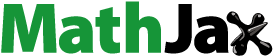
Abstract
Carbonic anhydrases (CAs) are widespread metalloenzymes which catalyse the reversible hydration of carbon dioxide (CO2) to bicarbonate (HCO3−) and a proton, relevant in many physiological processes. In the last few years, the involvement of CA activation in different metabolic pathways in the human brain addressed the research to the discovery of novel CA activators. Here, a new series of isoxazoline-based amino alcohols as CA activators was investigated. The synthesis and the CA activating effects towards four human CA isoforms expressed in the human brain, that are hCAs I, II, IV and VII, were reported. The best results were obtained for the (methyl)-isoxazoline-amino alcohols 3 and 5 with KA values in the submicromolar range (0.52–0.86 µM) towards hCA VII, and a good selectivity over hCA I. Being hCA VII involved in brain function and metabolism, the newly identified CA activators might be promising hit compounds with potential therapeutic applications in ageing, epilepsy or neurodegeneration.
Introduction
Carbonic anhydrases (CAs) are a superfamily of ubiquitous metalloenzymes responsible for the catalysis of the reversible hydration of carbon dioxide (CO2) to bicarbonate (HCO3−) by proton transfer mechanismCitation1. This simple reaction is essential to different biological processes, and it is represented by EquationEquation (1)(1)
(1) :
(1)
(1)
Although this reaction may also occur spontaneously, at physiological pH values it is too slow to guarantee metabolic needs and maintain homeostasis. In that context, CA catalysis becomes relevant in covering all the physiological processes speeding up the equilibrium of CO2/HCO3− interconversion.
Generally, CAs are phylogenetically classified into different subgroups: α-, β-, γ-, δ-, ζ-, η-, θ, and ιCitation2. Human CAs belong to the α-class, and are represented by 15 isoforms of which 12 are catalytically activeCitation3. The catalytic core is maintained in all the α-isoforms and it is constituted by a Zn2+ ion arranged in tetrahedral coordination with three His residues and a water molecule.
So far, CAs have been deeply explored as targets for the design of CA inhibitorsCitation4–6. In fact, CA inhibitors are in advanced pharmacological studies and some of them, in the last decade, reached clinical use for the treatment of different pathologies such as glaucomaCitation7, epilepsyCitation8 and obesityCitation9. Unfortunately, most of the CA inhibitors lack selectivity of action against the different CA isoformsCitation10 but also over other zinc metalloenzymes such as matrix metalloproteinases, due to the common structural requirement of a zinc-binding group in the inhibitor structureCitation11–14.
However, recently the less explored class of CA activators (CAAs) is gaining increased attention, principally due to the recent biological findings on CA activationCitation15. Some human isoform deficiencies are related to the development of specific pathological conditions such as osteopetrosis, hyperammonemia, hyperchlorhydrias and cystic fibrosisCitation16–20 Furthermore, the activation of CAs has been reported to increase memoryCitation21,Citation22 and promote bone mineralisationCitation23. Recent evidence focused on the involvement of CA activation in the treatment of neurological and neurodegenerative diseases, proposing an effective role of CA activation in the strengthening of synaptic efficacyCitation24.
In 1990, the general enzymatic mechanism of action of CAAs was reportedCitation25. The generation of a ternary enzyme-substrate-activator complex [EquationEquation (2)(2)
(2) ] is due to the non-competitive binding of the activator to the enzyme in the active site at the middle edge of CA, assisting the proton shuttlingCitation26.
(2)
(2)
The discovery of the activation mechanism turned on the interest in the activation target and many studies regarding CAAs have been carried outCitation27.
A CAA is usually a small molecule with the structural requirement of a basic moiety necessary to mimic the proton shuttling mechanism. Thus, the first molecules to be tested as CAAs have been amino acids and their respective amines, identifying histamine () as the prototypical CAACitation28. Therefore, at first, the CAA drug design was focused on histamine analogues, including histamine-inspired molecules, and His combined with its β-alanine dipeptide derivative carnosine ()Citation29. In the following years, with the purpose to explore the field of CAA Structure-Activity Relationships (SARs), different design approaches focused on amines and amino acid structures but were not strictly related to the parent compoundsCitation27. Among new scaffolds, β-arylchalcogeno amines containing sulfur, selenium and tellurium, structurally related to the psychoactive drug amphetamineCitation30, amino alcohol oxime ethersCitation31 and neuronal nitric oxide synthase (nNOS) inhibitor heterocycles conjugated with amino acids, represent the mainly investigated alternative to classic CAAsCitation32.
Of note, a large series of amino-alcohol derivatives, inspired by the lead CAA β-amino alcohol timolol, has been recently reported ()Citation31. Timolol is selective for hCA I and hCA II isoforms, binding the active site entrance through the formation of a ternary complex with enzyme and CO2Citation33. The new amino alcohol series of CAAs were obtained by ring opening of differently substituted epoxides with isopropylamine or tert-butylamine. All the amino alcohol derivatives showed good activation properties, reporting KA values spanning from a micromolar to a nanomolar range. In particular, compound 1 () turned out to be highly selective for hCA II and hCA VII, thus opening new perspectives in the use of amino alcohol scaffolds as potential CAAs.
Based on these promising results, we extended our SAR studies to an in-house library of isoxazoline amino alcohols, composed of diasteroisomeric isoxazolinyl-N-alkylethanolamines (2–23, ), some of them previously reported as antagonists of β-adrenergic receptorsCitation34–36. Taking into consideration that β-1 adrenergic receptor blockade was reported to have a positive effect on memory performanceCitation37, we here hypothesized that a dual activity of this class of compounds on CA activation and β-adrenergic receptor blockade could be beneficial for improving memory and cognitive functions. In this new series, an isoxazoline ring replaced the oxime moiety, as a conformationally restrained alternative structure, to explore the fitting inside the binding site, and gain new information about the SAR of amino alcohol CA activators. Similarly to the previously described amino alcohol series, these isoxazolines presented isopropyl or tert-butyl substituents in R1 ().
Table 1. Activation data of human CA isoforms I, II, IV and VII with isoxazoline amino alcohols 2–23 and histamine as reference CAA by a stopped-flow CO2 hydrase assayCitation38.
Here, we present our studies on amino alcohols as CAAs, describing the synthesis, NMR characterization and CA activating effects of this new series of isoxazoline-based amino alcohols towards four hCA isoforms mainly expressed in the human brain.
Materials and methods
Chemistry
1H and 13C NMR spectra were recorded on a Bruker Avance III HD 400 MHz spectrometer. Chemical shifts (δ) are reported in parts per million and coupling constants (J) are reported in hertz (Hz). 13C NMR spectra were fully decoupled. The following abbreviations were used to explain multiplicities: singlet (s), doublet (d), triplet (t), double doublet (dd), broad (br), and multiplet (m). Chromatographic separations were performed on silica gel columns by flash column chromatography (Kieselgel 40, 0.040 0.063 mm, Merck). Reactions were followed by thin-layer chromatography (TLC) on Merck aluminium silica gel (60 F254) sheets that were visualised under a UV lamp. Evaporation was performed in vacuo (rotating evaporator). Sodium sulphate was always used as the drying agent. Commercially available chemicals were purchased from Sigma-Aldrich.
Compounds prepared according to literature procedures
As mentioned in the introduction, compounds 2–5, 8–11, 12–15, and 22–23 34–36 () have been already reported as antagonists of β-adrenergic receptors. Here, we detail an updated characterisation by 1H NMR and 13C NMR spectra.
(syn)-2-(isopropylamino)-1-(3-methyl-4,5-dihydroisoxazol-5-yl)ethan-1-ol maleate (2): 1H NMR (400 MHz, DMSO-d6) δ: 6.01 (s, 2H); 4.40–4.34 (m, 1H); 3.66–3.63 (m, 1H); 3.00–2.89 (m, 3H); 2.75–2.73 (m, 1H); 1.91 (s, 3H); 1.19–1.18 (m, 6H).13C NMR (100 MHz, DMSO-d6) δ: 167.2; 155.5; 136.1; 80.6; 68.2; 49.4; 47.4; 19.9; 19.2; 12.6.
(anti)-2-(isopropylamino)-1-(3-methyl-4,5-dihydroisoxazol-5-yl)ethan-1-ol maleate (3): 1H NMR (400 MHz, DMSO-d6) δ: 8.45, 8.34 (2 × bs, 2H); 6.02 (s, 2H); 5.76–5.75 (d, J = 5.2 Hz, 1H); 4.45, 4.43 (dddd, J1 = 4.0 Hz, J2= 8.0 Hz, J3= 15.2 Hz, 1H); 3.78–3.77 (m, 1H); 3.02 (dd, J1 =7.2 Hz, J2 = 18 Hz, 1H); 2.89 (dd, J1= 7.6 Hz, J2= 16.8 Hz, 1H); 1.90 (s, 3H); 1.23 (m, 6H). 13C NMR (100 MHz, DMSO-d6) δ: 167.2; 155.6; 136.1; 80.3; 67.7; 49.8; 45.9; 18.8; 18.1; 12.6.
(syn)-2-(tert-butylamino)-1-(3-methyl-4,5-dihydroisoxazol-5-yl)ethan-1-ol maleate (4): 1H NMR (400 MHz, DMSO-d6) δ: 8.27 (bs, 2H); 6.01 (s, 2H); 5.85–5.84 (m, 1H); 4.43–4.37 (m, 1H); 3.69–3.67 (m, 1H); 3.06–2.97 (m, 3H); 2.75–2.71 (m, 1H); 1.92 (s, 3H); 1.28 (s, 9H). 13C NMR (100 MHz, DMSO-d6) δ: 167.1; 155.7; 136.1; 80.3; 67.7; 56.4; 43.7; 25.0; 12.6.
(anti)-2-(tert-butylamino)-1-(3-methyl-4,5-dihydroisoxazol-5-yl)ethan-1-ol maleate (5): 1H NMR (400 MHz, DMSO-d6) δ: 8.39, 8.30 (2 × bs, 2H); 6.01 (s, 2H); 5.73 (d, J = 5.2 Hz, 1H); 4.45 (dddd, J1 = 4.0 Hz, J2 = 8.0 Hz, J3=15.2 Hz, 1H); 3.75–3.73 (m, 1H); 3.87–2.83 (m, 4H); 1.91 (s, 3H); 1.28 (s, 9H). 13C NMR (100 MHz, DMSO-d6) δ: 167.1; 155.5; 136.1; 80.2; 68.1; 56.2; 43.3; 27.1; 25.1; 12.5.
(syn)-2-(isopropylamino)-1-(3-phenyl-4,5-dihydroisoxazol-5-yl)ethan-1-ol maleate (8): 1H NMR (400 MHz, DMSO-d6) δ: 8.34 (bs 2H); 7.69–7.66 (m, 2H); 7.49–7.47 (m, 3H); 6.01 (s, 2H); 5.98–5.97 (m, 1H); 4.68–4.62 (m, 1H); 3.86–3.83 (m, 1H); 3.49 (dd, J1= 10.6 Hz, J2= 17.2 Hz, 1H); 3.43 (dd, J1= 9.6 Hz, J2= 17.2 Hz, 1H); 3.11–3.09 (m, 1H); 2.91–2.86 (m, 1H); 1.24 (dd, J1= 3.6 Hz, J2= 6.4 Hz, 6H). 13C NMR (100 MHz, DMSO-d6) δ: 167.6; 157.1; 136.6; 130.7; 129.6; 129.4; 127.1; 82.1; 67.7; 50.4; 46.7; 36.2; 19.4; 18.6.
(anti)-2-(isopropylamino)-1-(3-phenyl-4,5-dihydroisoxazol-5-yl)ethan-1-ol oxalate (9): 1H NMR (400 MHz, DMSO-d6) δ: 7.68–7.64 (m, 2H); 7.48–7.42 (m, 3H); 4.98 (bs, 1H); 4.66 (dddd, J1= 4.4 Hz, J2= 8.8 Hz, J3 = 15.2 Hz, 1H); 3.57–3.53 (m, 1H); 3.30–3.23 (m, 1H); 2.76–2.69 (m, 1H); 2.65 (dd, J1=4.8 Hz, J2=12 Hz, 1H); 2.57 (dd, J1 = 8 Hz; J2= 11.6 Hz, 1H); 0.97 (dd, J1= 1.2 Hz, J2= 6 Hz, 6H). 13C NMR (100 MHz, DMSO-d6) δ: 156.4; 129.8; 129.6; 128.7; 126.4; 82.4; 70.9; 49.1; 48.2; 36.0; 22.8.
(syn)-2-(tert-butylamino)-1-(3-phenyl-4,5-dihydroisoxazol-5-yl)ethan-1-ol oxalate (10): 1H NMR (400 MHz, DMSO-d6) δ: 7.68–7.66 (m, 2H); 7.45–7.44 (m, 3H); 4.66 (td, J = 4.8 Hz, J = 9.2 Hz, 1H); 3.59–3.55 (m,1H); 3.35–3.33 (m, 2H); 2.58 (dd, J1=4.4 Hz, J2= 11.2 Hz, 1H); 2.52–2.47 (m, 1H); 1.29 (s, 9H). 13C NMR (100 MHz, DMSO-d6) δ: 156.3; 129.8; 129.7; 128.8; 126.4; 82.6; 70.7; 49.7; 45.1; 35.2; 28,8.
(anti)-2-(tert-butylamino)-1-(3-phenyl-4,5-dihydroisoxazol-5-yl)ethan-1-ol oxalate (11): 1H NMR (400 MHz, DMSO-d6) δ: 7.66–7.65 (m, 2H); 7.46-7.42 (m, 3H); 4.70 (dddd, J1= 4.4 Hz, J2= 8.8 Hz, J3= 12.8 Hz, 1H); 3.51–3.24 (m, 2H); 2.60–2.59 (m, 2H); 1.03 (s, 9H).13C NMR (100 MHz, DMSO-d6) δ: 156.8; 130.3; 130.1; 129.2; 126.9; 82.8; 72.0; 50.2; 45.0; 36.5; 29.2.
(syn)-1-(3-(2-chlorophenyl)-4,5-dihydroisoxazol-5-yl)-2-(isopropylamino)ethan-1-ol maleate (12): 1H NMR (400 MHz, DMSO-d6) δ: 8.38 (br s, 2H); 7.62–7.58 (m, 2H); 7.52–7.43 (m, 2H); 6.03–6.01 (m, 1H); 6.02 (s, 2H); 4.71–4.65 (m, 1H); 3.88–3.86 (m, 1H); 3.56 (dd, J1= 10.4 Hz, J2= 17.2 Hz, 1H); 3.51 (dd, J1= 7.6 Hz, J2= 17.6 Hz, 1H); 3.39–3.32 (m, 1H); 3.12–3.08 (dd J1= 2.4 Hz, J2= 10.4 Hz, 1H); 2.92 − 2.87 (m, 1H); 1.25–1.22 (dd, J1= 4 Hz, J2= 6.4 Hz, 6H). 13C NMR (100 MHz, DMSO-d6) δ: 167.2; 156.0; 136.1; 131.6; 131.4; 130.7; 130.6; 128.5; 127.5; 81.8; 67.1; 49.9; 46.2; 38.4; 18.8; 18.1.
(anti)-1-(3-(2-chlorophenyl)-4,5-dihydroisoxazol-5-yl)-2-(isopropylamino)ethan-1-ol maleate (13): 1H NMR (400 MHz, DMSO-d6) δ: 8.34 (br s, 2H); 7.62–7.57 (m, 2H); 7.51–7.42 (m, 2H); 6.02 (s, 2H); 5.92 (b s, 1H); 4.73 (dddd, J1= 4 Hz, J2= 7.6 Hz, J3= 11.2 Hz, 1H); 3.88–3.86 (m, 1H); 3.61 (dd, J1= 11.2 Hz, J2= 17.2 Hz, 1H); 3.38 (dd, J1= 11.2 Hz, J2= 17.2 Hz, 1H); 3.35-3.31 (m, 1H); 3.10 (dd, J1= 2.4 Hz, J2= 12.4 Hz, 1H); 2.98–2.95 (m, 1H); 1.25–1.22 (dd, J1= 4.4 Hz, J2= 6.4 Hz). 13C NMR (100 MHz, DMSO-d6) δ: 167.2; 155.9; 136.1; 131.6; 131.3; 130.7; 130.6; 128.5; 127.5; 81.7; 67.6; 49.8; 45.8; 38.5; 18.9; 18.1.
(syn)-2-(tert-butylamino)-1-(3-(2-chlorophenyl)-4,5-dihydroisoxazol-5-yl)ethanol maleate (14): 1H NMR (400 MHz, DMSO-d6) δ: 8.35 (bs, 2H); 7.63–7.58 (m, 2H); 7.53–7.43 (m, 2H); 6.02–6.01 (m, 1H); 6.02 (s, 2H); 4.73–4.67 (m, 1H); 3.87–3.85 (m, 1H); 3.55–3.52 (m, 2H); 3.09–3.07 (m, 1H); 2.85–2.82 (m, 1H); 1.48 (s, 9H). 13C NMR (100 MHz, DMSO-d6) δ: 167.2; 156.1; 136.2; 131.6; 131.4; 130.7; 130.6; 128.5; 127.6; 81.8; 67.4; 56.5; 43.5; 38.3; 25.1.
(anti)-2-(tert-butylamino)-1-(3-(2-chlorophenyl)-4,5-dihydroisoxazol-5-yl)ethanol maleate (15): 1H NMR (400 MHz, DMSO-d6) δ: 8.31 (bs, 2H); 7.63–7.57 (m, 2H); 7.52–7.43 (m, 2H); 6.01 (s, 2H); 5.89 (bs; 1H); 4.73–4.67 (m, 1H); 3.87–3.85 (m, 1H); 3.63 (dd, J1= 11.2 Hz, J2= 17.2 Hz, 1H); 3.42 (dd, J1= 7.6 Hz, J2= 17.2 Hz, 1H); 3.09–3.07 (m, 1H); 2.85–2.82 (m, 1H); 1.48 (s, 9H). 13C NMR (100 MHz, DMSO-d6) δ: 167.6; 156.3; 136.4; 132.0; 131.8; 131.2; 131.1; 129.0; 128.0; 82.1; 68.5; 56.9; 43.5; 39.0; 25.5.
(syn)-1-(3-(3-chlorophenyl)-4,5-dihydroisoxazol-5-yl)-2-(isopropylamino)ethan-1-ol maleate (22): 1H NMR (400 MHz, DMSO-d6) δ: 8.28 (bs, 2H); 7.69–7.65 (m, 2H); 7.57–7.49 (m, 2H); 6.02 (s, 2H); 6.02–6.01 (m, 1H); 4.72–4.66 (m, 1H); 3.87–3.84 (m, 1H); 3.52–3.25 (m, 2H); 3.11–3.08 (m, 1H); 2.90–2.84 (m, 1H); 1.24–1.22 (m, 6H). 13C NMR (100 MHz, DMSO-d6) δ: 167.7; 156.3; 136.6; 134.1; 131.7; 131.3; 130.5; 126.7; 125.7; 82.6; 67.7; 50.3; 46.6; 35.9; 19.4; 18.6.
(anti)-1-(3-(3-chlorophenyl)-4,5-dihydroisoxazol-5-yl)-2-(isopropylamino)ethan-1-ol maleate (23): 1H NMR (400 MHz, DMSO-d6) δ: 8.32 (bs, 2H); 7.68–7.64 (m, 2H); 7.56–7.49 (m, 2H); 6.01 (s, 2H); 5.86 (bs, 1H); 4.76-4.68 (m, 1H); 3.86–3.84 (m,1H); 3.57–3.48 (m, 1H); 3.45–3.43 (m, 1H); 3.11–3.08 (m, 1H); 3.00–2.84 (m, 1H); 1.25–1.22 (dd, J1= 4 Hz, J2= 6.4 Hz, 6H). 13C NMR (100 MHz, DMSO-d6) δ: 167.2; 155.8; 136.17; 133.6; 131.3; 130.8; 129.9; 126.2; 125.2; 81.9; 67.9; 49.8; 45.9; 35.9; 18.9; 18.2.
General procedure for the synthesis of cyclohexyl- isoxazolidinyl oxirane 27b or chlorophenyl isoxazolidinyl oxiranes 27e and 27f
The proper aldoxime 24b, 24e or 24f (1 eq) was added to a stirred mixture of N-chlorosuccinimide (2 eq) in anhydrous CHCl3 and pyridine at room temperature. After 15 min the reaction mixture was treated with an excess of 1,3-butadiene (3 eq) at 0 °C, and successively a solution of Et3N (1.5 eq) in dry CHCl3 was added dropwise and stirred for 3 h. Then the reaction mixture was washed with water and brine and evaporated to give a crude oil of the alkenes 26b, 26d or 26e, used directly in the next step without further purification.
A stirred mixture of the resulting crude alkene 26b, 26 d, or 26e (1 eq) and NaHCO3, (2 eq) in anhydrous CH2Cl2 was cooled to 0 °C and a solution of 70% m-chloroperoxybenzoic acid (2 eq) in anhydrous CH2Cl2 was added dropwise. The mixture was stirred under a nitrogen atmosphere at room temperature for 72 h and then filtered. The resulting solution was washed with 3% of aqueous K2CO3, Na2S2O3 1 N and brine, dried with Na2SO4, and evaporated. The crude residue, consisting almost exclusively of a 1:1 mixture of the diastereomeric anti- and syn-epoxide 27b, 27e and 27f, was submitted to MPLC on silica gel, eluting with a 4:2:1 hexane/CHCl3/AcOEt mixture, isolating pure the anti and syn epoxides.
(syn)-3-cyclohexyl-5-(-oxiran-2-yl)-4,5-dihydroisoxazole (27b): (47% from oxime 24b) 1H NMR (400 MHz, CDCl3): 4.67–4.54 (1H, m), 3.74–3.68 (m,1H), 3.02–2.73 (2H, m), 2,77–2,75 (m, 2H), (1.81–1.64 (6H, m), 1.34–1.20 (5H, m).
3-(4-chloro-2-methoxyphenyl)-5-(-oxiran-2-yl)-4,5-dihydroisoxazole (27f): (27f, anti): (41% from oxime 24f). 1H NMR (400 MHz, CDCl3): 7.70–7.69 (m, 1H); 7.63–7.61 (m, 1H); 7.25–7.23 (m, 1H); 4.44 (dddd, J = 4.2 Hz, J = 7.6 Hz, J = 9.0 Hz, 1H); 3.3-2.75 (m, 4H), 2.62 (dd, J = 2.3 Hz, J = 4.2 Hz, 1H); (27f, syn): (35% from oxime 24f). 1H NMR (400 MHz, CDCl3): 7.70–7.69 (m, 1H); 7.63–7.61 (m, 1H); 7.25–7.23 (m, 1H); 4.44 (dddd, J = 4.2 Hz, J = 7.6 Hz, J = 9.0 Hz, 1H); 3.38-2.93 (m, 3H), 2.65 (d, J = 3.8 Hz, 2H)
3-(2-chloro-4-methoxyphenyl)-5-(oxiran-2-yl)-4,5-dihydroisoxazole (27e): (27e, anti), (40% from oxime 23e); 1H NMR (400 MHz, CDCl3): 7.70–7.69 (m, 1H); 7.63–7.61 (m, 1H); 7.25–7.23 (m, 1H); 4.48 (dddd, J = 4.6 Hz, J = 8.1 Hz, J = 10.0 Hz, 1H), 3.32–2.72 (m, 4H), 2.65 (dd, J = 2.8 Hz, J = 4.0 Hz, 1H); (27e, sin) (40% from oxime 23f); 1H NMR (400 MHz, CDCl3): 7.70–7.69 (m, 1H); 7.63–7.61 (m, 1H); 7.25–7.23 (m, 1H); 4.52 (dddd, J = 4.2 Hz, J = 7.9 Hz J = 9.8 Hz, 1H), 3.28–2.89 (m, 3H), 2.70 (d, J = 3.9 Hz, 2H)
Synthesis of cyclohexyl-isoxazolidinyl-N-alkyl-ethanolamines 6 and 7
A solution of syn epoxide 27b (1 eq) and i-PrNH2, or t-BuNH2 (4.6 eq) in a mixture of 1:2 anhydrous benzene/EtOH was stirred at room temperature for 72 h, and then evaporated to dryness. The crude oily residue was taken up in 10% aqueous HCl and brine, and the resulting mixture was washed with Et2O, alkalinised with solid K2CO3, and then extracted with CHCl3. The organic phases were collected and evaporated and the residue was dissolved in diethyl ether and treated with a small excess of oxalic acid. The oxalic salts 6 and 7, have been obtained pure by crystallisation process in a 4:1 Et2O/EtOH mixture.
(syn)-1-(3-cyclohexyl-4,5-dihydroisoxazol-5-yl)-2-(isopropylamino)ethanol oxalate (6) (75% yield), m.p.: 124–126 °C. 1H NMR (400 MHz, DMSO-d6) δ: 8.65 (br s, 1H); 4.38–4.32 (m, 1H); 3.76–3.72 (m, 1H); 3.33–3.27 (m, 1H); 3.02–2.96 (m, 3H); 2.81–2.71 (m, 1H); 2.37–2.35 (m, 1H); 1.79–1.61 (m, 5H); 1.29–1.22 (m, 11H). 13C NMR (100 MHz, DMSO-d6) δ: 162.8; 80.4; 67.7; 50.3; 47.1; 37.3; 36.9; 30.4; 25.9; 25.6; 19.1; 18.6.
(syn)-2-(tert-butylamino)-1-(3-cyclohexyl-4,5-dihydroisoxazol-5-yl)ethan-1-ol oxalate (7): (67% yield) m.p.: 182–185 °C. 1H NMR (400 MHz, DMSO-d6) δ: 8.99 (bs, 1H); 8.48 (bs, 1H); 6.02–5.87 (m, 1H); 4.40–4.34 (m, 1H); 3.75–3.71 (m, 1H); 3.55–3.50 (m, 2H); 3.03–2.95 (m, 3H); 2.76–2.72 (m, 1H); 2.40–2.36 (m, 1H); 1.78–1.61 (m, 5H); 1.29–1.10 (m, 14H). 13C NMR (100 MHz, DMSO-d6) δ: 162.3; 79.8; 67.5; 56.3; 44.0; 36.8; 36.4; 29.9; 25.5; 25.2; 24.9.
General procedure for the synthesis of chloro-methoxyphenyl-isoxazolidinyl-N-alkyl-ethanolamines 16-21
A solution of the proper syn or anti epoxide 27e or 27f (1 eq) and i-PrNH2, or t-BuNH2 (4.6 eq) in a mixture of 1:2 anhydrous benzene/EtOH was stirred at room temperature for 72 h, and then evaporated to dryness. The crude oily residue was taken up in 10% aqueous HCl and brine, and the resulting mixture was washed with Et2O, alkalinised with solid K2CO3, and then extracted with CHCl3. The organic phases were collected and evaporated and the residue was dissolved in diethyl ether and treated with a small excess of proper organic acid. The isoxazolidinyl-N-alkyl-ethanolamine salts 16–21, have been obtained pure by crystallisation process in a 4:1 Et2O/EtOH mixture.
(syn)-1-(3-(2-chloro-4-methoxyphenyl)-4,5-dihydroisoxazol-5-yl)-2-(isopropylamino) ethan-1-ol maleate (16): (73% yield) m.p0.183–184 °C. 1H NMR (400 MHz, DMSO-d6) δ: 8.32 (bs, 2H); 7.70–7.69 (m, 1H); 7.64–7.61 (m, 1 H); 7.26–7.24 (m, 1H); 6.02 (s, 2H); 5.96 (bs, 1H); 4.66–4.60 (m, 1H); 3.91 (s, 3H); 3.83–3.81 (m, 1H); 3.49–3.40 (m, 2H); 3.09–3.07 (m, 1H); 2.89–2.84 (m, 1H); 1.24-1.21 (dd, J1= 4.4 Hz, J2= 6.4 Hz, 6H). 13C NMR (100 MHz, DMSO-d6) δ: 167.2; 155.8; 155.4; 136.1; 127.8; 126.9; 122.5; 121.5; 113.0; 81.7; 67.2; 56.4; 49.9; 46.2; 35.8; 18.9; 18.1.
(syn)-2-(tert-butylamino)-1-(3-(2-chloro-4-methoxyphenyl)-4,5-dihydroisoxazol-5-yl) ethan-1-ol oxalate (17): (67% yield) m.p: 210–212 °C. 1H NMR (400 MHz, DMSO-d6) δ: 7.72–7.71 (m, 1H); 7.65–7.62 (m, 1H); 7.26–7.24 (m, 1H); 4.69–4.64 (m, 1H); 3.91 (s, 3H); 3.87–3.83 (m, 1H); 3.49–3.39 (m, 2H); 3.09–3.06 (m, 1H); 2.84–2.78 (m, 1H); 1.29 (s, 9H). 13C NMR (100 MHz, DMSO-d6) δ: 165.3; 156.3; 155.9; 128.3; 127.4; 123.0; 121.9; 113.5; 82.2; 67.9; 56.9; 56.7; 44.1; 36.2; 25.5.
(anti)-2-(tert-butylamino)-1-(3-(2-chloro-4-methoxyphenyl)-4,5-dihydroisoxazol-5-yl) ethan-1-ol oxalate (18) (65% yield) m.p.: 205–207 °C. 1HNMR (400 MHz, DMSO-d6) δ: 7.70–7.69 (m, 1H); 7.63–7.61 (m, 1H); 7.25–7.23 (m, 1H); 4.71–4.63 (m, 1H); 3.91 (s, 3H); 3.67–3.65 (m, 1H); 3.43 (dd, J1= 10.8 Hz, J2= 16.8 Hz, 1H); 3.30 (dd, J1= 8.8 Hz, J2= 17.2 Hz, 1H); 2.87–2.83 (m, 1H); 2.77-2.63 (m, 1H); 1.17 (s, 9H). 13C NMR (100 MHz, DMSO-d6) δ: 167.2; 155.8; 155.4; 136.2; 127.8; 126.9; 122.6; 121.5; 113.1; 81.6; 68.3; 56.5; 56.4; 43.3; 36.2; 25.1.
(syn)-1-(3-(2-chloro-4-methoxyphenyl)-4,5-dihydroisoxazol-5-yl)-2-(isopropylamino) ethan-1-ol maleate (19) (85% yield) m.p.:140–141 °C. 1H NMR (400 MHz, DMSO-d6) δ: 8.31 (bs, 2H); 7.56–7.50 (m, 2H); 7.20–7.18 (m, 1H); 6.01 (s, 2H); 5.97–5.95 (m, 1H); 4.63–4.57 (m, 1H); 3.85 (s, 3H); 3.82–3.77 (m, 1H); 3.47–3.40 (m, 2H); 3.09–3.06 (m, 1H); 2.89–2.83 (m, 1H); 1.24–1.22 (dd, J1= 2 Hz, J2= 3.6 Hz, 6H). 13C NMR (100 MHz, DMSO-d6) δ: 167.6; 156.6; 155.3; 136.6; 131.4; 128.4; 124.8; 120.2; 114.8; 82.2; 68.9; 56.7; 50.3; 38.6; 19.4; 18.6.
(anti)1-(3-(4-chloro-2-methoxyphenyl)-4,5-dihydroisoxazol-5-yl)-2-(isopropylamino) ethan-1-ol maleate (20) (80% yield) m.p.:180–181 °C. 1H NMR (400 MHz, DMSO-d6) δ: 8.63 (br s, 2H); 7.71–7.70 (m, 1H); 7.70–7.48 (m, 1H); 7.19–7.17 (m, 1H); 6.02 (s, 2H); 5.92 (br s, 1H); 5.86–5.85 (m, 1H); 4.70–4.64 (m, 1H); 3.91–3.90 (m, 1H); 3.84 (s, 3H); 3.55–3.47 (m, 1H); 3.39–3.30 (m, 1H); 3.06–2.93 (m,1H); 1.24 (m, 6H). 13C NMR (100 MHz, DMSO-d6) δ: 164.9; 156.6; 155.3; 131.4; 128.4; 124.8; 120.3; 114.7; 82.2; 68.0; 56.7; 44.1; 38.5; 31.2; 25.6.
(sin)-2-(tert-butylamino)-1-(3-(4-chloro-2-methoxyphenyl)-4,5-dihydroisoxazol-5-yl) ethan-1-ol maleate (21) (70%yield) m.p: 163–164 °C. 1H NMR (400 MHz, DMSO-d6) δ: 8.31 (bs, 2H); 7.56–7.55 (m, 1H); 7.52–7.49 (m, 1H); 7.20–7.18 (m, 1H); 6.02 (s, 2H); 5.97 (bs, 1H); 4.66–4.60 (m, 1H); 3.84 (s, 3H); 3.83–3.81 (m, 1H); 3.48–3.43 (m, 2H); 3.35–3.34 (m, 1H); 3.08–3.05 (m, 1H); 2.83–2.77 (m, 1H); 1.29 (s, 9H). 13C NMR (100 MHz, DMSO-d6) δ: 167.2; 156.2; 154.9; 136.1; 131.0; 128.0; 124.3; 119.8; 114.3; 81.7; 67.5; 56.5; 56.3; 43.5; 38.0; 30.7; 25.0.
Carbonic anhydrase activation
A Stopped Flow methodCitation38 has been used for assaying the CA-catalysed CO2 hydration activity with Phenol red as an indicator, working at the absorbance maximum of 557 nm, following the initial rates of the CA-catalysed CO2 hydration reaction for 10–100 s. For each activator, at least six traces of the initial 5–10% of the reaction have been used for determining the initial velocity. The uncatalyzed rates were determined in the same manner and subtracted from the total observed rates. Stock solutions of activator (0.1 mM) were prepared in distilled-deionized water and dilutions up to 0.1 nM were done thereafter with the assay buffer. The activation constant (KA), defined similarly with the inhibition constant (KI), was obtained by considering the classical Michaelis–Menten equation [EquationEquation (3)(3)
(3) ], which has been fitted by non-linear least squares by using PRISM 3:
(3)
(3)
where [A]f is the free concentration of the activator
Working at substrate concentrations considerably lower than KM ([S]≪KM), and considering that [A]f can be represented in the form of the total concentration of the enzyme ([E]t) and activator ([A]t), the obtained competitive steady-state equation for determining the activation constant is given by EquationEquation(4)(4)
(4) :
(4)
(4)
where v0 represents the initial velocity of the enzyme-catalysed reaction in the absence of an activator. Enzyme concentrations in the assay system were in the range of 7.0–16.0 nM.
Results and discussion
Chemistry
As mentioned in the introduction, compounds 2–5, 8–15 and 22–23 () have been prepared according to literature procedures. The same reported procedure was applied to synthesise the new compounds 6–7, 16–21, as reported in Scheme 1.
Scheme 1. Reactions and conditions: (i) N-Chlorosuccinimide, DMF 15 min, rt; (ii) Et3N, 1,3-butadiene, CHCl3, 0 °C-rt, 3 h; (iii) m-Cl-perbenzoic acid, DCM, 0 °C-rt, 72 h; (iv) iPrNH2, tBuNH2, Benzene/EtOH, rt, 72 h.

The chlorination of the proper aldoximes 24a–d, g–i, conducted by treatment with N-chlorosuccinimide in DMF, afforded the corresponding hydroxamyl chlorides 25a–d and g–i. For the methoxy phenyl aldoximes 24h and 24i, an unexpected electrophilic addition of chlorine on the o- or p- aromatic position concerning the methoxy moiety, occurred. Probably, chlorine overreaction was due to the improved susceptibility of the p- or o-OMe substitution in the aromatic ring to the initial chlorination process. In fact, the substitution of a chlorine atom on the aromatic ring is easily promoted by the presence of a methoxy group in a general electrophilic aromatic substitution. Nevertheless, the synthesis was completed and the p- and o- methoxy chlorophenyl hydroxamyl chlorides 25e–f were used in the following reaction steps. The treatment by triethylamine and 1,3-butadiene of the proper hydroxamyl chlorides 25a–g, afforded the corresponding vinyl-2-isoxazolines 26a–g. The following oxidation of isoxazolines 26a–g with m-chloroperoxybenzoic acid yielded a mixture of anti and/or syn 27a–g epoxides which were separated by flash chromatography. Thus, aminolysis conducted by treatment of epoxides 27a–g with an excess of i-PrNH2 or t-BuNH2 yielded the corresponding anti and/or syn 2–23 amino alcohols purified by crystallization as organic salts (maleate, fumarate or oxalate).
The anti or syn configurations have been assigned by 1H NMR comparison with the already published compounds, deeply characterized by NMR and crystallographic studies. Moreover, by analyzing the 1H NMR and the bi-dimensional COSY spectra, it was possible to detect how the difference in the configuration affected the Δ chemical shift of diastereotopic vicinal protons of the hydroxyl group (OH–CH–CH2–NH–, protons D, ). Generally, each proton of methylene D (HD1 and HD2) presented a chemical shift sparing from 3.2 to 3.6 ppm, characterized by a double doublet signal, due to the couplings HD1-HD2 and HD-C. Interestingly, in the anti configuration two separated D proton double doublets with ΔHD1-HD2 of 0.20 ppm were detected at 3.60 and 3.40 ppm (). Otherwise, in the syn configuration a superimposition of the two D proton double doublet signals with a consequent ΔHD1-HD2=0 was evident (). This difference in Δ chemical shift is probably due to the different coupling between C and D protons caused by the different configurations.
Carbonic anhydrase activation
The novel isoxazoline-based amino alcohol series (2–23) has been tested on four physiologically relevant hCAs (hCA I, hCA II, hCA IV and hCA VII), mainly expressed in the human brain. Focussing on the central nervous system (CNS), the cytosolic hCA I is mainly expressed by motoneurons, sited in the human spinal cordCitation39, hCA II is located both in the choroid plexus, and in oligodendrocytes, myelinated tracts, astrocytes and myelin sheaths and hCA IV is expressed on the luminal surface of cerebral capillaries, associated with the blood-brain barrier, and in a different area of the cortex, thalamus and hippocampusCitation40,Citation41. The membrane-associated hCA VII might be considered the brain-target CA, because is predominantly expressed in the brain, in the cortex, thalamus and hippocampus, while it is almost absent in the other human tissuesCitation42.
The CA activation data of human brain CA isoforms (hCA I, hCA II, hCA IV and hCA VII), with isoxazoline-based amino alcohols (2–23) are reported in . Histamine has been tested as a reference compound. In general, all the new CA activators presented activity towards hCA II, hCA IV and hCA VII in the low micromolar range, and poor activity towards hCA I. The data evidenced no significant differences between tert-butyl and isopropyl derivatives for the activation of all the hCA isozymes, thus indicating a minor importance of the R1 amino substituent in determining the affinity for the enzymes.
Regarding the cytosolic isoform hCA I, the activation promoted by isoxazoline amino alcohols was weak, presenting KA values ranging from 11.7 to >100 µM. In particular, a lack of hCA I activity (KAs > 100 µM) was demonstrated by the p-OMe-o-Cl phenyl isoxazoline 18 and the phenyl isoxazoline 9. Furthermore, the m-Cl phenyl-isoxazoline-isopropylamines 21 and 22 showed KAs > 100 µM for hCA I and a 2-fold decrease of activity concerning their o-Cl analogues 12 and 13. It can be noted a preferred o-chloro substitution to maintain hCA I activity.
All the compounds activated cytosolic hCA II with potency in the low micromolar range (KAs from 1.53 to 4.15 µM). hCA II was activated more than hCA I and hCA IV by all the isoxazoline amino alcohols of this new series, without any exception. Interestingly, compounds 9, 18, and 22 reported almost a 50-fold enhancement of activation efficacy of hCA II when compared to hCA I (hCA I KA >100/hCA II 1.84–2.85 µM). Importantly, this new series of compounds showed an improved activity of at least 40-fold compared to histamine (KA of 125 µM), the reference compound which is a weak activator of this isoform.
As for activity towards the membrane-associated isozyme hCA IV, all KAs were in a rather flat low micromolar range, from 4.35 to 25.5 µM values, with no KA value measured in the submicromolar range. No significant differences exist between tert-butyl and isopropyl derivatives and between different configurations, for the activation of hCA IV. Notably, the reference CAA histamine was a poorer activator of hCA IV (KA of 25.3 µM) than the tested isoxazolines, with the only exception of derivative 10 with a KA of 35.7 µM against hCA IV.
The other isoform investigated here, hCA VII, was rather potently activated by all of the compounds reported in this work (KAs in the range 0.52–8.26 µM). The most interesting data were those reported for the methyl-substituted isoxazoline derivatives 2–5, able to activate hCA VII in a submicromolar range (KA values 0.52–0.86 µM). These compounds presented also a moderate selectivity over the other tested isoforms. The small size of the methyl group for the aryl substituents of other derivatives seems to be significant to address activity and selectivity towards hCA VII isoform.
Notably, configuration affected the hCA VII activity data in terms of activity and selectivity. In particular, a slight improvement (1.3–1.7 fold) of hCA VII activity was reported for the anti-diasteroisomers 3 and 5 compared to their syn analogues 2 and 4. Moreover, compounds 3 and 5 demonstrated a significantly improved selectivity over hCA I concerning 2 and 4. In fact, syn-methyl-isoxazoline 2 presented a 13-fold selectivity hCA VII/hCAI versus the 53-fold selectivity hCA VII/hCA I of anti-methyl-isoxazoline 3, and the syn-tert-butylamine 4 showed a 15-fold selectivity hCA VII/hCA I versus the 90-fold selectivity hCA VII/hCA I of anti-tert-butylamine 5.
Overall, among all tested derivatives the best results were achieved with the methyl-substituted isoxazoline 3, showing a 72-fold higher activity for hCA VII (KA = 0.52 µM) concerning histamine and a 53-fold selectivity over hCA I.
hCA VII is one of the most representative CA expressed in the brain, and because of its predominant presence in brain tissues, selective hCA VII-targeting can be considered an area of interest in the research of more effective agents for treating neurodegeneration-related diseases.
Conclusions
In the present study, the synthesis and CA activating properties of a new series of isoxazoline-based amino alcohols towards four CAs, that are hCA I, hCA II, hCA IV and hCA VII, mainly expressed in the human brain are reported. All the tested compounds induced a consistent activation of the tested CAs, with KA value ranges spanning from low to intermediate micromolar range. All compounds showed a generally poor affinity for hCA I, and a good activity profile for hCA II and hCA VII, main targets in CNS diseases. Specifically, hCA VII targeting was achieved by the (methyl)-isoxazoline-amino alcohols 2–5 with KA values in the submicromolar range (0.52–0.86 µM), but only with compounds in anti-configuration, 3 and 5, a selectivity over hCA I was achieved. These molecules represent a new chemotype in the field of CA activators and deserve further investigation. Owing to the wide CNS expression of hCA VII, such molecules could be useful as a tool to study the physiological role of this enzyme in diseases involved in CNS such as epilepsy, ageing and neurodegeneration.
Supplemental Material
Download PDF (896.9 KB)Disclosure statement
CT Supuran is Editor-in-Chief of the Journal of Enzyme Inhibition and Medicinal Chemistry. He was not involved in the assessment, peer review, or decision-making process of this paper. The authors have no relevant affiliations or financial involvement with any organisation or entity with a financial interest in or financial conflict with the subject matter or materials discussed in the manuscript. This includes employment, consultancies, honoraria, stock ownership or options, expert testimony, grants or patents received or pending, or royalties.
Additional information
Funding
References
- Supuran CT. Structure and function of carbonic anhydrases. Biochem J. 2016;473(14):2023–2032.
- Supuran CT, Scozzafava A. Carbonic anhydrase inhibitors and their therapeutic potential. Expert Opinion on Therapeutic Patents. 2000;10(5):575–600.
- Alterio V, Di Fiore A, D'Ambrosio K, Supuran CT, De Simone G. Multiple binding modes of inhibitors to carbonic anhydrases: how to design specific drugs targeting 15 different isoforms? Chem Rev. 2012;112(8):4421–4468.
- Supuran CT. Emerging role of carbonic anhydrase inhibitors. Clin Sci. 2021;135(10):1233–1249.
- Supuran CT. Structure-based drug discovery of carbonic anhydrase inhibitors. J Enzyme Inhib Med Chem. 2012;27(6):759–772.
- Cuffaro D, Nuti E, Rossello A. An overview of carbohydrate-based carbonic anhydrase inhibitors. J Enzyme Inhib Med Chem. 2020;35(1):1906–1922.
- Masini E, Carta F, Scozzafava A, Supuran CT. Antiglaucoma carbonic anhydrase inhibitors: a patent review. Expert Opin Ther Pat. 2013;23(6):705–716.
- Ciccone L, Cerri C, Nencetti S, Orlandini E. Carbonic anhydrase inhibitors and epilepsy: state of the art and future perspectives. Molecules. 2021;26(21):6380.
- Scozzafava A, Supuran CT, Carta F. Antiobesity carbonic anhydrase inhibitors: a literature and patent review. Expert Opin Ther Pat. 2013;23(6):725–735.
- Poli G, Galati S, Martinelli A, Supuran CT, Tuccinardi T. Development of a cheminformatics platform for selectivity analyses of carbonic anhydrase inhibitors. J Enzyme Inhib Med Chem. 2020;35(1):365–371.
- Fischer T, Senn N, Riedl R. Design and structural evolution of matrix metalloproteinase inhibitors. Chemistry. 2019;25(34):7960–7980.
- Nuti E, Cuffaro D, Bernardini E, Camodeca C, Panelli L, Chaves S, Ciccone L, Tepshi L, Vera L, Orlandini E, et al. Development of thioaryl-based matrix metalloproteinase-12 inhibitors with alternative zinc-binding groups: synthesis, potentiometric, NMR, and crystallographic studies. J Med Chem. 2018;61(10):4421–4435.
- Cuffaro D, Camodeca C, D'Andrea F, Piragine E, Testai L, Calderone V, Orlandini E, Nuti E, Rossello A. Matrix metalloproteinase-12 inhibitors: synthesis, structure-activity relationships and intestinal absorption of novel sugar-based biphenylsulfonamide carboxylates. Bioorg Med Chem. 2018;26(22):5804–5815.
- Nuti E, Rossello A, Cuffaro D, Camodeca C, Van Bael J, van der Maat D, Martens E, Fiten P, Pereira RVS, Ugarte-Berzal E, et al. Bivalent inhibitor with selectivity for trimeric mmp-9 amplifies neutrophil chemotaxis and enables functional studies on mmp-9 proteoforms. Cells. 2020;9(7):1634.
- Blandina P, Provensi G, Passsani MB, Capasso C, Supuran CT. Carbonic anhydrase modulation of emotional memory. Implications for the treatment of cognitive disorders. J Enzyme Inhib Med Chem. 2020;35(1):1206–1214.
- Datta R, Shah GN, Rubbelke TS, Waheed A, Rauchman M, Goodman AG, Katze MG, Sly WS. Progressive renal injury from transgenic expression of human carbonic anhydrase IV folding mutants is enhanced by deficiency of p58IPK. Proc Natl Acad Sci USA. 2010;107(14):6448–6452.
- Shah GN, Bonapace G, Hu PY, Strisciuglio P, Sly WS. Carbonic anhydrase II deficiency syndrome (osteopetrosis with renal tubular acidosis and brain calcification): novel mutations in CA2 identified by direct sequencing expand the opportunity for genotype-phenotype correlation. Hum Mutat. 2004;24(3):272.
- van Karnebeek CD, Sly WS, Ross CJ, Salvarinova R, Yaplito-Lee J, Santra S, Shyr C, Horvath GA, Eydoux P, Lehman AM, et al. Mitochondrial carbonic anhydrase VA deficiency resulting from CA5A alterations presents with hyperammonemia in early childhood. Am J Hum Genet. 2014;94(3):453–461.
- Feinstein Y, Yerushalmi B, Loewenthal N, Alkrinawi S, Birk OS, Parvari R, Hershkovitz E. Natural history and clinical manifestations of hyponatremia and hyperchlorhidrosis due to carbonic anhydrase XII deficiency. Horm Res Paediatr. 2014;81(5):336–342.
- Boyne K, Corey DA, Zhao P, Lu B, Boron WF, Moss FJ, Kelley TJ. Carbonic anhydrase and soluble adenylate cyclase regulation of cystic fibrosis cellular phenotypes. Am J Physiol Lung Cell Mol Physiol. 2022;322(3):L333–L347.
- Canto de Souza L, Provensi G, Vullo D, Carta F, Scozzafava A, Costa A, Schmidt SD, Passani MB, Supuran CT, Blandina P. Carbonic anhydrase activation enhances object recognition memory in mice through phosphorylation of the extracellular signal-regulated kinase in the cortex and the hippocampus. Neuropharmacology. 2017; 118(118148):148–156.
- Schmidt SD, Costa A, Rani B, Godfried Nachtigall E, Passani MB, Carta F, Nocentini A, de Carvalho Myskiw J, Furini CRG, Supuran CT, et al. The role of carbonic anhydrases in extinction of contextual fear memory. Proc Natl Acad Sci USA. 2020;117(27):16000–16008.
- Wang X, Schröder HC, Schlossmacher U, Neufurth M, Feng Q, Diehl-Seifert B, Müller WEG. Modulation of the initial mineralization process of SaOS-2 cells by carbonic anhydrase activators and polyphosphate. Calcif Tissue Int. 2014;94(5):495–509.
- Sun MK, Alkon DL. Pharmacological enhancement of synaptic efficacy, spatial learning, and memory through carbonic anhydrase activation in rats. J Pharmacol Exp Ther. 2001;297(3):961–967.
- Supuran CT. Carbonic anhydrase activators. Future Med Chem. 2018;10(5):561–573.
- Elder I, Tu C, Ming L-J, McKenna R, Silverman DN. Proton transfer from exogenous donors in catalysis by human carbonic anhydrase II. Arch Biochem Biophys. 2005;437(1):106–114.
- Angeli A, Berrino E, Carradori S, Supuran CT, Cirri M, Carta F, Costantino G. Amine- and amino acid-based compounds as carbonic anhydrase activators. Molecules. 2021;26(23):7331.
- Temperini C, Scozzafava A, Vullo D, Supuran CT. Carbonic anhydrase activators. Activation of isozymes I, II, IV, VA, VII, and XIV with l- and d-histidine and crystallographic analysis of their adducts with isoform II: engineering proton-transfer processes within the active site of an enzyme. Chemistry. 2006;12(27):7057–7066.
- Provensi G, Nocentini A, Passani MB, Blandina P, Supuran CT. Activation of carbonic anhydrase isoforms involved in modulation of emotional memory and cognitive disorders with histamine agonists, antagonists and derivatives. J Enzyme Inhib Med Chem. 2021;36(1):719–726.
- Tanini D, Capperucci A, Supuran CT, Angeli A. Sulfur, selenium and tellurium containing amines act as effective carbonic anhydrase activators. Bioorg Chem. 2019; 87(87516):516–522.
- Nocentini A, Cuffaro D, Ciccone L, Orlandini E, Nencetti S, Nuti E, Rossello A, Supuran CT. Activation of carbonic anhydrases from human brain by amino alcohol oxime ethers: towards human carbonic anhydrase VII selective activators. J Enzyme Inhib Med Chem. 2021;36(1):48–57.
- Maccallini C, Di Matteo M, Vullo D, Ammazzalorso A, Carradori S, De Filippis B, Fantacuzzi M, Giampietro L, Pandolfi A, Supuran CT, et al. Indazole, pyrazole, and oxazole derivatives targeting nitric oxide synthases and carbonic anhydrases. ChemMedChem. 2016;11(16):1695–1699.
- Sugimoto A, Ikeda H, Tsukamoto H, Kihira K, Ishioka M, Hirose J, Hata T, Fujioka H, Ono Y. Timolol activates the enzyme activities of human carbonic anhydrase I and II. Biol Pharm Bull. 2010;33(2):301–306.
- Balsamo A, Breschi M, Chini M, Domiano P, Giannaccini G, Lucacchini A, Macchia B, Macchia M, Manera C, Martinelli A, et al. Conformationally restrained β-blocking oxime ethers: synthesis and β-adrenergic properties of diastereoisomeric anti and syn 2-(5′-isoxazolidinyl)-ethanolamines. Eur J Med Chem. 1992;27(8):751–764.
- Balsamo A, Breschi M, Chiellini G, Lucacchini A, Macchia M, Martinelli A, Martini C, Nardini C, Orlandini E, Romagnoli F, et al. Conformationally restrained β-blocking oxime ethers. 2. Synthesis and β-adrenergic properties of diastereoisomeric anti and syn 2-(5′-(3′-aryl-substituted)isoxazolidinyl)-N-alkylethanolamines. Eur J Med Chem . 1994;29(11):855–867.
- Breschi M, Macchia M, Manera C, Micali E, Nardini C, Nencetti S, Rossello A, Scatizzi R. Conformationally restrained β-blocking oxime ethers. 3. Synthesis and β-adrenergic antagonistic activity of diastereomeric anti and syn 2-(5′-(3′-methyl)isoxazolidinyl)-N-alkylethanolamines. Eur J Med Chem. 1996;31(2):159–163.
- Ramos BP, Colgan L, Nou E, Ovadia S, Wilson SR, Arnsten AFT. The beta-1 adrenergic antagonist, betaxolol, improves working memory performance in rats and monkeys. Biol Psychiatry. 2005;58(11):894–900.
- Khalifah RG. The carbon dioxide hydration activity of carbonic anhydrase. I. Stop-flow kinetic studies on the native human isoenzymes B and C. J Biol Chem. 1971;246(8):2561–2573.
- Liu X, Lu D, Bowser R, Liu J. Expression of carbonic anhydrase i in motor neurons and alterations in ALS. IJMS. 2016;17(11):1820.
- Halmi P, Parkkila S, Honkaniemi J. Expression of carbonic anhydrases II, IV, VII, VIII and XII in rat brain after kainic acid induced status epilepticus. Neurochem Int. 2006;48(1):24–30.
- Svichar N, Waheed A, Sly WS, Hennings JC, Hübner CA, Chesler M. Carbonic anhydrases CA4 and CA14 both enhance AE3-mediated Cl–HCO3- exchange in hippocampal neurons. J Neurosci. 2009;29(10):3252–3258.
- Ruusuvuori E, Kaila K. Carbonic anhydrases and brain pH in the control of neuronal excitability. Subcell Biochem. 2014;75:75271–75290.