularly interesting. Furthermore, application of functionalised carbon nanomaterials as delivery systems of antibiotics may reduce the associated resistance, improve bioavailability and facilitate targeted deliveryCitation259. The size of carbon NPs is of importance for the inactivation of the microorganisms. Thus, shorter tubes demonstrate more effective bactericidal performance in comparison to longer tubes. CNT-cell interactions in a liquid medium are rather different if compared to the solid surface. Tube diameter is also significant for the bacterial inactivation process. Tubes with smaller diameters can provide higher damage to cell membrane though the cell-surface interactionCitation260,Citation261. Direct contact with microorganisms is their main mechanism of action, which involves interference with their cellular membrane integrity, metabolic processes and morphologyCitation258,Citation259.
Structural features such as type of core and surface functionalities of carbon dots (CDs) have a crucial influence on their interaction with other cells and organisms, and this can be used in the design of probes to target specific bacterial classes. Due to their fluorescent properties CDs are used to label and image bacteria. CDs have also found applications as photosensitisers, which provide excellent spatiotemporal control upon irradiation with light of the production of ROS leading to membrane damage. Nanocomposite CDs containing antimicrobial agents including antibiotics and transition metals are effective theranostic agents displaying synergistically improved antimicrobial activities. Incorporation of CDs in hydrogels, nanofibrous materials, and polymers is one of the ways for improvement of materials for application in wound healing and regeneration, and also of materials with a long-lasting protection against infectionsCitation262.
Silica nanoparticles
Mesoporous nanostructures are attractive building blocks due to their biomolecule adsorption/desorption capabilitiesCitation263,Citation264. Mesoporous silica-based nanoparticles (MSiNPs) offer advantages in nanotechnology because of their applicability in the design of complex systems and their cost-effectiveness. Their specific surface characteristics, porosity and possibilities for functionalization make them useful in therapeutic deliveryCitation265. MSiNPs constitute a material, which combine an efficient drug delivery profile and facile (bio)chemical modificationCitation266–268. The antibacterial effect of silicon-based nanocoatings was demonstrated on Gram-positive (S. aureus) and Gram-negative (P. aeruginosa) bacteria by using them in the form of biofilm-durable polymeric matricesCitation269. The modification of particles with antibiotics was one of the first nanotechnological strategies explored to combat bacterial infectionsCitation268. Various antimicrobials such as antibiotics, peptides, and other functional materials can be covalently or non-covalently loaded onto MSiNPs, offering a rational solution to the issues of resistance, physiological barriers and functional barriers such as drug solubility or toxicityCitation270.
Quantum dots
Quantum Dots (QD) are a type of fluorescent nanomaterial developed recently. QDs are tiny particles or nanocrystals of a semiconducting material with diameters within the range 2–10 nm. QDs are semiconductor nanocrystals with peculiar photoluminescence properties. Use of QDs for biological imaging is one of the most successful new nanobiotechnology developmentsCitation260. These particles are comprised of a semiconductor inorganic core such as CdSe and coated shell such as ZnS improving optical properties and solubility in aqueous buffersCitation271. Polymer-functionalised QDs possessing more promising features and higher antibacterial activity have been reportedCitation272. Functionalised QDs could be applied in antibacterial research as an effective alternative to traditional antibiotic drugs due to their exceptional antibacterial mechanisms. Functionalised QDs can insert into the cell membrane, thereby destroying the cell barrier and produce a large amount of ROS, thereby damaging the cell componentsCitation273. Compared with other functionalised NPs, QDs with quantum size are generally more effective against microorganisms than metal-based NPs with sizes ranging within 30–100 nmCitation274. Additionally it has been demonstrated that heavy metal-free QDs selectively eliminate multidrug-resistant (MDR) pathogenic bacteria, while remaining non-toxic to human host cellsCitation275. CdTe-QD are non-toxic at concentrations relevant for reducing the bacterial load of MDR E. coli infections in a murine subcutaneous abscess model. These QDs destroy bacteria through the generation of superoxideCitation276.
Antibiotic delivery systems based on cores of organic materials
Organic nanosystems-liposomes, lipid-based NPs, polymeric NPs have preferable biodegradability and biocompatibility features, making them suitable as candidates for clinical useCitation190,Citation277.
Lipid-based nanoformulations
Lipid-based nanoformulations such as liposomes, nanoemulsions, and solid lipid NPs (SLNs), are often employed as delivery systems for antibacterial drugs. Liposomes can easily bind to bacterial membrane, and deliver antibiotics directly to bacteriaCitation278.
Liposomes are concentric, bilayered vesicles formed by a membranous lipid bilayer, which mainly is composed of natural or synthetic lipids. Liposome diameters are within the size range of 0.01–5.0 µm, and they can act as a carriers for a variety of drugsCitation279,Citation280. The antibacterial activity and the pharmacokinetics properties of antibiotics can be enhanced by entrapping them within liposomes. Use of lipid vesicles as drug carriers may influence drug distribution and reduce toxic effectsCitation281. Liposomes are usually non-toxic, biodegradable and can encapsulate both hydrophobic and hydrophilic drugs without any chemical modification. In addition, liposomes can be composed of materials possessing distinctive desirable properties such as long systemic circulation time, specific cell targeting, and release that is sensitive to pH, reductive environment and/or temperatureCitation282. Another outstanding feature of liposomes is their ability to fuse easily with the bacterial membranes, thereby releasing the drug within the cell membrane, or into the interior of the microorganismCitation283–285. As a result, the effective dose of the drug and its toxicity decreasesCitation286. Additionally liposomes protect the entrapped drug against enzymatic degradation before reaching its target. For example, liposomes protect penicillins and cephalosporins from degradation by β-lactamases, which are produced by certain microorganismsCitation287,Citation288. Recently, several reviews on antibiotic encapsulation in liposomes, comprised of various lipids, have been publishedCitation289–292.
During the last years attention has been dedicated to the development of stimuli-responsive nanocarriers, such us pH-, enzyme-, redox-, and ionic-microenvironment-dependent antibacterial therapyCitation293–295. It was also found out, that liposomes may be designed to fuse with bacterial cells, holding the potential to overcome antimicrobial resistance and biofilm formation and constituting a promising solution for the treatment of potential fatal MDR bacterial infections e.g. caused by methicillin-resistant S. aureusCitation278,Citation292,Citation296.
Another way to improve the activity of liposomal antibiotic system is to promote the synergy between drug and lipid activity. It has been found that various natural and synthetic lipids used for liposome preparation also possess inherent antibacterial activity. For example, it was demonstrated that selected fatty acids and cholesteryl esters packaged with phospholipids possessed antibacterial activity against Gram-positive and Gram-negative bacteria, and thus may augment the activity of antibiotics demonstrating a potential as novel lipophilic antimicrobial agentsCitation297. The toxicity of the 4-(N-alkylpyridinium)-1,4-DHP derivatives on both Gram-positive and Gram-negative bacteria species and eukaryotic microorganisms has been studied, and the results showed that activity depended on the alkyl chain length at the N-alkyl pyridinium moiety and the number of propargyl groups. These lipid-like compounds warrant further exploration as constituents of drug formulationsCitation298. Also sulfonium-based antibacterial lipids for the delivery of antibiotics have been recently developed. The presence of cationic sulfonium moieties and the inherent membrane-targeting properties of the lipids contributed to the reduction of antibiotic resistance development in bacteria, and also enabled delivery of antibiotics to remove the infectious pathogens selectively. Sulfonium-based liposome-encapsulated antibiotics is an example of synergy between drug and lipidCitation299.
Solid lipid nanoparticles (SLNs) are the most effective lipid-based colloidal delivery systems, introduced in the end of the 20th century. In contrast to liposomes, SLNs do not have a two-layer structure and are composed of a solid fat matrix and stabilised by surfactants. The main benefits of SLNs are their long-term stability, ease of use, encapsulation of hydrophilic and lipophilic drugs, and biocompatibilityCitation300. SLNs are within the size range of 50–1000 nm, and they are composed of physiologically tolerated lipid components, which are in the solid state at room temperatureCitation301. For preparation of SLNs solid lipids such as stearic acid, glyceryl monostearate, glyceryl distearate, and decanoic acid as well as surfactants such as soy lecithin, egg lecithin, sodium cholate, sodium glycocholate, tween 20 and 80, or polaxamers 188, 182, or 407 are usedCitation302. Proposed advantages of SLNs include the possibility of controlled drug release, high drug payload, excellent bioavailability, avoidance of the use of organic solvent, no toxicity of the carrier, easy upscaling and sterilisation, increased drug stability, and possibility for incorporation of lipophilic and hydrophilic drugsCitation303.
Polymeric nanoparticles
Some polymers also exhibit antimicrobial properties in themselves. Such antimicrobial polymeric materials can be employed for biomedical devices and in healthcare to avoid the resistance problems associated with use of antibioticsCitation304. Polymeric particles produced from natural and/or synthetic polymers are biocompatibile and often multifunctional, and they may also possess high stability in vitro and in vivo. The polymeric particles allow for controlled and sustained release of antibiotics in order to improve antibacterial efficacy against biofilm-related infectious diseasesCitation305. The commonly used natural polymers are human or bovine serum albumin, gelatine, collagen, alginate, chitosan, and starch. Use of synthetic polymers such as poly (α-hydroxy acids), polyanhydrides, polylactide, poly(lactide-co-glycolide) (PLGA), and poly(fatty acid dimer-sebacic acid) has been reported alsoCitation306.
Dendrimers are hyperbranched polymers with a precise nanoarchitecture and low polydispersity, which are synthesised in a layer-by-layer fashion around a core unit, resulting in a high-level control of size, branching points, and surface functionality. The highly dense surface of functional groups allow for the synthesis of dendrimers with specific and high binding affinities to a wide variety of viral and bacterial receptorsCitation280.
The encapsulation of antibiotics in dendrimeric systems can improve their therapeutic efficacy and minimise their side effects. A primary point in the design of dendrimers as delivery systems are the control of particle size, the properties of the surface, the functionality and branch length/density, and the proper release of drugs to obtain the desired effect on the targeted siteCitation307. Drugs, including antibiotics, can be trapped inside dendrimers, be physically adsorbed onto their surface or chemically attached to the dendrimer surface. Encapsulation of antibiotics in dendrimeric materials is a tool for an improvement of their pharmacokinetic and pharmacodynamic propertiesCitation308. Both hydrophilic and hydrophobic agents can be loaded at the same time either by encapsulating drug within the dendrimer structure, or by interacting with the drugs at their terminal groups via electrostatic attacation, or via covalent linkage to suitable functional groupsCitation187.
One of the most studied dendrimers for the release of antibacterial drugs is the PAMAM dendrimer. PAMAM is a non-immunogenic and biocompatible compound that is water-solubleCitation309. PAMAM materials are regarded as carriers or drugs (e.g. antibacterials or antifungals), which have a capacity to improve solubility, drug permeation, and therapeutic efficiencyCitation309. Based on the type of linkage and chemical moieties presented in the molecule, dendrimers can be divided into four types: glycol dendrimersCitation310, peptide dendrimersCitation311, and Janus dendrimersCitation312,Citation313.
Chitosan is obtained by deacylation of the natural polymer chitin. Properties such as biocompatibility, biodegradability, and also inherent broad-spectrum antimicrobial properties make chitosan suitable as a carrier for antibiofilm agentsCitation314. Chitosan NPs were found to be effective against 24-hold P. aeruginosa biofilms of six different clinical isolatesCitation315.
The surface charge of chitosan NPs is positive, allowing them to interact with negatively charged bacterial cell walls and biofilm-derived extracellular polymeric substance (EPS) surfacesCitation316. Chitosan derivatives possess the antibacterial activity, which depends on the type of microorganism, the Mw or degree of deacylation of chitosan, the concentration of chitosan, pH value of media and temperatureCitation317. On one hand biocompatibility, degradability, and antimicrobial nature of chitosan constitute the features, which make it suitable for antibiotic delivery into biofilms, on the other hand the hydrophobicity of chitosan promotes aggregation under biological condition, and hence limits its use. Aggregation of chitosan NPs could be observed after an hour in cell mediumCitation318. The addition of poly(ethylene glycol) (PEG) to the surface of chitosan NPs proved to be an efficient way to overcome this obstracleCitation319. NPs of chitosan modified with linoleic acid were used for immobilisation of the enzyme β-N-acetylglucosaminidase (DspB), which then was found to be more effective as compared to the free enzyme with respect to inhibition and detachment of biofilms via degradation of poly-β(1,6)-N-acetyl-glucosamine (PNAG), a major polysaccharide found in the EPS produced by S. epidermidis, S. aureus, and Actinobacillus actinomycetemcomitansCitation320.
Alginate is biopolymer derived from the cell wall of algae, and it is commonly used for construction of nanocarriers for drug delivery. Alginate is a naturally occurring polysaccharide, which unlike chitosan, is an anionic polymer. Alginate-based NPs can be loaded with antimicrobial agents for treatment of tuberculosis or fungal infectionsCitation321. Usage of alginate has several advantages including ease of preparation, biocompatibility, biodegradability, and absence of toxicityCitation322. Alginate-based beads were reported to be useful for sustaining the release of poorly water-soluble flavonesCitation323.
Poly(lactic-co-glycolic acid) nanoparticles (PLGA NPs). Poly(lactic-co-glycolic acid) (PLGA) is a biocompatible and degradable copolymer. The ester linkages in PLGA are degraded through hydrolysis in the presence of water, and the polymer degradation products lactic acid and glycolic acid are natural byproducts of several metabolic pathways. Various drug delivery devices made of PLGA NPs are FDA approvedCitation316. Generally, the size of PLGA NPs is within the range 100–400 nmCitation324. PLGA NPs provide robust drug delivery systems. Most PLGA-encapsulated antibiotics show a biphasic sustained release profile in vitro. With the surface decoration of PLGA NPs with PEG, the biocompatibility of PLGA NPs can further be improved, and the circulation time elongated when the application necessitates this. The main disadvantage of PLGA NPs is low encapsulation efficiency, especially of hydrophilic agentsCitation316.
Poly-ε-lysine. Poly-ε-lysine is a cationic homo-oligopeptide of L-lysine found to be active against Gram-positive and Gram-negative bacteria. It also exhibits activity against the spore forms of B. coagulans, B. stearothermophilus and B. subtilisCitation195,Citation325.
Other polymeric particles are also successfully used instead of PLGA NPs. For example, dental resin composites with quaternary ammonium polyethylenimine (QPEI) NPs demonstrated antimicrobial activity against intraoral biofilmCitation326, while poly(L-lactic acid) (PLLA) NPs modified by Ag particles was capable of inhibiting S. epidermidis biofilm formationCitation327. Levofloxacin encapsulated into poly(caprolactone) nanoparticles (PCL NPs) was used as a component in treatment of lung infectionsCitation328.
Hybrid nanoparticles
Recent progress in the field of hybrid NPs has provided new opportunities for the development of a wide range of antibacterial functional materials. For example, hybrid nanosystems such as AgNPs dispersed inside a functionalised novolac resin matrix, combining properties of both components, offer antibacterial activity towards Gram-positive and Gram-negative bacteriaCitation329. Lipid-coated hybrid nanoparticles (LCHNPs) are the next-generation core–shell structured nanodelivery systems, in which an inorganic or organic core, loaded with antimicrobials, is coated with lipid layers. This core–shell structure with diverse coatings may improve the capacity for loading of therapeutics, and also has the potential to improve therapeutic delivery, especially for targeting biofilm-associated and intracellular infectionsCitation330. So, to improve the efficiency of antibiotic delivery and targeting, Yang et al. reported a unique gentamicin-loaded mesoporous silica NPs coated with bacteria toxin-responsive lipid bilayers that is decorated with the bacteria-targeting peptide, UBICitation331.
The AgNPε-polylysine nanocomposite demonstrated antibacterial effects against P. aeruginosa and S. aureus speciesCitation332. Also hybrid NPs, formed from a PLGA nanoparticulate core with a lipid shell composed of DOTAP and DSPE-PEG5K, demonstrated biofilm-penetrating properties. Such NPs with dimensions of 100–130 nm showed a superior binding affinity to several bacterial species, including both Gram-positive and -negative pathogens. These hybrid NPs were capable often capsulating various hydrophobic antibiotics with relatively high loading efficiencies, and they gave rise to a significantly increased biofilm inhibitionCitation332.
Composite devices in which antibiotic-loaded silica NPs were embedded within collagen hydrogels have been described. These resulted in a delivery system that was capable of preventing bacterial infections due to a sustained delivery of antibiotic (gentamicin or rifampicin), while the outer collagen layer promoted the healing of chronic woundsCitation333.
Among various nanocarriers, vesicles such as liposomes and polymersomes are considered to be promising alternatives for delivery of hydrophilic and lipophilic drugs. They have different classifications according to their composition, among which hybrid vesicles, unlike liposomes, are composed of both lipids and polymers. These vesicular systems stand out since they combine the advantages of both components, overcoming the limitations of traditional delivery systems arising from low stability and premature release of the encapsulated active substanceCitation334.
Conclusion and prospects
Even though there are various strategies utilised by pharmaceutical companies and research groups worldwide to develop new antibacterial drugs, we have highlighted in our opinion one of the most novel and promising ones.
Since the discovery of penicillin natural products still remain one of very important sources for discovery of novel antibacterial agents being natural products or synthetic derivatives. Therefore, taking into account that pull of natural compounds is far from being fully investigated, natural products remain and most probable will remain of one of the sources of inspiration for the development of new antibacterial drugs in next decades.
As one of important aspects of the discovery of novel antibacterial agents is the identification of new drug targets. During last decade great attention was dedicated to bacterial metalloenzymes and carbonic anhydrases in particular. Even though these enzymes are ubiquitous in nature’s kingdom, bacterial carbonic anhydrases usually belong to different family compared to human ones. This in turn allows the development of novel inhibitors which targets bacterial metalloenzymes and will not interfere with human carbonic anhydrases. Additionally, carbonic anhydrases along different bacteria also differ, and that allows selective targeting of pathogenic bacteria and allows not killing friendly bacteria, for instance, in microbiota. Considering these facts bacterial carbonic anhydrases will be in focus for the development of novel antibacterial agents for the next decades.
Biopharmaceuticals and antisense peptide nucleic acids as novel antibacterials have gained attention in recent years. These types of compounds reduce the viability of pathogenic bacteria by selective binding to bacterial RNA, thus allowing targeting specific bacteria only. Even though there is still a lot of research to be performed, the development of this type of biopharmaceuticals is very intriguing in will take the attention of many scientists in nearest future.
Biofilm formation often is involved in bacterial infectious diseases. Biofilm formation is based on the bacterial communication system or quorum sensing. An interruption or inhibition of this communication will not only lead to treatment of disease and reducing of pathogenic bacteria, but also to the reduction of formation of bacterial resistance. This bacterial control system, where pathogenic bacteria are not directly targeted, but instead its communication is interrupted is one of the rising and probably also one of the most important strategies for bacterial control.
And last but not least antibiotic drug delivery nanosystems play a great role in the application of know antibiotics as well as novel molecules. Such nanosystems not only solve problems with drug delivery to and in pathogenic bacteria thus allowing more efficient treatment of bacterial infectious diseases which was not possible in the past, but also may solve the problem with drug availability in less developed regions of our planet, where instead of injection drug nanofurmalates might be used for slow and regular drug release.
Taking all together this review covers in our opinion one of the most aspects of the discovery of novel antibacterials.
Disclosure statement
No potential conflict of interest was reported by the author(s) except CTS. CT Supuran is Editor-in-Chief of the Journal of Enzyme Inhibition and Medicinal Chemistry. He was not involved in the assessment, peer review, or decision-making process of this paper. The authors have no relevant affiliations of financial involvement with any organisation or entity with a financial interest in or financial conflict with the subject matter or materials discussed in the manuscript. This includes employment, consultancies, honoraria, stock ownership or options, expert testimony, grants or patents received or pending, or royalties.
Additional information
Funding
References
- Newman DJ, Cragg GM. Natural products as sources of new drugs over the nearly four decades from 01/1981 to 09/2019. J Nat Prod. 2020;83(3):770–803.
- Patridge E, Gareiss P, Kinch MS, Hoyer D. An analysis of FDA-approved drugs: natural products and their derivatives. Drug Discov Today. 2016;21(2):204–207.
- Karageorgis G, Foley DJ, Laraia L, Brakmann S, Waldmann H. Pseudo natural products—chemical evolution of natural product structure. Angew Chem Int Ed Engl. 2021;60(29):15705–15723.
- Jukes TH. Some historical notes on chlortetracycline. Rev Infect Dis. 1985;7(5):702–707.
- Grossman TH. Tetracycline antibiotics and resistance. Cold Spring Harb Perspect Med. 2016;6(4):a025387.
- Ronn M, Zhu Z, Hogan PC, Zhang W-Y, Niu J, Katz CE, Dunwoody N, Gilicky O, Deng Y, Hunt DK, et al. Process R&D of eravacycline: the first fully synthetic fluorocycline in clinical development. Org Process Res Dev. 2013;17(5):838–845.
- Nordmann P, Dortet L, Poirel L. Carbapenem resistance in Enterobacteriaceae: here is the storm!. Trends Mol Med. 2012;18(5):263–272.
- Hecker SJ, Reddy KR, Totrov M, Hirst GC, Lomovskaya O, Griffith DC, King P, Tsivkovski R, Sun D, Sabet M, et al. Discovery of a cyclic boronic acid β-lactamase inhibitor (RPX7009) with utility vs. class a serine carbapenemases. J Med Chem. 2015;58(9):3682–3692.
- Kahan JS, Kahan FM, Goegelman R, Currie SA, Jackson M, Stapley EO, Miller TW, Miller AK, Hendlin D, Mochales S, et al. Thienamycin, a new beta-lactam antibiotic. I. Discovery, taxonomy, isolation and physical properties. J Antibiot (Tokyo)). 1979;32(1):1–12.
- Iso Y, Irie T, Nishino Y, Motokawa K, Nishitani Y. A novel 1β-methylcarbapenem antibiotic, S-4661 synthesis and structure-activity relationships of 2-(5-substituted pyrrolidin-3-ylthio)-1β-methylcarbapenems. J Antibiot. 1996;49(2):199–209.
- Nishino Y, Kobayashi M, Shinno T, Izumi K, Yonezawa H, Masui Y, Takahira M. Practical large-scale synthesis of doripenem: a novel 1β- methylcarbapenem antibiotic. Org Process Res Dev. 2003;7(6):846–850.
- Sipponen A, Rautio M, Jokinen J, Laakso T, Saranpaa P, Lohi J. Resin-salve from norway spruce – a potential method to treat infected chronic skin ulcers? Drug Metab Lett. 2007;1(2):143–145.
- Manner S, Vahermo M, Skogman ME, Krogerus S, Vuorela PM, Yli-Kauhaluoma J, Fallarero A, Moreira VM. New derivatives of dehydroabietic acid target planktonic and biofilm bacteria in Staphylococcus aureus and effectively disrupt bacterial membrane integrity. Eur J Med Chem. 2015;102:68–79.
- Hassan G, Forsman N, Wan X, Keurulainen L, Bimbo LM, Johansson L-S, Sipari N, Yli-Kauhaluoma J, Zimmermann R, Stehl S, et al. Dehydroabietylamine-based cellulose nanofibril films: a new class of sustainable biomaterials for highly efficient, broad-spectrum antimicrobial effects. ACS Sustainable Chem Eng. 2019;7(5):5002–5009.
- Supuran CT, Winum JY. Introduction to zinc enzymes as drug targets. In: Supuran CT, Winum JY, editors. Drug design of zinc-enzyme inhibitors: functional, structural, and disease applications. Hoboken: Wiley; 2009. p. 3–12.
- Chen AY, Adamek RN, Dick BL, Credille CV, Morrison CN, Cohen SM. Targeting metalloenzymes for therapeutic intervention. Chem Rev. 2019;119(2):1323–1455.
- Capasso, C, Supuran, CT, Bacterial carbonic anhydrases. In: Supuran CT, Capasso C, editors. Zinc enzyme inhibitors. Topics in medicinal chemistry. Cham: Springer. Vol. 22; 2016. p. 135–152. DOI: 10.1007/7355_2016_12
- Capasso C, Supuran CT. Developing novel bacterial targets: carbonic anhydrases as antibacterial drug targets. In: Phoenix DA, Harris F, Dennison SR, editors. Novel antimicrobial agents and strategies. Heidelberg: Wiley-Springer; 2015. p. 31–46.
- Supuran CT. Bacterial zinc proteases as orphan targets. In: Supuran CT, Winum JY, editors. Drug design of zinc-enzyme inhibitors: functional, structural, and disease applications. Hoboken: Wiley; 2009. p. 675–704.
- Mastrolorenzo A, Supuran CT. Botulinum toxin, tetanus toxin and anthrax lethal factor inhibitors. In: Supuran CT, Winum JY, editors. Drug design of zinc-enzyme inhibitors: functional, structural, and disease applications. Hoboken: Wiley; 2009. p. 705–720.
- Supuran CT. Clostridium histolyticum collagenase inhibitors in the drug design. In: Supuran CT, Winum JY, editors. Drug design of zinc-enzyme inhibitors: functional, structural, and disease applications. Hoboken; Wiley; 2009. p. 721–730.
- Smith KS, Jakubzick C, Whittam TS, Ferry JG. Carbonic anhydrase is an ancient enzyme widespread in prokaryotes. Proc Natl Acad Sci USA. 1999;96(26):15184–15189.
- Capasso C, Supuran CT. An overview of the alpha-, beta- and gamma-carbonic anhydrases from Bacteria: can bacterial carbonic anhydrases shed new light on evolution of bacteria? J Enzyme Inhib Med Chem. 2015;30(2):325–332.
- Supuran CT. Carbonic anhydrases: Novel therapeutic applications for inhibitors and activators. Nat Rev Drug Discov. 2008;7(2):168–181.
- Supuran CT. Emerging role of carbonic anhydrase inhibitors. Clin Sci. 2021;135(10):1233–1249.
- Merlin C, Masters M, McAteer S, Coulson A. Why is carbonic anhydrase essential to Escherichia coli? J Bacteriol. 2003;185(21):6415–6424.
- Nishimori I, Minakuchi T, Vullo D, Scozzafava A, Innocenti A, Supuran CT. Carbonic anhydrase inhibitors. Cloning, characterization, and inhibition studies of a new β-carbonic anhydrase from Mycobacterium tuberculosis. J Med Chem. 2009;52(9):3116–3120.
- Supuran CT, Capasso C. Antibacterial carbonic anhydrase inhibitors: an update on the recent literature. Expert Opin Ther Pat. 2020;30(12):963–982.
- Supuran CT. Bacterial carbonic anhydrases as drug targets: toward novel antibiotics? Front Pharmacol. 2011;2:34–36.
- Campestre C, De Luca V, Carradori S, Grande R, Carginale V, Scaloni A, Supuran CT, Capasso C. Carbonic anhydrases: New perspectives on protein functional role and inhibition in Helicobacter pylori. Front Microbiol. 2021;12:629163–12.
- Mishra CB, Tiwari M, Supuran CT. Progress in the development of human carbonic anhydrase inhibitors and their pharmacological applications: where are we today? Med Res Rev. 2020;40(6):2485–2565.
- Supuran CT. Structure and function of carbonic anhydrases. Biochem J. 2016;473(14):2023–2032.
- Del Prete S, Nocentini A, Supuran CT, Capasso C. Bacterial ι-carbonic anhydrase: a new active class of carbonic anhydrase identified in the genome of the Gram-negative bacterium Burkholderia territorii. J Enzyme Inhib Med Chem. 2020;35(1):1060–1068.
- Del Prete S, Bua S, Supuran CT, Capasso C. Escherichia coli γ-carbonic anhydrase: characterisation and effects of simple aromatic/heterocyclic sulphonamide inhibitors. J Enzyme Inhib Med Chem. 2020;35(1):1545–1554.
- Supuran CT, Capasso C. Biomedical applications of prokaryotic carbonic anhydrases. Expert Opin Ther Pat. 2018;28(10):745–754.
- Rahman MM, Tikhomirova A, Modak JK, Hutton ML, Supuran CT, Roujeinikova A. Antibacterial activity of ethoxzolamide against Helicobacter pylori strains SS1 and 26695. Gut Pathog. 2020;12. DOI: 10.1186/s13099-020-00358-5
- Modak JK, Tikhomirova A, Gorrell RJ, Rahman MM, Kotsanas D, Korman TM, Garcia-Bustos J, Kwok T, Ferrero RL, Supuran CT, et al. Anti-Helicobacter pylori activity of ethoxzolamide. J Enzyme Inhib Med Chem. 2019;34(1):1660–1667.
- Modak JK, Liu YC, Supuran CT, Roujeinikova A. Structure-activity relationship for sulfonamide inhibition of Helicobacter pylori α-carbonic anhydrase. J Med Chem. 2016;59(24):11098–11109.
- Del Prete S, De Luca V, Bua S, Nocentini A, Carginale V, Supuran CT, Capasso C. The effect of substituted benzene-sulfonamides and clinically licensed drugs on the catalytic activity of CynT2, a carbonic anhydrase crucial for Escherichia coli life cycle. IJMS. 2020;21(11):4175.
- Aspatwar A, Kairys V, Rala S, et al. Kairys Rala Parikka Bozdag Carta Supuran Parkkila Mycobacterium tuberculosis β-carbonic anhydrases: novel targets for developing antituberculosis drugs. IJMS. 2019;20(20):5153.
- Wani TV, Bua S, Khude PS, Chowdhary AH, Supuran CT, Toraskar MP. Evaluation of sulphonamide derivatives acting as inhibitors of human carbonic anhydrase isoforms I, II and Mycobacterium tuberculosis β-class enzyme Rv3273. J Enzyme Inhib Med Chem. 2018;33(1):962–971.
- Aspatwar A, Hammarén M, Koskinen S, Luukinen B, Barker H, Carta F, Supuran CT, Parikka M, Parkkila S. β-CA-specific inhibitor dithiocarbamate Fc14–584B: a novel antimycobacterial agent with potential to treat drug-resistant tuberculosis. J Enzyme Inhib Med Chem. 2017;32(1):832–840.
- Carta F, Maresca A, Covarrubias AS, Mowbray SL, Jones TA, Supuran CT. Carbonic anhydrase inhibitors. Characterization and inhibition studies of the most active β-carbonic anhydrase from Mycobacterium tuberculosis, Rv3588c. Bioorg Med Chem Lett. 2009;19(23):6649–6654.
- Kaur J, Cao X, Abutaleb NS, Elkashif A, Graboski AL, Krabill AD, AbdelKhalek AH, An W, Bhardwaj A, Seleem MN, et al. Optimization of acetazolamide-based scaffold as potent inhibitors of vancomycin-resistant Enterococcus. J Med Chem. 2020;63(17):9540–9562.
- Abutaleb NS, Elkashif A, Flaherty DP, Seleem MN. In vivo antibacterial activity of acetazolamide. Antimicrob Agents Chemother. 2021;65(4):e01715-20.
- Abutaleb NS, Elhassanny AEM, Flaherty DP, Seleem MN. In vitro and in vivo activities of the carbonic anhydrase inhibitor, dorzolamide, against vancomycin-resistant Enterococci. PeerJ. 2021;9:e11059.
- Hewitt CS, Abutaleb NS, Elhassanny AEM, Nocentini A, Cao X, Amos DP, Youse MS, Holly KJ, Marapaka AK, An W, et al. Structure-activity relationship studies of acetazolamide-based carbonic anhydrase inhibitors with activity against Neisseria gonorrhoeae. ACS Infect Dis. 2021;7(7):1969–1984.
- Narenji H, Gholizadeh P, Aghazadeh M, Rezaee MA, Asgharzadeh M, Kafil HS. Peptide nucleic acids (PNAs): currently potential bactericidal agents. Biomed Pharmacother. 2017;93:580–588.
- Lee HT, Kim SK, Yoon JW. Antisense peptide nucleic acids as a potential anti-infective agent. J Microbiol. 2019;57(6):423–430.
- Wojciechowska M, Równicki M, Mieczkowski A, Miszkiewicz J, Trylska J. Antibacterial peptide nucleic acids—facts and perspectives. Molecules. 2020;25(3):559.
- Good L, Nielsen PE. Antisense inhibition of gene expression in bacteria by PNA targeted to mRNA. Nat Biotechnol. 1998;16(4):355–358.
- Good L, Awasthi SK, Dryselius R, Larsson O, Nielsen PE. Bactericidal antisense effects of peptide – PNA conjugates. Nat Biotechnol. 2001;19(4):360–364.
- Vaara M, Porro M. Group of peptides that act synergistically with hydrophobic antibiotics against gram-negative enteric bacteria. Antimicrob Agents Chemother. 1996;40(8):1801–1805.
- Ghosal A, Nielsen PE. Potent antibacterial antisense peptide-peptide nucleic acid conjugates against Pseudomonas aeruginosa. Nucleic Acid Ther. 2012;22(5):323–334.
- Ghosal A, Vitali A, Stach JEM, Nielsen PE. Role of SbmA in the uptake of peptide nucleic acid (PNA)-peptide conjugates in E. coli. ACS Chem Biol. 2013;8(2):360–367.
- Goltermann L, Yavari N, Zhang M, Ghosal A, Nielsen PE. PNA length restriction of antibacterial activity of peptide-PNA conjugates in Escherichia coli through effects of the inner membrane. Front Microbiol. 2019;10(MAY):1032.
- Yavari N, Goltermann L, Nielsen PE. Uptake, stability, and activity of antisense anti-acpP PNA-peptide conjugates in Escherichia coli and the role of SbmA. ACS Chem Biol. 2021;16(3):471–479.
- Corbalan N, Runti G, Adler C, Covaceuszach S, Ford RC, Lamba D, Beis K, Scocchi M, Vincent PA. Functional and structural study of the dimeric inner membrane protein SbmA. J Bacteriol. 2013;195(23):5352–5361.
- Goltermann L, Zhang M, Ebbensgaard AE, Fiodorovaite M, Yavari N, Løbner-Olesen A, Nielsen PE. Effects of LPS composition in Escherichia coli on antibacterial activity and bacterial uptake of antisense peptide-PNA conjugates. Front Microbiol. 2022;13:877377.
- Montagner G, Bezzerri V, Cabrini G, Fabbri E, Borgatti M, Lampronti I, Finotti A, Nielsen PE, Gambari R. An antisense peptide nucleic acid against Pseudomonas aeruginosa inhibiting bacterial-induced inflammatory responses in the cystic fibrosis IB3-1 cellular model system. Int J Biol Macromol. 2017;99:492–498.
- Hansen AM, Bonke G, Larsen CJ, Yavari N, Nielsen PE, Franzyk H. Antibacterial peptide nucleic acid-antimicrobial peptide (PNA-AMP) conjugates: antisense targeting of fatty acid biosynthesis. Bioconjug Chem. 2016;27(4):863–867.
- Hansen AM, Bonke G, Hogendorf WFJ, Björkling F, Nielsen J, Kongstad KT, Zabicka D, Tomczak M, Urbas M, Nielsen PE, et al. Microwave-assisted solid-phase synthesis of antisense acpP peptide nucleic acid-peptide conjugates active against colistin- and tigecycline-resistant E. coli and K. pneumoniae. Eur J Med Chem. 2019;168:134–145.
- Iubatti M, Gabas IM, Cavaco LM, Mood EH, Lim E, Bonanno F, Yavari N, Brolin C, Nielsen PE. Antisense peptide nucleic acid-diaminobutanoic acid dendron conjugates with SbmA-independent antimicrobial activity against gram-negative bacteria. ACS Infect Dis. 2022;8(5):1098–1106.
- da Silva KE, Ribeiro SM, Rossato L, Dos Santos CP, Preza SE, Cardoso MH, Franco OL, Migliolo L, Simionatto S. Antisense peptide nucleic acid inhibits the growth of KPC-producing Klebsiella pneumoniae strain. Res Microbiol. 2021;172(4–5):103837–5.
- Nejad AJ, Shahrokhi N, Nielsen PE. Targeting of the essential acpP, ftsZ, and rne genes in carbapenem-resistant Acinetobacter baumannii by antisense PNA precision antibacterials. Biomedicines. 2021;9(4):429.
- Otsuka T, Brauer AL, Kirkham C, Sully EK, Pettigrew MM, Kong Y, Geller BL, Murphy TF. Antimicrobial activity of antisense peptide-peptide nucleic acid conjugates against non-typeable Haemophilus influenzae in planktonic and biofilm forms. J Antimicrob Chemother. 2017;72(1):137–144.
- Sugimoto S, Maeda H, Kitamatsu M, Nishikawa I, Shida M. Selective growth inhibition of Porphyromonas gingivalis and Aggregatibacter actinomycetemcomitans by antisense peptide nucleic acids. Mol Cell Probes. 2019;43:45–49.
- Popella L, Jung J, Popova K, Urica-Mitic S, Barquist L, Vogel J. Global RNA profiles show target selectivity and physiological effects of peptide-delivered antisense antibiotics. Nucleic Acids Res. 2021;49(8):4705–4724.
- Ghosh S, Saini S, Saraogi I. Peptide nucleic acid mediated inhibition of the bacterial signal recognition particle. Chem Commun. 2018;54(59):8257–8260.
- Równicki M, Pieńko T, Czarnecki J, Kolanowska M, Bartosik D, Trylska J. Artificial activation of Escherichia coli mazEF and hipBA toxin–antitoxin systems by antisense peptide nucleic acids as an antibacterial strategy. Front Microbiol. 2018;9(NOV):2870.
- Wang X, Wang Y, Ling Z, Zhang C, Fu M, Wang Y, Wang S, Zhang S, Shen Z. Peptide nucleic acid restores colistin susceptibility through modulation of MCR-1 expression in Escherichia coli. J Antimicrob Chemother. 2020;75(8):2059–2065.
- Campion C, Charbon G, Thomsen TT, Nielsen PE, Løbner-Olesen A. Antisense inhibition of the Escherichia coli NrdAB aerobic ribonucleotide reductase is bactericidal due to induction of DNA strand breaks. J Antimicrob Chemother. 2021;76(11):2802–2814.
- Barkowsky G, Lemster A-L, Pappesch R, Jacob A, Krüger S, Schröder A, Kreikemeyer B, Patenge N. Influence of different cell-penetrating peptides on the antimicrobial efficiency of PNAs in Streptococcus pyogenes. Mol Ther Nucleic Acids. 2019;18:444–454.
- Narenji H, Teymournejad O, Rezaee MA, Taghizadeh S, Mehramuz B, Aghazadeh M, Asgharzadeh M, Madhi M, Gholizadeh P, Ganbarov K, et al. Antisense peptide nucleic acids againstftsZ andefaA genes inhibit growth and biofilm formation of Enterococcus faecalis. Microb Pathog. 2020; 139:103907.
- Równicki M, Dąbrowska Z, Wojciechowska M, Wierzba AJ, Maximova K, Gryko D, Trylska J. Inhibition of Escherichia coli growth by vitamin B12-peptide nucleic acid conjugates. ACS Omega. 2019;4(1):819–824.
- Castillo JI, Równicki M, Wojciechowska M, Trylska J. Antimicrobial synergy between mRNA targeted peptide nucleic acid and antibiotics in E. coli. Bioorg Med Chem Lett. 2018;28(18):3094–3098.
- Martínez-Guitián M, Vázquez-Ucha JC, Álvarez-Fraga L, Conde-Pérez K, Bou G, Poza M, Beceiro A. Antisense inhibition of lpxB gene expression in Acinetobacter baumannii by peptide-PNA conjugates and synergy with colistin. J Antimicrob Chemother. 2020;75(1):51–59.
- Yamamoto K, Yamamoto N, Ayukawa S, Yasutake Y, Ishiya K, Nakashima N. Scaffold size-dependent effect on the enhanced uptake of antibiotics and other compounds by Escherichia coli and Pseudomonas aeruginosa. Sci Rep. 2022;12(1):1–12.
- Vaara M, Siikanen O, Apajalahti J, Fox J, Frimodt-Møller N, He H, Poudyal A, Li J, Nation RL, Vaara T. A novel polymyxin derivative that lacks the fatty acid tail and carries only three positive charges has strong synergism with agents excluded by the intact outer membrane. Antimicrob Agents Chemother. 2010;54(8):3341–3346.
- González-García I, Mangas-Sanjuán V, Merino-Sanjuán M, Bermejo M. In vitro-in vivo correlations: general concepts, methodologies and regulatory applications. Drug Dev Ind Pharm. 2015;41(12):1935–1947.
- Knight A. Systematic reviews of animal experiments demonstrate poor contributions toward human healthcare. Rev Recent Clin Trials. 2008;3(2):89–96.
- Rai J, Kaushik K. Reduction of animal sacrifice in biomedical science & research through alternative design of animal experiments. Saudi Pharm J. 2018;26(6):896–902.
- Garattini S, Grignaschi G. Animal testing is still the best way to find new treatments for patients. Eur J Intern Med. 2017;39:32–35.
- Freires IA, Sardi J. d C, de Castro RD, Rosalen PL. Alternative animal and non-animal models for drug discovery and development: bonus or burden? Pharm Res. 2017;34(4):681–686.
- Jaroch K, Jaroch A, Bojko B. Cell cultures in drug discovery and development: the need of reliable in vitro-in vivo extrapolation for pharmacodynamics and pharmacokinetics assessment. J Pharm Biomed Anal. 2018;147:297–312.
- Middleton AM, Reynolds J, Cable S, Baltazar MT, Li H, Bevan S, Carmichael PL, Dent MP, Hatherell S, Houghton J, et al. Are non-animal systemic safety assessments protective? A Toolbox and workflow. Toxicol Sci. 2022;189(1):124–147.
- Hernández Yero LY, Pinos-Rodríguez D, Gibert JM. I. Animals devoid of pulmonary system as infection models in the study of lung bacterial pathogens. Front Microbiol. 2015;6(FEB):38.
- Lebeaux D, Chauhan A, Rendueles O, Beloin C. From in vitro to in vivo models of bacterial biofilm-related infections. Pathogens. 2013;2(2):288–356.
- Watkins-Chow DE, Pavan WJ. Genomic copy number and expression variation within the C57BL/6J inbred mouse strain. Genome Res. 2008;18(1):60–66.
- Mizgerd JP, Skerrett SJ. Animal models of human pneumonia. Am J Physiol Lung Cell Mol Physiol. 2008;294(3):L387–L398.
- Irvin CG, Bates JHT. Measuring the lung function in the mouse: the challenge of size. Respir Res. 2003;4(1):1–9.
- Bal HS, Ghoshal NG. Morphology of the terminal bronchiolar region of common laboratory mammals. Lab Anim. 1988;22(1):76–82.
- Tyler WS. Comparative subgross anatomy of lungs. Pleuras, interlobular septa, and distal airways. Am Rev Respir Dis. 1983;128(2 Pt 2):S32–S36.
- Jeffery PK. Morphologic features of airway surface epithelial cells and glands. Am Rev Respir Dis. 1983;128(2 Pt 2):S14–S20.
- Pack RJ, Al-Ugaily LH, Morris G. The cells of the tracheobronchial epithelium of the mouse: a quantitative light and electron microscope study. J Anat. 1981;132(Pt 1):71.
- Plopper CG. Comparative morphologic features of bronchiolar epithelial cells. The Clara cell. Am Rev Respir Dis. 1983;128(2 Pt 2):S37–S41.
- Rehli M. Of mice and men: species variations of toll-like receptor expression. Trends Immunol. 2002;23(8):375–378.
- Schneemann M, Schoeden G. Macrophage biology and immunology: man is not a mouse. J Leukoc Biol. 2007;81(3):579–579.
- Moser C, Hougen HP, Song Z, Rygaard J, Kharazmi A, Høiby N. Early immune response in susceptible and resistant mice strains with chronic Pseudomonas aeruginosa lung infection determines the type of T-helper cell response. APMIS. 1999;107(12):1093–1100.
- Moser C, Johansen HK, Song Z, Hougen HP, Rygaard J, Høiby N. Chronic Pseudomonas aeruginosa lung infection is more severe in Th2 responding BALB/c mice compared to Th1 responding. C3H/HeN mice. APMIS. 1997;105(11):838–842.
- Starke JR, Edwards MS, Langston C, Baker CJ. A mouse model of chronic pulmonary infection with Pseudomonas aeruginosa and Pseudomonas cepacia. Pediatr Res. 1987;22(6):698–702.
- Bragonzi A. Murine models of acute and chronic lung infection with cystic fibrosis pathogens. Int J Med Microbiol. 2010;300(8):584–593.
- Baldan R, Cigana C, Testa F, Bianconi I, De Simone M, Pellin D, Di Serio C, Bragonzi A, Cirillo DM. Adaptation of Pseudomonas aeruginosa in cystic fibrosis airways influences virulence of Staphylococcus aureus in vitro and murine models of co-infection. PLOS One. 2014;9(3):e89614.
- Bragonzi A, Farulla I, Paroni M, Twomey KB, Pirone L, Lorè NI, Bianconi I, Dalmastri C, Ryan RP, Bevivino A. Modelling co-infection of the cystic fibrosis lung by Pseudomonas aeruginosa and Burkholderia cenocepacia reveals influences on biofilm formation and host response. PLOS One. 2012;7(12):e52330.
- Hoffmann N, Rasmussen TB, Jensen PØ, Stub C, Hentzer M, Molin S, Ciofu O, Givskov M, Johansen HK, Høiby N. Novel mouse model of chronic Pseudomonas aeruginosa lung infection mimicking cystic fibrosis. Infect Immun. 2005;73(4):2504–2514.
- Moser C, Kjaergaard S, Pressler T, Kharazmi A, Koch C, Høiby N. The immune response to chronic Pseudomonas aeruginosa lung infection in cystic fibrosis patients is predominantly of the Th2 type. APMIS. 2000;108(5):329–335.
- Moser C, Van Gennip M, Bjarnsholt T, Jensen PØ, Lee B, Hougen HP, Calum H, Ciofu O, Givskov M, Molin S, et al. Novel experimental Pseudomonas aeruginosa lung infection model mimicking long-term host-pathogen interactions in cystic fibrosis. APMIS. 2009;117(2):95–107.
- Aoki N, Tateda K, Kikuchi Y, Kimura S, Miyazaki C, Ishii Y, Tanabe Y, Gejyo F, Yamaguchi K. Efficacy of colistin combination therapy in a mouse model of pneumonia caused by multidrug-resistant Pseudomonas aeruginosa. J Antimicrob Chemother. 2009;63(3):534–542.
- Jacqueline C, Roquilly A, Desessard C, Boutoille D, Broquet A, Le Mabecque V, Amador G, Potel G, Caillon J, Asehnoune K. Efficacy of ceftolozane in a murine model of Pseudomonas aeruginosa acute pneumonia: in vivo antimicrobial activity and impact on host inflammatory response. J Antimicrob Chemother. 2013;68(1):177–183.
- Lorenz A, Pawar V, Häussler S, Weiss S. Insights into host-pathogen interactions from state-of-the-art animal models of respiratory Pseudomonas aeruginosa infections. FEBS Lett. 2016;590(21):3941–3959.
- Louie A, Fregeau C, Liu W, Kulawy R, Drusano GL. Pharmacodynamics of levofloxacin in a murine pneumonia model of Pseudomonas aeruginosa infection: determination of epithelial lining fluid targets. Antimicrob Agents Chemother. 2009;53(8):3325–3330.
- Maciá MD, Borrell N, Segura M, Gómez C, Pérez JL, Oliver A. Efficacy and potential for resistance selection of antipseudomonal treatments in a mouse model of lung infection by hypermutable Pseudomonas aeruginosa. Antimicrob Agents Chemother. 2006;50(3):975–983.
- Sabet M, Miller CE, Nolan TG, Senekeo-Effenberger K, Dudley MN, Griffith DC. of aerosol MP-376, a levofloxacin inhalation solution, in models of mouse lung infection due to Pseudomonas aeruginosa. Antimicrob Agents Chemother. 2009;53(9):3923–3928.
- van Gennip M, Moser C, Christensen LD. Augmented effect of early antibiotic treatment in mice with experimental lung infections due to sequentially adapted mucoid strains of Pseudomonas aeruginosa. J Antimicrob Chemother. 2009;64(6):1241–1250.
- Cash HA, Woods DE, McCullough B, Johanson WG, Bass JA. A rat model of chronic respiratory infection with Pseudomonas aeruginosa. Am Rev Respir Dis. 1979;119(3):453–459.
- Stenvang Pedersen S, Shand GH, Langvad Hansen B, Norgaard Hansen G. Induction of experimental chronic Pseudomonas aeruginosa lung infection with P. aeruginosa entrapped in alginate microspheres. APMIS. 1990;98(1-6):203–211.
- ECDC. Infographic healthcare-associated infections – a threat to patient safety in Europe. European Center for Disease Prevention and control [cited 2022 Jun 22]. Available from: https://www.ecdc.europa.eu/en/publications-data/infographic-healthcare-associated-infections-threat-patient-safety-europe.
- Cassini A, Plachouras D, Eckmanns T, Abu Sin M, Blank H-P, Ducomble T, Haller S, Harder T, Klingeberg A, Sixtensson M, et al. Burden of six healthcare-associated infections on european population health: Estimating incidence-based disability-adjusted life years through a population prevalence-based modelling study. PLOS Med. 2016;13(10):e1002150.
- Limper AH. Overview of pneumonia. In: Goldman L, Schafer AI, editors. Goldman’s Cecil medicine: twenty fourth edition. Vol. 1. Philadelphia: Saunders Elsevier; 2012. p. 587–596.
- Torres A, Niederman MS, Chastre J, Ewig S, Fernandez-Vandellos P, Hanberger H, Kollef M, Li Bassi G, Luna CM, Martin-Loeches I, et al. International ERS/ESICM/ESCMID/ALAT guidelines for the management of hospital-acquired pneumonia and ventilator-associated pneumonia: Guidelines for the management of hospital-acquired pneumonia (HAP)/ventilator-associated pneumonia (VAP) of the. Eur Respir J. 2017;50(3):1700582.
- Papazian L, Klompas M, Luyt CE. Ventilator-associated pneumonia in adults: a narrative review. Intensive Care Med. 2020;46(5):888–906.
- Güner CK, Kutlutürkan S. Role of head-of-bed elevation in preventing ventilator-associated pneumonia bed elevation and pneumonia. Nurs Crit Care. 2022;27(5):635–645.
- Pericolini E, Colombari B, Ferretti G, Iseppi R, Ardizzoni A, Girardis M, Sala A, Peppoloni S, Blasi E. Real-time monitoring of Pseudomonas aeruginosa biofilm formation on endotracheal tubes in vitro. BMC Microbiol. 2018;18(1):1–10.
- Koulenti D, Tsigou E, Rello J. Nosocomial pneumonia in 27 ICUs in Europe: perspectives from the EU-VAP/CAP study. Eur J Clin Microbiol Infect Dis. 2017;36(11):1999–2006.
- Melsen WG, Rovers MM, Groenwold RHH, Bergmans DCJJ, Camus C, Bauer TT, Hanisch EW, Klarin B, Koeman M, Krueger WA, et al. Attributable mortality of ventilator-associated pneumonia: a meta-analysis of individual patient data from randomised prevention studies. Lancet Infect Dis. 2013;13(8):665–671.
- Mathew E, Domínguez-Robles J, Larrañeta E, Lamprou DA. Fused deposition modelling as a potential tool for antimicrobial dialysis catheters manufacturing: new trends vs. conventional approaches. Coatings. 2019;9(8):515.
- Bielen K, Jongers B, Malhotra-Kumar S, Jorens PG, Goossens H, Kumar-Singh S. Animal models of hospital-acquired pneumonia: current practices and future perspectives. Ann Transl Med. 2017;5(6):132.
- Clarke LL, Grubb BR, Gabriel SE, Smithies O, Koller BH, Boucher RC. Defective epithelial chloride transport in a gene-targeted mouse model of cystic fibrosis. Science. 1992;257(5073):1125–1128.
- Hoffmann N, Lee B, Hentzer M, Rasmussen TB, Song Z, Johansen HK, Givskov M, Høiby N. Azithromycin blocks quorum sensing and alginate polymer formation and increases the sensitivity to serum and stationary-growth-phase killing of Pseudomonas aeruginosa and attenuates chronic P. aeruginosa lung infection in Cftr(-/-) mice. Antimicrob Agents Chemother. 2007;51(10):3677–3687.
- Bassi GL, Senussi T, Xiol EA. Prevention of ventilator-associated pneumonia. Curr Opin Infect Dis. 2017;30(2):214–220.
- Yanagihara K, Tomono K, Sawai T, Hirakata Y, Kadota J, Koga H, Tashiro T, Kohno S. Effect of clarithromycin on lymphocytes in chronic respiratory Pseudomonas aeruginosa infection. Am J Respir Crit Care Med. 1997;155(1):337–342.
- Kaku N, Morinaga Y, Takeda K, Kosai K, Uno N, Hasegawa H, Miyazaki T, Izumikawa K, Mukae H, Yanagihara K. Efficacy and pharmacokinetics of ME1100, a novel optimized formulation of arbekacin for inhalation, compared with amikacin in a murine model of ventilator-associated pneumonia caused by Pseudomonas aeruginosa. J Antimicrob Chemother. 2017;72(4):1123–1128.
- Kaneko Y, Yanagihara K, Kuroki M, Ohi H, Kakeya H, Miyazaki Y, Higashiyama Y, Hirakata Y, Tomono K, Kadota JI, et al. Effects of parenterally administered ciprofloxacin in a murine model of pulmonary Pseudomonas aeruginosa infection mimicking ventilator-associated pneumonia. Chemotherapy. 2001;47(6):421–429.
- Yamada K, Yamamoto Y, Yanagihara K, Araki N, Harada Y, Morinaga Y, Izumikawa K, Kakeya H, Hasegawa H, Kohno S, et al. In vivo efficacy and pharmacokinetics of biapenem in a murine model of ventilator-associated pneumonia with Pseudomonas aeruginosa. J Infect Chemother. 2012;18(4):472–478.
- Burleson GR, Burleson FG. Testing human biologicals in animal host resistance models. J Immunotoxicol. 2008;5(1):23–31.
- Zecconi A, Scali F. Staphylococcus aureus virulence factors in evasion from innate immune defenses in human and animal diseases. Immunol Lett. 2013;150(1–2):12–22.
- Borsa ND, Pasquale M, Restrepo MI. Animal models of Pneumococcal pneumonia. IJMS. 2019;20(17):4220.
- Berra L, De Marchi L, Yu ZX, Laquerriere P, Baccarelli A, Kolobow T. Endotracheal tubes coated with antiseptics decrease bacterial colonization of the ventilator circuits, lungs, and endotracheal tube. Anesthesiology. 2004;100(6):1446–1456.
- Berra L, Curto F, Li Bassi G, Laquerriere P, Baccarelli A, Kolobow T. Antibacterial-coated tracheal tubes cleaned with the Mucus Shaver : a novel method to retain long-term bactericidal activity of coated tracheal tubes. Intensive Care Med. 2006;32(6):888–893.
- Fernández-Barat L, Li Bassi G, Ferrer M, Bosch A, Calvo M, Vila J, Gabarrús A, Martínez-Olondris P, Rigol M, Esperatti M, et al. Direct analysis of bacterial viability in endotracheal tube biofilm from a pig model of methicillin-resistant Staphylococcus aureus pneumonia following antimicrobial therapy. FEMS Immunol Med Microbiol. 2012;65(2):309–317.
- Olson ME, Harmon BG, Kollef MH. Silver-coated endotracheal tubes associated with reduced bacterial burden in the lungs of mechanically ventilated dogs. Chest. 2002;121(3):863–870.
- Jean SS, Chang YC, Lin WC, Lee W, Sen Hsueh PR, Hsu CW. Epidemiology, treatment, and prevention of nosocomial bacterial pneumonia. JCM. 2020;9(1):275.
- Koenig SM, Truwit JD. Ventilator-associated pneumonia: diagnosis, treatment, and prevention. Clin Microbiol Rev. 2006;19(4):637–657.
- Dutta S, Sengupta P. Men and mice: relating their ages. Life Sci. 2016;152:244–248.
- Facchini M, Fino Riva DI, Bragonzi C. A. Long term chronic Pseudomonas aeruginosa airway infection in mice. J Vis Exp. 2014;(85):e51019.
- Zhao M, Lepak AJ, Andes DR. Animal models in the pharmacokinetic/pharmacodynamic evaluation of antimicrobial agents. Bioorg Med Chem. 2016;24(24):6390–6400.
- Christophersen LJ, Trøstrup H, Malling Damlund DS, Bjarnsholt T, Thomsen K, Jensen PØ, Hougen HP, Høiby N, Moser C. Bead-size directed distribution of Pseudomonas aeruginosa results in distinct inflammatory response in a mouse model of chronic lung infection. Clin Exp Immunol. 2012;170(2):222–230.
- Kukavica-Ibrulj I, Levesque RC. Animal models of chronic lung infection with Pseudomonas aeruginosa: useful tools for cystic fibrosis studies. Lab Anim. 2008;42(4):389–412.
- Schwab U, Abdullah LH, Perlmutt OS, Albert D, Davis CW, Arnold RR, Yankaskas JR, Gilligan P, Neubauer H, Randell SH, et al. Localization of Burkholderia cepacia complex bacteria in cystic fibrosis lungs and interactions with Pseudomonas aeruginosa in hypoxic mucus. Infect Immun. 2014;82(11):4729–4745.
- Fux CA, Costerton JW, Stewart PS, Stoodley P. Survival strategies of infectious biofilms. Trends Microbiol. 2005;13(1):34–40.
- Waters CM, Bassler BL. Quorum sensing: cell-to-cell communication in bacteria. Annu Rev Cell Dev Biol. 2005;21:319–346.
- Brackman G, Coenye T. Quorum sensing inhibitors as anti-biofilm agents. Curr Pharm Des. 2015;21(1):5–11.
- Qvortrup K, Hultqvist LD, Nilsson M. Small molecule anti-biofilm agents developed on the basis of mechanistic understanding of biofilm formation. Front Chem. 2019;7:742.
- Parsek MR, Val DL, Hanzelka BL, Cronan JE, Greenberg EP. Acyl homoserine-lactone quorum-sensing signal generation. Proc Natl Acad Sci USA. 1999;96(8):4360–4365.
- Hanzelka BL, Greenberg EP. Quorum sensing in Vibrio fischeri: evidence that S-adenosylmethionine is the amino acid substrate for autoinducer synthesis. J Bacteriol. 1996;178(17):5291–5294.
- Favre-Bonté S, Köhler T, Van Delden C. Biofilm formation by Pseudomonas aeruginosa: role of the C4-HSL cell-to-cell signal and inhibition by azithromycin. J Antimicrob Chemother. 2003;52(4):598–604.
- Uroz S, Dessaux Y, Oger P. Quorum sensing and quorum quenching: the Yin and Yang of bacterial communication. Chembiochem. 2009;10(2):205–216.
- Zhang R-g, Pappas KM, Brace JL, Miller PC, Oulmassov T, Molyneaux JM, Anderson JC, Bashkin JK, Winans SC, Joachimiak A. Structure of a bacterial quorum-sensing transcription factor complexed with pheromone and DNA. Nature. 2002;417(6892):971–974.
- Christiaen SEA, Brackman G, Nelis HJ, Coenye T. Isolation and identification of quorum quenching bacteria from environmental samples. J Microbiol Methods. 2011;87(2):213–219.
- Chen F, Gao Y, Chen X, Yu Z, Li X. Quorum quenching enzymes and their application in degrading signal molecules to block quorum sensing-dependent infection. Int J Mol Sci. 2013;14(9):17477–17500.
- Brackman G, Risseeuw M, Celen S, Cos P, Maes L, Nelis HJ, Van Calenbergh S, Coenye T. Synthesis and evaluation of the quorum sensing inhibitory effect of substituted triazolyldihydrofuranones. Bioorg Med Chem. 2012;20(15):4737–4743.
- Morohoshi T, Shiono T, Takidouchi K, Kato M, Kato N, Kato J, Ikeda T. Inhibition of quorum sensing in Serratia marcescens AS-1 by synthetic analogs of N-acylhomoserine lactone. Appl Environ Microbiol. 2007;73(20):6339–6344.
- Ishida T, Ikeda T, Takiguchi N, Kuroda A, Ohtake H, Kato J. Inhibition of quorum sensing in Pseudomonas aeruginosa by N-acyl cyclopentylamides. Appl Environ Microbiol. 2007;73(10):3183–3188.
- Girennavar B, Cepeda ML, Soni KA, Vikram A, Jesudhasan P, Jayaprakasha GK, Pillai SD, Patil BS. Grapefruit juice and its furocoumarins inhibits autoinducer signaling and biofilm formation in bacteria. Int J Food Microbiol. 2008;125(2):204–208.
- Rasmussen TB, Bjarnsholt T, Skindersoe ME, Hentzer M, Kristoffersen P, Köte M, Nielsen J, Eberl L, Givskov M. Screening for quorum-sensing inhibitors (QSI) by use of a novel genetic system, the QSI selector. J Bacteriol. 2005;187(5):1799–1814.
- Bjarnsholt T, Jensen PØ, Rasmussen TB, Christophersen L, Calum H, Hentzer M, Hougen H-P, Rygaard J, Moser C, Eberl L, et al. Garlic blocks quorum sensing and promotes rapid clearing of pulmonary Pseudomonas aeruginosa infections. Microbiology. 2005;151(Pt 12):3873–3880.
- Jakobsen TH, van Gennip M, Phipps RK, Shanmugham MS, Christensen LD, Alhede M, Skindersoe ME, Rasmussen TB, Friedrich K, Uthe F, et al. Ajoene, a sulfur-rich molecule from garlic, inhibits genes controlled by quorum sensing. Antimicrob Agents Chemother. 2012;56(5):2314–2325.
- Barzegari A, Kheyrolahzadeh K, Hosseiniyan Khatibi SM, Sharifi S, Memar MY, Zununi Vahed S. The battle of probiotics and their derivatives against biofilms. Infect Drug Resist. 2020;13:659–672.
- Gomaa EZ. Antimicrobial and anti-adhesive properties of biosurfactant produced by lactobacilli isolates, biofilm formation and aggregation ability. J Gen Appl Microbiol. 2013;59(6):425–436.
- Tan Y, Leonhard M, Moser D, Schneider-Stickler B. Inhibition activity of Lactobacilli supernatant against fungal-bacterial multispecies biofilms on silicone. Microb Pathog. 2017;113:197–201.
- Sharma V, Harjai K, Shukla G. Effect of bacteriocin and exopolysaccharides isolated from probiotic on P. aeruginosa PAO1 biofilm. Folia Microbiol (Praha)). 2018;63(2):181–190.
- Merghni A, Dallel I, Noumi E, Kadmi Y, Hentati H, Tobji S, Ben Amor A, Mastouri M. Antioxidant and antiproliferative potential of biosurfactants isolated from Lactobacillus casei and their anti-biofilm effect in oral Staphylococcus aureus strains. Microb Pathog. 2017;104:84–89.
- Kim Y, Oh S, Kim SH. Released exopolysaccharide (r-EPS) produced from probiotic bacteria reduce biofilm formation of enterohemorrhagic Escherichia coli O157:H7. Biochem Biophys Res Commun. 2009;379(2):324–329.
- Pan D, Mei X. Antioxidant activity of an exopolysaccharide purified from Lactococcus lactis subsp. lactis 12. Carbohydr Polym. 2010;80(3):908–914.
- Wasfi R, Abd El-Rahman OA, Zafer MM, Ashour HM. Probiotic Lactobacillus sp. inhibit growth, biofilm formation and gene expression of caries‐inducing Streptococcus mutans. J Cell Mol Med. 2018;22(3):1972–1983.
- Kiymaci ME, Altanlar N, Gumustas M, Ozkan SA, Akin A. Quorum sensing signals and related virulence inhibition of Pseudomonas aeruginosa by a potential probiotic strain’s organic acid. Microb Pathog. 2018;121:190–197.
- Fong FLY, Shah NP, Kirjavainen P, El-Nezami H. Mechanism of action of probiotic bacteria on intestinal and systemic immunities and antigen-presenting cells. Int Rev Immunol. 2016;35(3):179–188.
- Maccelli A, Carradori S, Puca V, Sisto F, Lanuti P, Crestoni ME, Lasalvia A, Muraro R, Bysell H, Di Sotto A, et al. Correlation between the antimicrobial activity and metabolic profiles of cell free supernatants and membrane vesicles produced by Lactobacillus reuteri DSM 17938. Microorganisms. 2020;8(11):1653–22.
- Rigauts C, Aizawa J, Taylor SL, Rogers GB, Govaerts M, Cos P, Ostyn L, Sims S, Vandeplassche E, Sze M, et al. Rothia mucilaginosa is an anti-inflammatory bacterium in the respiratory tract of patients with chronic lung disease. Eur Respir J. 2022;59(5):2101293.
- European Centre for Disease Prevention and Control (ECDC). Summary of the latest data on antibiotic resistance in the European Union. Euro-CDC; 2012, p. 1–4. [cited 2022 Jun 27]. Available from: https://www.ecdc.europa.eu/en/publications-data/summary-latest-data-antibiotic-resistance-european-union.
- Marchianò V, Matos M, Serrano-Pertierra E, Gutiérrez G, Blanco-López MC. Vesicles as antibiotic carrier: state of art. Int J Pharm. 2020;585:119478.
- European Commission. EU action on antimicrobial resistance | Public Health [cited 2022 Jun 22]. https://ec.europa.eu/health/antimicrobial-resistance/eu-action-on-antimicrobial-resistance_en.
- Vader P, Mol EA, Pasterkamp G, Schiffelers RM. Extracellular vesicles for drug delivery. Adv Drug Deliv Rev. 2016;106(Pt A):148–156.
- Jan T, Iqbal J, Ismail M, Badshah N, Mansoor Q, Arshad A, Ahkam QM. Synthesis, physical properties and antibacterial activity of metal oxides nanostructures. Mater Sci Semicond Process. 2014;21:154–160.
- Vega-Jiménez AL, Vázquez-Olmos AR, Acosta-Gío E, Álvarez-Pérez MA. In vitro antimicrobial activity evaluation of metal oxide nanoparticles. In: Koh KS, Wong VL, editors. Nanoemulsions – properties, fabrications and applications. London: IntechOpen; 2019. p. 1–18.
- Ghafelehbashi R, Akbarzadeh I, Tavakkoli Yaraki M, Lajevardi A, Fatemizadeh M, Heidarpoor Saremi L. Preparation, physicochemical properties, in vitro evaluation and release behavior of cephalexin-loaded niosomes. Int J Pharm. 2019;569:118580.
- Ranghar S, Sirohi P, Verma P, Agarwal V. Nanoparticle-based drug delivery systems: promising approaches against infections. Braz Arch Biol Technol. 2013;57(2):209–222.
- Mitchell MJ, Billingsley MM, Haley RM, Wechsler ME, Peppas NA, Langer R. Engineering precision nanoparticles for drug delivery. Nat Rev Drug Discov. 2021;20(2):101–124.
- Yetisgin AA, Cetinel S, Zuvin M, Kosar A, Kutlu O. Therapeutic nanoparticles and their targeted delivery applications. Molecules. 2020;25(9):2193.
- Eleraky NE, Allam A, Hassan SB, Omar MM. Nanomedicine fight against antibacterial resistance: an overview of the recent pharmaceutical innovations. Pharmaceutics. 2020;12(2):142.
- Miller KP, Wang L, Benicewicz BC, Decho AW. Inorganic nanoparticles engineered to attack bacteria. Chem Soc Rev. 2015;44(21):7787–7807.
- Lah NAC, Samykano M, Trigueros S. Nanoscale metal particles as nanocarriers in targeted drug delivery system. J Nanomedicine Res. 2016;4(2):2–7.
- McNamara K, Tofail SAM. Nanoparticles in biomedical applications. Adv Phys X. 2017;2(1):54–88.
- Długosz O, Szostak K, Staroń A, Pulit-Prociak J, Banach M. Methods for reducing the toxicity of metal and metal oxide NPs as biomedicine. Materials. 2020;13(2):279.
- Beyth N, Houri-Haddad Y, Domb A, Khan W, Hazan R. Alternative antimicrobial approach: nano-antimicrobial materials. Evidence-Based Complement Altern Med. 2015;2015:1–16.
- Aderibigbe BA. Metal-based nanoparticles for the treatment of infectious diseases. Molecules. 2017;22(8):1370.
- Zhang X-F, Liu Z-G, Shen W, Gurunathan S. Silver nanoparticles: Synthesis, characterization, properties, applications, and therapeutic approaches. IJMS. 2016;17(9):1534.
- Rai M, Yadav A, Gade A. Silver nanoparticles as a new generation of antimicrobials. Biotechnol Adv. 2009;27(1):76–83.
- Kim JS, Kuk E, Yu KN, Kim J-H, Park SJ, Lee HJ, Kim SH, Park YK, Park YH, Hwang C-Y, et al. Antimicrobial effects of silver nanoparticles. Nanomedicine. 2007;3(1):95–101.
- Avalos A, Haza AI, Mateo D, Morales P. Interactions of manufactured silver nanoparticles of different sizes with normal human dermal fibroblasts. Int Wound J. 2016;13(1):101–109.
- Aditya NP, Vathsala PG, Vieira V, Murthy RSR, Souto EB. Advances in nanomedicines for malaria treatment. Adv Colloid Interface Sci. 2013;201–202:1–17.
- Poulose S, Panda T, Nair PP, Théodore T. Biosynthesis of silver nanoparticles. J Nanosci Nanotechnol. 2014;14(2):2038–2049.
- Panacek A, Kvítek L, Prucek R, Kolar M, Vecerova R, Pizúrova N, Sharma VK, Nevecna T, Zboril R. Silver colloid nanoparticles: Synthesis, characterization, and their antibacterial activity. J Phys Chem B. 2006;110(33):16248–16253.
- De Simone S, Gallo AL, Paladini F, Sannino A, Pollini M. Development of silver nano-coatings on silk sutures as a novel approach against surgical infections. J Mater Sci Mater Med. 2014;25(9):2205–2214.
- Leid JG, Ditto AJ, Knapp A, Shah PN, Wright BD, Blust R, Christensen L, Clemons CB, Wilber JP, Young GW, et al. In vitro antimicrobial studies of silver carbene complexes: activity of free and nanoparticle carbene formulations against clinical isolates of pathogenic bacteria. J Antimicrob Chemother. 2012;67(1):138–148.
- Chernousova S, Epple M. Silver as antibacterial agent: Ion, nanoparticle, and metal. Angew Chem Int Ed Engl. 2013;52(6):1636–1653.
- Panáček A, Kolář M, Večeřová R, Prucek R, Soukupová J, Kryštof V, Hamal P, Zbořil R, Kvítek L. Antifungal activity of silver nanoparticles against Candida spp. Biomaterials. 2009;30(31):6333–6340.
- Silver S. Bacterial silver resistance: Molecular biology and uses and misuses of silver compounds. FEMS Microbiol Rev. 2003;27(2-3):341–353.
- Ug A, Ceylan O. Occurrence of resistance to antibiotics, metals, and plasmids in clinical strains of Staphylococcus spp. Arch Med Res. 2003;34(2):130–136.
- Tolaymat TM, El Badawy AM, Genaidy A, Scheckel KG, Luxton TP, Suidan M. An evidence-based environmental perspective of manufactured silver nanoparticle in syntheses and applications: a systematic review and critical appraisal of peer-reviewed scientific papers. Sci Total Environ. 2010;408(5):999–1006.
- Bartłomiejczyk T, Lankoff A, Kruszewski M, Szumiel I. Silver nanoparticles – allies or adversaries? Ann Agric Environ Med. 2013;20(1):48–54.
- Majdalawieh A, Kanan MC, El-Kadri O, Kanan SM. Recent advances in gold and silver nanoparticles: synthesis and applications. J Nanosci Nanotechnol. 2014;14(7):4757–4780.
- Din FU, Aman W, Ullah I, Qureshi OS, Mustapha O, Shafique S, Zeb A. Effective use of nanocarriers as drug delivery systems for the treatment of selected tumors. Int J Nanomedicine. 2017;12:7291–7309.
- Khurana C, Vala AK, Andhariya N, Pandey OP, Chudasama B. Antibacterial activities of silver nanoparticles and antibiotic-adsorbed silver nanoparticles against biorecycling microbes. Environ Sci Process Impacts. 2014;16(9):2191–2198.
- Shahverdi AR, Fakhimi A, Shahverdi HR, Minaian S. Synthesis and effect of silver nanoparticles on the antibacterial activity of different antibiotics against Staphylococcus aureus and Escherichia coli. Nanomed Nanotechnol Biol Med. 2007;3(2):168–171.
- Kubo A-L, Capjak I, Vrček IV, Bondarenko OM, Kurvet I, Vija H, Ivask A, Kasemets K, Kahru A. Antimicrobial potency of differently coated 10 and 50 nm silver nanoparticles against clinically relevant bacteria Escherichia coli and Staphylococcus aureus. Colloids Surf B Biointerfaces. 2018;170:401–410.
- Grande R, Sisto F, Puca V, Carradori S, Ronci M, Aceto A, Muraro R, Mincione G, Scotti L. Antimicrobial and antibiofilm activities of new synthesized silver ultra-nanoclusters (SUNCs) against Helicobacter pylori. Front Microbiol. 2020;11:1705.
- Dakal TC, Kumar A, Majumdar RS, Yadav V. Mechanistic basis of antimicrobial actions of silver nanoparticles. Front Microbiol. 2016;7(NOV):1831.
- Sánchez-López E, Gomes D, Esteruelas G, Bonilla L, Lopez-Machado AL, Galindo R, Cano A, Espina M, Ettcheto M, Camins A, et al. Metal-based nanoparticles as antimicrobial agents: an overview. Nanomaterials. 2020;10(2):292.
- Ciriminna R, Albo Y, Pagliaro M. New antivirals and antibacterials based on silver nanoparticles. ChemMedChem. 2020;15(17):1619–1623.
- Puca V, Traini T, Guarnieri S, Carradori S, Sisto F, Macchione N, Muraro R, Mincione G, Grande R. The antibiofilm effect of a medical device containing TiAb on microorganisms associated with surgical site infection. Molecules. 2019;24(12):2280.
- Gerber A, Bundschuh M, Klingelhofer D, Groneberg DA. Gold nanoparticles: recent aspects for human toxicology. J Occup Med Toxicol. 2013;8(1):32–36.
- Her S, Jaffray DA, Allen C. Gold nanoparticles for applications in cancer radiotherapy: mechanisms and recent advancements. Adv Drug Deliv Rev. 2017;109:84–101.
- Shamaila S, Zafar N, Riaz S, Sharif R, Nazir J, Naseem S. Gold nanoparticles: An efficient antimicrobial agent against enteric bacterial human pathogen. Nanomaterials. 2016;6(4):71.
- MubarakAli D, Thajuddin N, Jeganathan K, Gunasekaran M. Plant extract mediated synthesis of silver and gold nanoparticles and its antibacterial activity against clinically isolated pathogens. Colloids Surf B Biointerfaces. 2011;85(2):360–365.
- Ayaz Ahmed KB, Subramanian S, Sivasubramanian A, Veerappan G, Veerappan A. Preparation of gold nanoparticles using Salicornia brachiata plant extract and evaluation of catalytic and antibacterial activity. Spectrochim Acta A Mol Biomol Spectrosc. 2014;130:54–58.
- Yougbare S, Chang T-K, Tan S-H, Kuo J-C, Hsu P-H, Su C-Y, Kuo T-R. Antimicrobial gold nanoclusters: recent developments and future perspectives. IJMS. 2019;20(12):2924.
- Abbaszadegan A, Ghahramani Y, Gholami A, Hemmateenejad B, Dorostkar S, Nabavizadeh M, Sharghi H. The effect of charge at the surface of silver nanoparticles on antimicrobial activity against gram-positive and gram-negative bacteria: A preliminary study. J Nanomater. 2015;2015:1–8.
- Losasso C, Belluco S, Cibin V, Zavagnin P, Mičetić I, Gallocchio F, Zanella M, Bregoli L, Biancotto G, Ricci A. Antibacterial activity of silver nanoparticles: sensitivity of different Salmonella serovars. Front Microbiol. 2014;5(MAY):227.
- Zia R, Riaz M, Farooq N, Qamar A, Anjum S. Antibacterial activity of Ag and Cu nanoparticles synthesized by chemical reduction method: a comparative analysis. Mater Res Express. 2018;5(7):075012.
- Wang L, Hu C, Shao L. The antimicrobial activity of nanoparticles: present situation and prospects for the future. Int J Nanomedicine. 2017;12:1227–1249.
- Rajendran K, Anwar A, Khan NA, Siddiqui R. Brain-eating amoebae: Silver nanoparticle conjugation enhanced efficacy of anti-amoebic drugs against Naegleria fowleri. ACS Chem Neurosci. 2017;8(12):2626–2630.
- Faedmaleki F, Shirazi FH, Salarian AA, Ashtiani HA, Rastegar H. Toxicity effect of silver nanoparticles on mice liver primary cell culture and HepG2 cell line. Iran J Pharm Res. 2014;13(1):235–242.
- Sruthi S, Ashtami J, Mohanan PV. Biomedical application and hidden toxicity of zinc oxide nanoparticles. Mater Today Chem. 2018;10:175–186.
- Zhang Y, Chen W, Wang S, Liu Y, Pope C. Phototoxicity of zinc oxide nanoparticle conjugates in human ovarian cancer NIH: OVCAR-3 cells. j iomed Nanotechnol. 2008;4(4):432–438.
- Allahverdiyev AM, Abamor ES, Bagirova M, Rafailovich M. Antimicrobial effects of TiO2 and Ag2O nanoparticles against drug-resistant bacteria and leishmania parasites. Future Microbiol. 2011;6(8):933–940.
- Wei C, Lin WY, Zainal Z, Williams NE, Zhu K, Kruzic AP, Smith RL, Rajeshwar K. Bactericidal activity of TiO2 photocatalyst in aqueous media: toward a solar-assisted water disinfection system. Environ Sci Technol. 1994;28(5):934–938.
- Allahverdiyev AM, Abamor ES, Bagirova M, Baydar SY, Ates SC, Kaya F, Kaya C, Rafailovich M. Investigation of antileishmanial activities of Tio2@Ag nanoparticles on biological properties of L. tropica and L. infantum parasites, in vitro. Exp Parasitol. 2013;135(1):55–63.
- Sudakar C, Dixit A, Regmi R, Naik R, Lawes G, Naik VM, Vaishnava PP, Toti U, Panyam J. Fe3O4 incorporated AOT-alginate nanoparticles for drug delivery. IEEE Trans Magn. 2008;44(11):2800–2803.
- Jarosz M, Kapusta-Kołodziej J, Pawlik A, Syrek K, Sulka GD. Drug delivery systems based on titania nanostructures. In: Andronescu E, Grumezescu AM, editors. Nanostructures for drug delivery. Amsterdam: Elsevier; 2017. p. 299–326.
- Zhang H, Wang C, Chen B, Wang X. Daunorubicin-TiO2 nanocomposites as a “smart” pH-responsive drug delivery system. Int J Nanomedicine. 2012;7:235–242.
- Gomathi Devi L, Nagaraj B. Disinfection of Escherichia Coli gram negative bacteria using surface modified TiO2: optimization of Ag metallization and depiction of charge transfer mechanism. Photochem Photobiol. 2014;90(5):1089–1098.
- Ungureanu C, Popescu S, Purcel G, Tofan V, Popescu M, Sălăgeanu A, Pîrvu C. Improved antibacterial behavior of titanium surface with torularhodin-polypyrrole film. Mater Sci Eng C Mater Biol Appl. 2014;42:726–733.
- Petronella F, Truppi A, Striccoli M, Curri ML, Comparelli R. Photocatalytic application of Ag/TiO2 hybrid nanoparticles. In: Mohapatra S, Nguyen TA, Nguyen-Tri P, editors. Noble metal-metal oxide hybrid nanoparticles: fundamentals and applications. Sawston: Woodhead Publishing; 2018. p. 373–394.
- Mohammad MR, Ahmed DS, Mohammed MKA. Synthesis of Ag-doped TiO2 nanoparticles coated with carbon nanotubes by the sol–gel method and their antibacterial activities. J Sol-Gel Sci Technol. 2019;90(3):498–509.
- Harandi FN, Khorasani AC, Shojaosadati SA, Hashemi-Najafabadi S. Surface modification of electrospun wound dressing material by Fe2O3 nanoparticles incorporating Lactobacillus strains for enhanced antimicrobial and antibiofilm activity. Surf. Interfaces. 2022; 28:101592.
- Sihem L, Hanine D, Faiza B. Antibacterial activity of α-Fe2O3 and α-Fe2O3@Ag nanoparticles prepared by Urtica leaf extract. Nanotechnol Russia. 2020;15(2):198–203.
- Li D, Shen M, Xia J, Shi X. Recent developments of cancer nanomedicines based on ultrasmall iron oxide nanoparticles and nanoclusters. Nanomedicine. 2021;16(8):609–612.
- Anghel AG, Grumezescu AM, Chirea M, Grumezescu V, Socol G, Iordache F, Oprea AE, Anghel I, Holban AM. MAPLE fabricated Fe3O4@Cinnamomum verum antimicrobial surfaces for improved gastrostomy tubes. Molecules. 2014;19(7):8981–8994.
- Alavijeh MS, Bani MS, Rad I, Hatamie S, Zomorod MS, Haghpanahi M. Antibacterial properties of ferrimagnetic and superparamagnetic nanoparticles: a comparative study. J Mech Sci Technol. 2021;35(2):815–821.
- Taylor EN, Webster TJ. The use of superparamagnetic nanoparticles for prosthetic biofilm prevention. Int J Nanomedicine. 2009;4:145–152.
- Taylor EN, Kummer KM, Durmus NG, Leuba K, Tarquinio KM, Webster TJ. Superparamagnetic iron oxide nanoparticles (SPION) for the treatment of antibiotic-resistant biofilms. Small. 2012;8(19):3016–3027.
- Armijo LM, Wawrzyniec SJ, Kopciuch M, Brandt YI, Rivera AC, Withers NJ, Cook NC, Huber DL, Monson TC, Smyth HDC, et al. Antibacterial activity of iron oxide, iron nitride, and tobramycin conjugated nanoparticles against Pseudomonas aeruginosa biofilms. J Nanobiotechnol. 2020;18(1):1–27.
- Subbiahdoss G, Sharifi S, Grijpma DW, Laurent S, van der Mei HC, Mahmoudi M, Busscher HJ. Magnetic targeting of surface-modified superparamagnetic iron oxide nanoparticles yields antibacterial efficacy against biofilms of gentamicin-resistant Staphylococci. Acta Biomater. 2012;8(6):2047–2055.
- Mahmoudi M, Serpooshan V. Silver-coated engineered magnetic nanoparticles are promising for the success in the fight against antibacterial resistance threat. ACS Nano. 2012;6(3):2656–2664.
- Niemirowicz-Laskowska K, Głuszek K, Piktel E, Pajuste K, Durnaś B, Król G, Wilczewska AZ, Janmey PA, Plotniece A, Bucki R. Bactericidal and immunomodulatory properties of magnetic nanoparticles functionalized by 1,4-dihydropyridines. Int J Nanomedicine. 2018;13:3411–3424.
- Gudkov SV, Burmistrov DE, Serov DA, Rebezov MB, Semenova AA, Lisitsyn AB. Do iron oxide nanoparticles have significant antibacterial properties? Antibiotics. 2021;10(7):884.
- Innocenzi P, Stagi L. Carbon-based antiviral nanomaterials: graphene, C-dots, and fullerenes. A perspective. Chem Sci. 2020;11(26):6606–6622.
- Dizaj SM, Mennati A, Jafari S, Khezri K, Adibkia K. Antimicrobial activity of carbon-based nanoparticles. Adv Pharm Bull. 2015;5(1):19–23.
- Smith SC, Rodrigues DF. Carbon-based nanomaterials for removal of chemical and biological contaminants from water: a review of mechanisms and applications. Carbon N Y. 2015;91:122–143.
- Abo-Neima SE, Motaweh HA, Elsehly EM. Antimicrobial activity of functionalised carbon nanotubes against pathogenic microorganisms. IET Nanobiotechnol. 2020;14(6):457–464.
- Ghirardello M, Ramos-Soriano J, Galan MC. Carbon dots as an emergent class of antimicrobial agents. Nanomaterials. 2021;11(8):1877.
- Davoodi E, Zhianmanesh M, Montazerian H, Milani AS, Hoorfar M. Nano-porous anodic alumina: fundamentals and applications in tissue engineering. J Mater Sci Mater Med. 2020;31(7):60.
- Seaberg J, Montazerian H, Hossen MN, Bhattacharya R, Khademhosseini A, Mukherjee P. Hybrid nanosystems for biomedical applications. ACS Nano. 2021;15(2):2099–2142.
- Chen F, Hableel G, Zhao ER, Jokerst JV. Multifunctional nanomedicine with silica: Role of silica in nanoparticles for theranostic, imaging, and drug monitoring. J Colloid Interface Sci. 2018;521:261–279.
- Bernardos A, Piacenza E, Sancenón F, Hamidi M, Maleki A, Turner RJ, Martínez‐Máñez R. Mesoporous silica-based materials with bactericidal properties. Small. 2019;15(24):1900669.
- Colilla M, Vallet-Regí M. Targeted stimuli-responsive mesoporous silica nanoparticles for bacterial infection treatment. IJMS. 2020;21(22):8605–8632.
- Castillo RR, Vallet-Regí M. Recent advances toward the use of mesoporous silica nanoparticles for the treatment of bacterial infections. Int J Nanomedicine. 2021;16:4409–4430.
- Smirnov NA, Kudryashov SI, Nastulyavichus AA, Rudenko AA, Saraeva IN, Tolordava ER, Gonchukov SA, Romanova YM, Ionin AA, Zayarny DA. Antibacterial properties of silicon nanoparticles. Laser Phys Lett. 2018;15(10):105602.
- Selvarajan V, Obuobi S, Ee PLR. Silica nanoparticles—a versatile tool for the treatment of bacterial infections. Front Chem. 2020;8:602.
- Valizadeh A, Mikaeili H, Samiei M, Farkhani SM, Zarghami N, Kouhi M, Akbarzadeh A, Davaran S. Quantum dots: synthesis, bioapplications, and toxicity. Nanoscale Res Lett. 2012;7(1):480–414.
- Owusu EGA, Macrobert AJ, Naasani I, Parkin IP, Allan E, Yaghini E. Photoactivable polymers embedded with cadmium-free quantum dots and crystal violet: efficient bactericidal activity against clinical strains of antibiotic-resistant bacteria. ACS Appl Mater Interfaces. 2019;11(13):12367–12378.
- Rajendiran K, Zhao Z, Pei DS, Fu A. Antimicrobial activity and mechanism of functionalized quantum dots. Polymers. 2019;11(10):1670.
- Dizaj SM, Lotfipour F, Barzegar-Jalali M, Zarrintan MH, Adibkia K. Antimicrobial activity of the metals and metal oxide nanoparticles. Mater Sci Eng C Mater Biol Appl. 2014;44:278–284.
- Levy M, Bertram JR, Eller KA, Chatterjee A, Nagpal P. Near-infrared-light-triggered antimicrobial indium phosphide quantum dots. Angew Chem Int Ed Engl. 2019;58(33):11414–11418.
- McCollum CR, Levy M, Bertram JR, Nagpal P, Chatterjee A. Photoexcited quantum dots as efficacious and nontoxic antibiotics in an animal model. ACS Biomater Sci Eng. 2021;7(5):1863–1875.
- Martin-Serrano Á, Gómez R, Ortega P, Mata FJDL. Nanosystems as vehicles for the delivery of antimicrobial peptides (AMPs). Pharmaceutics. 2019;11(9):448.
- Ferreira M, Ogren M, Dias JNR, Silva M, Gil S, Tavares L, Aires-da-Silva F, Gaspar MM, Aguiar SI. Liposomes as antibiotic delivery systems: a promising nanotechnological strategy against antimicrobial resistance. Molecules. 2021;26(7):2047.
- Deshmukh RR, Gawale V, Kailas Bhagwat M, Ahire A, Diliprao Derle N. A review on: liposomes. World J Pharm Pharm Sci. 2016;5(3):506–517.
- Priyanka R, Bhattacharyya S. A review on promising antibiotic therapy by novel delivery systems. Asian J Pharm Clin Res. 2018;11(5):18–24.
- Sharma A, Kumar Arya D, Dua M, Chhatwal GS, Johri AK. Nano-technology for targeted drug delivery to combat antibiotic resistance. Expert Opin Drug Deliv. 2012;9(11):1325–1332.
- Lian T, Ho RJY. Trends and developments in liposome drug delivery systems. J Pharm Sci. 2001;90(6):667–680.
- Rai A, Comune M, Ferreira L. Nanoparticle-based drug delivery systems: Promising approaches against bacterial infections. In: Ahmad I, Ahmad S, Rumbaugh K, editors. Antibacterial drug discovery to combat MDR. Singapore: Springer; 2019. p. 605–633.
- Fang CL, Aljuffali IA, Li YC, Fang JY. Delivery and targeting of nanoparticles into hair follicles. Ther Deliv. 2014;5(9):991–1006.
- Yeh YC, Huang TH, Yang SC, Chen CC, Fang JY. Nano-based drug delivery or targeting to eradicate bacteria for infection mitigation: a review of recent advances. Front Chem. 2020;8:286.
- Karami N, Moghimipour E, Salimi A. Liposomes as a novel drug delivery system: fundamental and pharmaceutical application. Asian J Pharm. 2018;12(1):S31–S41.
- Mamizuka EM, Carmona-Ribeiro AM. Cationic liposomes as antimicrobial agents. In: Mendez-Vilas A, editors. Communicating current research and educational topics and trends in applied microbiology. Badajoz: Formatex; 2007. p. 636–647.
- Skwarczynski M, Bashiri S, Yuan Y, Ziora ZM, Nabil O, Masuda K, Khongkow M, Rimsueb N, Cabral H, Ruktanonchai U, et al. Antimicrobial activity enhancers: towards smart delivery of antimicrobial agents. Antibiotics. 2022; 11(3):412.
- Kang SN, Hong SS, Kim SY, Oh H, Lee MK, Lim SJ. Enhancement of liposomal stability and cellular drug uptake by incorporating tributyrin into celecoxib-loaded liposomes. Asian J Pharm Sci. 2013;8(2):138–148.
- Gonzalez Gomez A, Xu C, Hosseinidoust Z. Preserving the efficacy of glycopeptide antibiotics during nanoencapsulation in liposomes. ACS Infect Dis. 2019;5(10):1794–1801.
- Yang Z, Liu J, Gao J, Chen S, Huang G. Chitosan coated vancomycin hydrochloride liposomes: characterizations and evaluation. Int J Pharm. 2015;495(1):508–515.
- Gonzalez Gomez A, Hosseinidoust Z. Liposomes for antibiotic encapsulation and delivery. ACS Infect Dis. 2020;6(5):896–908.
- Moorcroft SCT, Jayne DG, Evans SD, Ong ZY. Stimuli-responsive release of antimicrobials using hybrid inorganic nanoparticle-associated drug-delivery systems. Macromol Biosci. 2018;18(12):1800207.
- Devnarain N, Osman N, Fasiku VO, et al. Intrinsic stimuli-responsive nanocarriers for smart drug delivery of antibacterial agents—an in-depth review of the last two decades. Wiley Interdiscip Rev Nanomedicine Nanobiotechnology. 2021;13(1):e1664.
- Zhang W, Hu E, Wang Y, Miao S, Liu Y, Hu Y, Liu J, Xu B, Chen D, Shen Y. Emerging antibacterial strategies with application of targeting drug delivery system and combined treatment. Int J Nanomedicine. 2021;16:6141–6156.
- Wang DY, van der Mei HC, Ren Y, Busscher HJ, Shi L. Lipid-based antimicrobial delivery-systems for the treatment of bacterial infections. Front Chem. 2020;7:872.
- Cheung Lam AH, Sandoval N, Wadhwa R, Gilkes J, Do TQ, Ernst W, Chiang S-M, Kosina S, Howard Xu H, Fujii G, et al. Assessment of free fatty acids and cholesteryl esters delivered in liposomes as novel class of antibiotic. BMC Res Notes. 2016;9(1):1–11.
- Rucins M, Dimitrijevs P, Pajuste K, Petrichenko O, Jackevica L, Gulbe A, Kibilda S, Smits K, Plotniece M, Tirzite D, et al. Contribution of molecular structure to self-assembling and biological properties of bifunctional lipid-like 4-(N-alkylpyridinium)-1,4-dihydropyridines. Pharmaceutics. 2019;11(3):115.
- Richards S-J, Keenan T, Vendeville J-B, Wheatley DE, Chidwick H, Budhadev D, Council CE, Webster CS, Ledru H, Baker AN, et al. Introducing affinity and selectivity into galectin-targeting nanoparticles with fluorinated glycan ligands. Chem Sci. 2020;12(3):905–910.
- Liu D, Chen L, Jiang S, Zhu S, Qian Y, Wang F, Li R, Xu Q. Formulation and characterization of hydrophilic drug diclofenac sodium-loaded solid lipid nanoparticles based on phospholipid complexes technology. J Liposome Res. 2014;24(1):17–26.
- Kheradmandnia S, Vasheghani-Farahani E, Nosrati M, Atyabi F. Preparation and characterization of ketoprofen-loaded solid lipid nanoparticles made from beeswax and carnauba wax. Nanomedicine. 2010;6(6):753–759.
- Yadav N, Khatak SS, Sara UV. Solid lipid nanoparticles – a review. Int J Appl Pharm. 2013;5(2):8–18.
- Kalhapure RS, Mocktar C, Sikwal DR, Sonawane SJ, Kathiravan MK, Skelton A, Govender T. Ion pairing with linoleic acid simultaneously enhances encapsulation efficiency and antibacterial activity of vancomycin in solid lipid nanoparticles. Colloids Surf B Biointerfaces. 2014;117:303–311.
- Álvarez-Paino M, Muñoz-Bonilla A, Fernández-García M. Antimicrobial polymers in the nano-world. Nanomaterials. 2017;7(2):48.
- Xiong MH, Bao Y, Yang XZ, Zhu YH, Wang J. Delivery of antibiotics with polymeric particles. Adv Drug Deliv Rev. 2014;78:63–76.
- Imbuluzqueta E, Lemaire S, Gamazo C, Elizondo E, Ventosa N, Veciana J, Van Bambeke F, Blanco-Prieto MJ. Cellular pharmacokinetics and intracellular activity against Listeria monocytogenes and Staphylococcus aureus of chemically modified and nanoencapsulated gentamicin. J Antimicrob Chemother. 2012;67(9):2158–2164.
- Karthikeyan R, Koushik OS, Kumar PV. Dendrimeric architecture for effective antimicrobial therapy. In: Sharma AK, Keservani RK, editors. Dendrimers for drug delivery. Palm Bay, FL, USA: Apple Academic Press Inc.; 2019. p. 375–405.
- Authimoolam SP, Dziubla TD. Biopolymeric mucin and synthetic polymer analogs: their structure, function and role in biomedical applications. Polymers. 2016;8(3):71.
- Chis AA, Dobrea C, Morgovan C, Arseniu AM, Rus LL, Butuca A, Juncan AM, Totan M, Vonica-Tincu AL, Cormos G, et al. Applications and limitations of dendrimers in biomedicine. Molecules. 2020;25(17):3982.
- Bhadra D, Yadav AK, Bhadra S, Jain NK. Glycodendrimeric nanoparticulate carriers of primaquine phosphate for liver targeting. Int J Pharm. 2005;295(1–2):221–233.
- Scorciapino MA, Serra I, Manzo G, Rinaldi AC. Antimicrobial dendrimeric peptides: structure, activity and new therapeutic applications. IJMS. 2017;18(3):542.
- Selin M, Nummelin S, Deleu J, Ropponen J, Viitala T, Lahtinen M, Koivisto J, Hirvonen J, Peltonen L, Kostiainen MA, et al. High-generation amphiphilic janus-dendrimers as stabilizing agents for drug suspensions. Biomacromolecules. 2018;19(10):3983–3993.
- Falanga A, Del Genio V, Kaufman EA, Zannella C, Franci G, Weck M, Galdiero S. Engineering of janus-like dendrimers with peptides derived from glycoproteins of Herpes simplex virus type 1: toward a versatile and novel antiviral platform.IJMS. 2021;22(12):6488.
- Rabea EI, Badawy MET, Stevens CV, Smagghe G, Steurbaut W. Chitosan as antimicrobial agent: applications and mode of action. Biomacromolecules. 2003;4(6):1457–1465.
- Machul A, Mikołajczyk D, Regiel-Futyra A, Heczko PB, Strus M, Arruebo M, Stochel G, Kyzioł A. Study on inhibitory activity of chitosan-based materials against biofilm producing Pseudomonas aeruginosa strains. J Biomater Appl. 2015;30(3):269–278.
- Han C, Romero N, Fischer S, Dookran J, Berger A, Doiron AL. Recent developments in the use of nanoparticles for treatment of biofilms. Nanotechnol Rev. 2017;6(5):383–404.
- Li J, Zhuang S. Antibacterial activity of chitosan and its derivatives and their interaction mechanism with bacteria: current state and perspectives. Eur Polym J. 2020;138:109984.
- Enríquez de Salamanca A, Diebold Y, Calonge M, García-Vazquez C, Callejo S, Vila A, Alonso MJ. Chitosan nanoparticles as a potential drug delivery system for the ocular surface: toxicity, uptake mechanism and in vivo tolerance. Invest Ophthalmol Vis Sci. 2006;47(4):1416–1425.
- Hassani Najafabadi A, Abdouss M, Faghihi S. Synthesis and evaluation of PEG-O-chitosan nanoparticles for delivery of poor water soluble drugs: ibuprofen. Mater Sci Eng C Mater Biol Appl. 2014;41:91–99.
- Tan Y, Ma S, Liu C, Yu W, Han F. Enhancing the stability and antibiofilm activity of DspB by immobilization on carboxymethyl chitosan nanoparticles. Microbiol Res. 2015;178:35–41.
- Jana S, Kumar Sen K, Gandhi A. Alginate based nanocarriers for drug delivery applications. Curr Pharm Des. 2016;22(22):3399–3410.
- Hariyadi DM, Islam N. Current status of alginate in drug delivery. Adv Pharmacol Pharm Sci. 2020;2020(2):8886095–8886222.
- Aldawsari MF, Ahmed MM, Fatima F, Anwer MK, Katakam P, Khan A. Development and characterization of calcium-alginate beads of apigenin: in vitro antitumor, antibacterial, and antioxidant activities. Mar Drugs. 2021;19(8):467.
- Dinarvand R, Sepehri N, Manoochehri S, Rouhani H, Atyabi F. Polylactide-co-glycolide nanoparticles for controlled delivery of anticancer agents. Int J Nanomedicine. 2011;6:877–895.
- Hiraki J. Basic and applied studies on ε-polylysine. J Antibact Antifung Agents. 1995;23:349–354.
- Beyth N, Yudovin-Farber I, Perez-Davidi M, Domb AJ, Weiss EI. Polyethyleneimine nanoparticles incorporated into resin composite cause cell death and trigger biofilm stress in vivo. Proc Natl Acad Sci USA. 2010;107(51):22038–22043.
- Taheri S, Baier G, Majewski P, Barton M, Förch R, Landfester K, Vasilev K. Synthesis and surface immobilization of antibacterial hybrid silver-poly(l-lactide) nanoparticles. Nanotechnology. 2014;25(30):305102.
- Kho K, Cheow WS, Lie RH, Hadinoto K. Aqueous re-dispersibility of spray-dried antibiotic-loaded polycaprolactone nanoparticle aggregates for inhaled anti-biofilm therapy. Powder Technol. 2010;203(3):432–439.
- Ghosh S, Acharyya M, Mandal SM. Novolac-based polymer-silver nanoparticles hybrid: Synthesis, characterization and antibacterial evaluation. CAPS. 2019;3(1):75–82.
- Jiang L, Lee HW, Loo SCJ. Therapeutic lipid-coated hybrid nanoparticles against bacterial infections. RSC Adv. 2020;10(14):8497–8517.
- Yang S, Han X, Yang Y, Qiao H, Yu Z, Liu Y, Wang J, Tang T. Bacteria-targeting nanoparticles with microenvironment-responsive antibiotic release to eliminate intracellular Staphylococcus aureus and associated infection. ACS Appl Mater Interfaces. 2018;10(17):14299–14311.
- Dai X, Guo Q, Zhao Y, Zhang P, Zhang T, Zhang X, Li C. Functional silver nanoparticle as a benign antimicrobial agent that eradicates antibiotic-resistant bacteria and promotes wound healing. ACS Appl Mater Interfaces. 2016;8(39):25798–25807.
- Baek J-S, Tan CH, Ng NKJ, Yeo YP, Rice SA, Loo SCJ. A programmable lipid-polymer hybrid nanoparticle system for localized, sustained antibiotic delivery to Gram-positive and Gram-negative bacterial biofilms. Nanoscale Horiz. 2018;3(3):305–311.
- Castro NR, Pinto CSC, Santos EP, Mansur CRE. Hybrid vesicular nanosystems based on lipids and polymers applied in therapy, theranostics, and cosmetics. Crit Rev Ther Drug Carrier Syst. 2020;37(3):271–303.