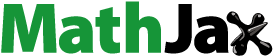
Abstract
Carbonic anhydrases (CAs) are important regulators of pH homeostasis and participate in many physiological and pathological processes. CA activators (CAAs) are becoming increasingly important in the biomedical field since enhancing CA activity may have beneficial effects at neurological level. Here, we investigate selected antihistamines, phenothiazine-based antipsychotics, and tricyclic antidepressants (TCAs) as potential activators of human CAs I, II, IV, and VII. Our findings indicate that these compounds are more effective at activating hCA II and VII compared to hCA I and IV. Overall, hCA VII was the most efficiently activated isoform, particularly by phenothiazines and TCAs. This is especially relevant since hCA VII is the most abundant isoform in the central nervous system (CNS) and is implicated in neuronal signalling and bicarbonate balance regulation. This study offers additional insights into the pharmacological profiles of clinically employed drugs and sets the ground for the development of novel optimised CAAs.
Introduction
Organisms in all kingdoms of life function in a water-based medium and rely on a plethora of biochemical transformations of the carbon element. In this context, the reversible hydration of carbon dioxide (CO2) into water-soluble bicarbonate (HCO3-) and proton ions, chemically represented by EquationEquation (1)(1)
(1) , is of utmost importance.Citation1–3
(1)
(1)
Nonetheless, at typical intracellular CO2 concentrations, this reaction is incredibly slow as CO2 is weakly soluble in water, with kcat values for the uncatalysed reaction of 0.15 s−1 and 50 s−1 for the hydration and dehydration reactions, respectively.Citation4,Citation5 Hence, the metalloenzyme superfamily of carbonic anhydrases (CAs), which catalyse the reversible hydration of CO2, has a central role in regulating cell and organism homeostasis.Citation6,Citation7 Given the nature of the catalysed reactions, CA activity is pivotal for controlling pH and maintaining the acid-base balance, thereby being involved in the metabolism regulation under both physiological and pathological conditions.Citation8–10
The exceptional physiological importance of these enzymes is testified by the existence of eight different genetic families (α-, β-, γ-, δ-, ζ-, η-, θ-, and ι-CAs) and the multitude of isoforms present in each of them, which represents one of the most dramatic instances of convergent evolution in biology.Citation4,Citation11–14 In vertebrates, at least 16 CA isoforms are known, all belonging to the α-CA family, and 15 different CAs are expressed in humans, 12 of which are catalytically active.Citation2 The fundamental differences between the different α-CA isoforms are mostly linked to their secondary and tertiary structures which influence their chemical and physical stability. The catalytic site, which is shared by all family members, comprises a Zn2+ ion coordinated by three histidine residues and a H2O/OH- molecule.Citation2
The catalytically active human CAs (hCAs) are distinct based on their subcellular localisation: hCA I-III, VII, and XIII are cytosolic;Citation15,Citation16 hCA IV is membrane-anchored via a glycosylphosphatiydyl-inositol and faces the extracellular space;Citation17,Citation18 hCA VA and VB are mitochondrial;Citation19 hCA VI is secreted (in saliva and tears);Citation20,Citation21 hCA IX, XII, and XIV are transmembrane enzymes.Citation22,Citation23 Over the years, numerous studies have identified hCAs as drug targets and many CA inhibitors are of pharmacological interest for the treatment of epilepsy, oedema, glaucoma, obesity, and cancer.Citation6,Citation24–27hCAs are widely distributed in human tissues, and many of them are highly expressed in the central nervous system (CNS) and choroid plexus.Citation7,Citation8,Citation16 Regarding the cytosolic CAs, the only one absent in the CNS is hCA XIII. The other cytosolic isoforms are all present in the CNS, with hCA VII, localised in the cortex, thalamus, and hippocampus, being the most abundant one.Citation9 The membrane-anchored hCA IV and the transmembrane isoform hCA XIV are also expressed in many regions of the CNS, with hCA IV having the same expression pattern as hCA VII.Citation28,Citation29 Differently, the transmembrane proteins hCA IX and XII are hypoxia-induced enzymes that have been found overexpressed in numerous brain cancers such as glioblastoma multiforme.Citation30–32 Finally, mitochondrial hCA VA and VB are present in the CNS, with VA being originally identified in neurons and astrocytes.Citation33–35
Ever since the resolution of first X-ray crystal structure of a CA bound to a CA activator (CAA),Citation36 which revealed their mode of action, the interest in CAAs has been constantly increasing. To date, the identified CAAs comprise amino acids, oligopeptides, biogenic amines such as histamine, serotonin, and catecholamines, and their derivatives.Citation5 The first CA-CAA cocrystal structure showed the binding mode of histamine (1) to hCA II () and indicated that CAAs bind at the entrance of the CA catalytic site.Citation36 Here, CAAs facilitate the rate-limiting step of the catalytic cycle consisting of the proton transfer process from the Zn2+-coordinated water molecule to the external reaction medium (). In the apo protein, the residue responsible for proton transfer is histidine (His64 in hCA I, II and many other α-CAs), whose pKa is ∼7, thereby being able to shuttle protons in a pH range of 6–8.Citation37 Thus, following the formation of the enzyme-substrate-activator ternary complex as a consequence of CAA binding into a region close to His64, the proton transfer process to the medium becomes intramolecular, thus being more efficient than the intermolecular transfer from the enzyme to the solvent which is not stably bound inside the enzyme cleft [see EquationEquation (2)(2)
(2) ].
(2)
(2)
Figure 1. (A) Co-crystal structure of hCA II (yellow) in complex with histamine (1, shown in green) (PDB 1AVN).Citation36 (B) Focus on the hydrogen bond network linking the Zn2+-bound water molecule to 1 (PDB 1AVN). (C) Schematic of CA activation mechanism for histamine-like compounds binding to the activator binding site A. Histamine (or analogous CAAs) bind to the activator binding site A and, through their PSM, facilitate proton transfer from Zn2+-bound H2O to the external medium. (D) Co-crystal structure of hCA II (yellow) in complex with D-Trp (shown in light blue) bound to (PDB 3EFI).Citation40 (E) Focus on the hydrogen bond network linking the Zn2+-bound water molecule to D-Trp (PDB 3EFI). (F) Schematic of CA activation mechanism for D-Trp-like compounds binding to the activator binding site B. D-Trp (or analogous CAAs) bind to the activator binding site B and, through their PSM, facilitate proton transfer from Zn2+-bound H2O to the medium. In panels (A), (B), (D), and (E) the Zn2+ atom is shown as a black sphere, water molecules are displayed as red spheres, and polar interactions are depicted in purple.

Ever since 1 has been co-crystallised with hCA II, many other CAAs have been identified, and different co-crystal structures of hCA I/II in complex with CAAs have been reported, including those with L- and D-His,Citation38 L- and D-Phe,Citation39 D-Trp (),Citation40 L-adrenaline,Citation41 and pyridinium-based analogues of histamine.Citation42 All CAAs possess proton shuttling moieties (PSMs) that are capable of binding and transferring protons from the enzyme to the external medium. They all bind to the so-called activator binding site A (), except for D-Trp, which binds to another region (activator binding site B), although its PSMs (both the amino and carboxylic acid portions) are located close to the activator binding site A ().Citation5,Citation40
From a therapeutic point of view, the primary applications of CAAs are in the fields of pharmacological augmentation of synaptic efficiency, spatial learning, and memory.Citation43 Indeed, hCA activity was shown to be decreased in Alzheimer’s disease (AD) patients.Citation44 Moreover, hCA activation was shown to increase memory performance by enhancing the ERK pathways, whose activation in the cortex and hippocampus is known to cause structural synaptic modifications that promote memory storage.Citation45–47 Hence, CAAs may find therapeutic application in the treatment of post-traumatic stress disorders (PTSD), phobias, anxiety, and memory-related disorders linked to ageing and neurodegenerative diseases.Citation48
In line with the importance of hCA activation in the CNS, many psychoactive drugs and therapeutically used histamine receptor modulators, including antihistamines, all of which bear potential PSMs (i.e. protonable moieties such as imidazole, amine, guanidine or its bioisosteres), have been recently indicated to also act as CAAs.Citation49,Citation50 Similarly, selective serotonin reuptake inhibitors antidepressants such as fluoxetine, sertraline, and citalopram have been shown to act as hCAI and II activators.Citation51 In contrast, no antipsychotic drugs have been shown to activate CAs, with the atypical antipsychotics sulpiride and aripiprazole actually inhibiting hCAI/II and hCAI/II/IV, respectively.Citation52,Citation53 Overall, these data suggest that the therapeutic and/or adverse effects of many marketed drugs may be linked to a polypharmacology-based mode of action.
Nonetheless, there are still many drugs acting on the CNS and possessing protonable moieties that have not been evaluated yet for their hCA activation potential. These include the H1 receptor (H1R) inverse agonists diphenhydramine (2),Citation54 the nicotinic receptor antagonist adiphenine (3),Citation55 the phenothiazine-based H1R inverse agonists fenethazine (4) and promethazine (5),Citation54 its bis desmethyl analogue 6, the phenothiazine-based neuroleptic drugs promazine (7), 9–12,Citation56 the promazine desmethyl metabolite 8,Citation57,Citation58 and the tricyclic antidepressants (TCA) 13–18 ().Citation59
Hence, through this study, we investigated the potential of such derivatives as activators of four pharmacologically significant CA isoforms: the cytosolic hCA I, II, and VII, and the membrane-anchored hCA IV.
Materials and methods
Chemistry
Compounds 1–3, 5, 7, and 9–18 were commercially available, highest purity reagents from Sigma-Aldrich (Milan, Italy). Compounds 4, 6, and 8 were commercially available, highest purity derivatives from Ambinter SARL, Hong Kong Chemhere Co. Ltd, and Enamine Ltd, respectively.
Carbonic anhydrase activation
The CA-catalysed CO2 hydration was monitored via a stopped-flow methodCitation60 using Phenol red as indicator and executing the measurements at the maximum absorbance of 557 nm, monitoring the initial rates of the CA-catalysed CO2 hydration reaction for 10 to 100 s. For every compound, at least six traces of the initial 5–10% of the reaction were employed for establishing the initial velocity. The uncatalysed rates were measured with the same approach and subtracted from the total rates. Stock solutions of each compound (0.1 mM) were prepared in distilled-deionised water and dilutions up to 0.1 nM were prepared using the assay buffer. The activation constant (KA), defined in a similar way as the inhibition constant (KI), was calculated using the classical Michaelis–Menten [EquationEquation (3)(3)
(3) ], which was fitted using nonlinear least squares employing GraphPad Prism 3:
(3)
(3)
where [A]f is the free concentration of the potential activator.
Since the assays were carried out at substrate concentrations significantly lower than the KM ([S] ≪ KM), and because [A]f can be defined in the form of the total concentration of the enzyme ([E]t) and activator ([A]t), the obtained competitive steady-state equation for calculating the activation constant is given by EquationEquation (4)(4)
(4) :
(4)
(4)
where v0 represents the initial velocity of the enzyme-catalysed reaction in the absence of activator. All CA isoforms used in the experiments were purified recombinant proteins obtained as already reported.Citation61–65 Enzyme concentrations in each assay were in the range of 7.0 − 12.0 nM.
Results and discussion
As previously mentioned, histamine (1) was among the first uncovered CAAs, and histamine receptor modulators have been recently investigated for their potential as CAAs.Citation59 Hence, we set out to assess the influence on hCA activity of yet unexplored antihistamines, such as the benzhydryloxyethylamine derivative 2 and phenothiazine derivatives 4 and 5. We also evaluated the anticholinergic compound 3, possessing a scaffold similar to 2, but with an additional carbonyl spacer between the oxyethylamine and benzhydryl moieties, and compound 6, the bis desmethyl derivative of 5. Given the phenothiazine-based structure of the first-generation H1 antihistamines 4 and 5, we went on to evaluate the CA activation potential of the phenothiazine-based neuroleptics 7, and 9–12, as well as the desmethyl metabolite of 7, compound 8.Citation57,Citation58 Finally, we also analysed TCAs 13–18, structurally characterised by an expansion of the central ring of phenothiazines from a 6-membered to a 7-membered cycle (). All the assayed compounds possess groups that may in principle act as PSM in the pH range 6–8, which represents an essential feature for CAAs.Citation37,Citation66
Based on the results obtained from the stopped-flow CO2 hydrase assay on the four hCA isoforms I, II, IV and VII (), the following observations may be drawn:
Table 1. hCA I, II, IV and VII activation with compounds 2–18 by a stopped-flow CO2 hydrase assay. Histamine (1) used as reference CAA.
Only four compounds exhibited cytosolic hCA I activation (KA < 50 µM) and none of them outperformed 1 in increasing hCA I activity, with the most potent ones being 3 (KA = 10.2 µM) and 13 (KA = 9.3 µM). The demethylation of 13 decreased hCA I activation potency, as demonstrated by the KA value measured for the mono desmethyl analogue 14, which is >2.5-fold higher (KA = 24.8 µM).
The cytosolic hCA II was activated by all tested compounds, apart from 2. Specifically, all compounds exhibited improved KA values over 1 for this isoform. Phenothiazines 6–10, and TCAs 13 and 14 displayed KA values lower than 5 µM, with norpromazine (8) being the most potent hCA II activator (KA = 1.4 µM), immediately followed by triflupromazine (10) and imipramine (13), with KA values of 1.9 and 2.0 µM, respectively. Notably, demethylation had divergent effects on hCA II activation for 13 and the rest of the assayed compounds. Indeed, the double demethylation of 5 and the mono demethylation of 7, leading to 6 and 8, respectively, increased the hCA II activation profile by 1.5- to 2.6-fold. Similarly, removal of a methyl group from 15 augmented hCA II activation [KA(15) = 18.9 µM, KA(16) = 11.7 µM], while the opposite effect was observed in the case of 13, since its mono desmethyl derivative 14 was ∼2-fold less potent.
The membrane-anchored hCA IV displayed an activation profile analogous to that observed for hCA I, with only few molecules capable of enhancing its activity. The only compound exhibiting a hCA IV activation more efficient than 1 was 14, with a KA value of 10.0 µM. Notably, in this case, methylation of the exocyclic nitrogen decreased CA activation potency, with 13 showing a ∼2.8-fold higher KA. The same pattern could be observed for phenothiazines 7 and 8, with the mono desmethyl derivative 8 having a KA value of 27.6 µM, while 7 was essentially not active (KA > 50 µM).
The cytosolic hCA VII was efficiently activated by the compounds investigated in the study, and all of them possessed an improved activation profile compared to 1, with a wide range of derivatives being active in the low micromolar range. Specifically, half of the assayed compounds exhibited a KA value lower than 5 µM. These include: (i) the benzhydryl derivatives 2 and 3; (ii) the phenothiazine-based compounds 7, 10, 11, and 12; (iii) the TCAs 13, 14, and 17. Among the tested molecules, the most promising hCA VII activator was desipramine 14 (KA = 1.6 µM), the mono desmethyl analogue of 13, which could still activate hCA VII, albeit almost 2-fold less efficiently. The same structure-activity relationship (SAR) was observed for TCAs 15 and 16, with the mono desmethyl derivative being >2-fold more potent than its parent compound 15. Conversely, the phenothiazines bearing a 1,3-diaminopropane chain (7 and 8) followed the opposite trend, with 7 being ∼3.5-fold more potent than the mono desmethyl analogue 8. In the case of the phenothiazine-based 1,2-diaminopropane compounds 5 and 6, removal of both N-methyl groups led to a 3-fold decrease in CA VII activation potency. Interestingly, compound 2, which was inactive towards all other isoforms, was a good hCA VII activator (KA = 4.7 µM). Similarly, TCAs 17 and 18, which were only weakly potent towards hCA II, could both activate hCA VII with KA values of 4.9 and 9.2 µM, respectively.
By looking at the different chemical classes of the analysed compounds, we could also draw some interesting SAR:
The benzhydryl compounds 2 and 3 preferentially activated hCA VII, with 2 being hCA VII-selective, while 3 was one of the few pan-hCA activators among the assayed compounds.
Both phenothiazine-based antihistamines (4, 5, and the bis desmethyl derivative 6) and neuroleptics (7–12) displayed selective hCA II/VII activation properties. Specifically, compound 7 and its derivatives 9 and 10, possessing an electron-withdrawing moiety at C2 of the phenothiazine core, displayed KA values in the low micromolar range for both hCA II and VII. In this series, compounds 4 and 5 and, partly, 6 were slightly less potent towards both isoforms, and this may be ascribed to the shorter 2-carbon spacer between the endocyclic and exocyclic nitrogen atoms. Moreover, 11 and 12 suffered from a significant drop in hCA II activation compared to analogues 7–10. In this case, the only difference between the tested compounds is the presence of a bulkier substituent at C2 of the phenothiazine core in 11 and 12.
TCAs were endowed with a similar activation pattern to phenothiazines, with all compounds preferentially activating hCA II and VII. In this case, while compounds 15-18 were selective for hCA II/VII, 13 and its mono desmethyl derivative 14 displayed a non-selective activation profile, with KA values of 10 µM or less towards hCA I and hCA IV, respectively. Although being less selective, 13 and 14 were the most potent activators of hCA II and VII in this series. Indeed, modification of some key structural features led to a drop in potency, particularly emphasised in the case of hCA II. According to the data, replacement of the endocyclic electron-rich nitrogen with a sp2 carbon and the consequent generation of a 5-exocyclic double bond (compounds 15, 16, 18) was detrimental for compound activity. Moreover, in compounds 17 and 18, the ethylene bridge between the two phenyl rings was replaced by a double bond or by inserting an oxygen atom, and this further modification especially affected their hCA II activation potency. As a result, the 10,11-dihydro-5H-dibenzo[b,f]azepine core represented the preferred chemotype for hCA II/VII activation, despite its lack of selectivity. On the other hand, the 5H-dibenzo[a,d][7]annulene core offered a fair compromise between hCA activation and selectivity, as exemplified by compound 17, the most hCA VII-selective activator among the tested TCAs.
Finally, as illustrated in the "Literature Data" section of , most of the examined compounds have been shown to cross the blood-brain barrier (BBB) and have CNS effects. As a result, hCA activation in the CNS may contribute to their pharmacological activity.
Conclusions
In this study, we examined the hCA activating properties of a variety of clinically-approved drugs (or derivatives thereof, ) known to act at CNS level such as antihistamines, neuroleptics, or antidepressants. The compounds have been evaluated on four hCA isoforms expressed in the CNS, namely hCA I, II, IV, and VII. All the assessed compounds displayed activation of at least one hCA isoform, which is consistent with the fact that they all include portions that may act as PSM at pH values ranging from 6 to 8. The compound series displayed lower activation of hCA I and IV while being generally more efficient in activating hCA II and VII. Specifically, the benzhydryl amines 2 and 3 displayed preferential activation towards hCA VII, while both phenothiazine-based compounds and TCAs could activate both hCA II and VII, with an overall slight preference of phenothiazines towards hCA II. Among the tested compounds, the phenothiazine derivative promazine (7) displayed the best profile in terms of potency and hCA II/VII selectivity over the other hCA isoforms. TCAs 13 and 14, on the other hand, were essentially non-selective, and modifications to their 5H-dibenzo[b,f]azepine core, although improving the selectivity towards hCA VII, lowered compound potency, as evidenced by the activity profiles of 17 and 18.
Remarkably, hCA VII was overall the most efficiently activated isoform by the compounds evaluated in our analysis. This is of significant relevance since hCA VII is the most abundant CA in the human CNS9 and is involved in the maintenance of the bicarbonate gradient in neurons as well as neuronal signalling.Citation7 It is therefore of great interest that all tested compounds, belonging to a diverse subset of chemical and pharmacological classes, were identified as activators of hCA VII. These molecules may serve as lead compounds for drug discovery campaigns aiming at developing novel CNS-specific CAAs.
Furthermore, the evidence collected through this study offers additional insights into the pharmacological profile and therapeutic effects of clinically employed drugs such as antihistamines, phenothiazine-based antipsychotics, and TCAs. Indeed, both their therapeutic activity and adverse effects are connected to polypharmacology-based mechanisms, and the enhancement of hCA activity should now be considered. Ultimately, the present research may provide novel perspectives into the complex network linking hCA function and CNS homeostasis. Indeed, while CAAs have only recently been considered for their pharmacological applications in memory and cognition therapy,Citation43,Citation45,Citation47,Citation48 their potential is undeniable, and research aimed at investigating hCA activation at central level is critical for the development of new drugs with possible applications in neurodegeneration.
Disclosure statement
No potential conflict of interest was reported by all authors except CTS. CT Supuran is Editor-in-Chief of the Journal of Enzyme Inhibition and Medicinal Chemistry. He was not involved in the assessment, peer review, or decision-making process of this paper. The authors have no relevant affiliations of financial involvement with any organisation or entity with a financial interest in or financial conflict with the subject matter or materials discussed in the manuscript. This includes employment, consultancies, honoraria, stock ownership or options, expert testimony, grants or patents received or pending, or royalties.
Additional information
Funding
References
- Supuran CT. How many carbonic anhydrase inhibition mechanisms exist? J Enzyme Inhib Med Chem. 2016;31(3):345–360.
- Alterio V, Di Fiore A, D'Ambrosio K, Supuran CT, De Simone G. Multiple binding modes of inhibitors to carbonic anhydrases: how to design specific drugs targeting 15 different isoforms? Chem Rev. 2012;112(8):4421–4468.
- Supuran CT. Exploring the multiple binding modes of inhibitors to carbonic anhydrases for novel drug discovery. Expert Opin Drug Discov. 2020;15(6):671–686.
- Supuran CT. Carbonic anhydrases: novel therapeutic applications for inhibitors and activators. Nat Rev Drug Discov. 2008;7(2):168–181.
- Angeli A, Berrino E, Carradori S, Supuran CT, Cirri M, Carta F, Costantino G. Amine- and amino acid-based compounds as carbonic anhydrase activators. Molecules. 2021;26(23):7331.
- Supuran CT, Altamimi ASA, Carta F. Carbonic anhydrase inhibition and the management of glaucoma: a literature and patent review 2013-2019. Expert Opin Ther Pat. 2019;29(10):781–792.
- Nocentini A, Donald WA, Supuran CT. Chapter 8 - Human carbonic anhydrases: tissue distribution, physiological role, and druggability. In: Supuran CT and Nocentini A, editors. Carbonic anhydrases: biochemistry and pharmacology of an evergreen pharmaceutical target. Cambridge (MA): Academic Press; 2019. p. 151–185.
- Ruusuvuori E, Kaila K. Carbonic anhydrases and brain pH in the control of neuronal excitability. Subcell Biochem. 2014;75:271–290.
- Lee SH, McIntyre D, Honess D, Hulikova A, Pacheco-Torres J, Cerdán S, Swietach P, Harris AL, Griffiths JR. Carbonic anhydrase IX is a pH-stat that sets an acidic tumour extracellular pH in vivo. Br J Cancer. 2018;119(5):622–630.
- Supuran CT. Carbonic anhydrases and metabolism. Metabolites. 2018;8(2):25.
- Nocentini A, Supuran CT, Capasso C. An overview on the recently discovered iota-carbonic anhydrases. J Enzyme Inhib Med Chem. 2021;36(1):1988–1995.
- Capasso C. Chapter 5 - δ-Carbonic anhydrases. In: Supuran CT and Nocentini A editors. Carbonic anhydrases: biochemistry and pharmacology of an evergreen pharmaceutical target. Cambridge (MA): Academic Press; 2019. p. 107–129.
- Capasso C, Supuran CT. An overview of the alpha-, beta- and gamma-carbonic anhydrases from bacteria: can bacterial carbonic anhydrases shed new light on evolution of bacteria? J Enzyme Inhib Med Chem. 2015;30(2):325–332.
- Nocentini A, Supuran CT. Advances in the structural annotation of human carbonic anhydrases and impact on future drug discovery. Expert Opin Drug Discov. 2019;14(11):1175–1197.
- Ozensoy Guler O, Capasso C, Supuran CT. A magnificent enzyme superfamily: carbonic anhydrases, their purification and characterization. J Enzyme Inhib Med Chem. 2016;31(5):689–694.
- D’Ambrosio K, De Simone G, Supuran CT. Chapter 2 - Human carbonic anhydrases: catalytic properties, structural features, and tissue distribution. In: Supuran CT, De Simone G, editors. Carbonic anhydrases as biocatalysts. Amsterdam: Elsevier; 2015.
- Stams T, Nair SK, Okuyama T, Waheed A, Sly WS, Christianson DW. Crystal structure of the secretory form of membrane-associated human carbonic anhydrase iv at 2.8-Å resolution. Proc Natl Acad Sci U S A. 1996;93(24):13589–13594.
- Waheed A, Sly WS. Membrane associated carbonic anhydrase IV (CA IV): a personal and historical perspective. Subcell Biochem. 2014;75:157–179.
- Aspatwar A, Supuran CT, Waheed A, Sly WS, Parkkila S. Mitochondrial carbonic anhydrase va and vb: properties and roles in health and disease. J Physiol. 2023;601(2):257–274.
- Kivelä J, Parkkila S, Parkkila A-K, Leinonen J, Rajaniemi H. Salivary carbonic anhydrase isoenzyme VI. J Physiol. 1999;520(2):315–320.
- Ogawa Y, Matsumoto K, Maeda T, Tamai R, Suzuki T, Sasano H, Fernley RT. Characterization of lacrimal gland carbonic anhydrase VI. J Histochem Cytochem. 2002;50(6):821–827.
- Supuran CT, Alterio V, Di Fiore AD, Ambrosio K, Carta F, Monti SM, De Simone G. Inhibition of carbonic anhydrase ix targets primary tumors, metastases, and cancer stem cells: three for the price of one. Med Res Rev. 2018;38(6):1799–1836.
- Supuran CT. Carbonic anhydrase inhibitors as emerging agents for the treatment and imaging of hypoxic tumors. Expert Opin Investig Drugs. 2018;27(12):963–970.
- Ciccone L, Cerri C, Nencetti S, Orlandini E. Carbonic anhydrase inhibitors and epilepsy: state of the art and future perspectives. Molecules. 2021;26(21):6380.
- Scozzafava A, Supuran CT, Carta F. Antiobesity carbonic anhydrase inhibitors: a literature and patent review. Expert Opin Ther Pat. 2013;23(6):725–735.
- Nocentini A, Supuran CT. Carbonic anhydrase inhibitors as antitumor/antimetastatic agents: a patent review (2008–2018). Expert Opin Ther Pat. 2018;28(10):729–740.
- Boztaş M, Çetinkaya Y, Topal M, Gülçin İ, Menzek A, Şahin E, Tanc M, Supuran CT. Synthesis and carbonic anhydrase isoenzymes I, II, IX, and XII inhibitory effects of dimethoxybromophenol derivatives incorporating cyclopropane moieties. J Med Chem. 2015;58(2):640–650.
- Halmi P, Parkkila S, Honkaniemi J. Expression of carbonic anhydrases ii, iv, vii, viii and xii in rat brain after kainic acid induced status epilepticus. Neurochem Int. 2006;48(1):24–30.
- Parkkila S, Parkkila AK, Rajaniemi H, Shah GN, Grubb JH, Waheed A, Sly WS. Expression of membrane-associated carbonic anhydrase XIV on neurons and axons in mouse and human brain. Proc Natl Acad Sci U S A. 2001;98(4):1918–1923.
- McIntyre A, Patiar S, Wigfield S, Li J-L, Ledaki I, Turley H, Leek R, Snell C, Gatter K, Sly WS, et al. Carbonic anhydrase ix promotes tumor growth and necrosis in vivo and inhibition enhances anti-VEGF therapy. Clin Cancer Res. 2012;18(11):3100–3111.
- Waheed A, Sly WS. Carbonic anhydrase xii functions in health and disease. Gene. 2017;623(:33–40.
- Nocentini A, Lucidi A, Perut F, Massa A, Tomaselli D, Gratteri P, Baldini N, Rotili D, Mai A, Supuran CT. Α,γ-diketocarboxylic acids and their esters act as carbonic anhydrase IX and XII selective inhibitors. ACS Med Chem Lett. 2019;10(4):661–665.
- Ghandour MS, Parkkila AK, Parkkila S, Waheed A, Sly WS. Mitochondrial carbonic anhydrase in the nervous system: expression in neuronal and glial cells. J Neurochem. 2000;75(5):2212–2220.
- Shah GN, Hewett-Emmett D, Grubb JH, Migas MC, Fleming RE, Waheed A, Sly WS. Mitochondrial carbonic anhydrase ca vb: differences in tissue distribution and pattern of evolution from those of ca va suggest distinct physiological roles. Proc Natl Acad Sci U S A. 2000;97(4):1677–1682.
- Fujikawa-Adachi K, Nishimori I, Taguchi T, Onishi S. Human mitochondrial carbonic anhydrase VB: cDNA cloning, mRNA expression, subcellular localization, and mapping to chromosome X. J Biol Chem. 1999;274(30):21228–21233.
- Briganti F, Mangani S, Orioli P, Scozzafava A, Vernaglione G, Supuran CT. Carbonic anhydrase activators: X-ray crystallographic and spectroscopic investigations for the interaction of isozymes I and II with histamine. Biochemistry. 1997;36(34):10384–10392.
- Supuran CT. Carbonic anhydrase activators. Future Med Chem. 2018;10(5):561–573.
- Temperini C, Scozzafava A, Vullo D, Supuran CT. Carbonic anhydrase activators. Activation of isozymes I, II, IV, VA, VII, and XIV with l- and d-histidine and crystallographic analysis of their adducts with isoform ii: engineering proton-transfer processes within the active site of an enzyme. Chemistry. 2006;12(27):7057–7066.
- Temperini C, Scozzafava A, Vullo D, Supuran CT. Carbonic anhydrase activators, activation of isoforms i, II, IV, VA, VII, and XIV with L- and D-phenylalanine and crystallographic analysis of their adducts with isozyme ii: stereospecific recognition within the active site of an enzyme and its consequences for the drug design. J Med Chem. 2006;49(10):3019–3027.
- Temperini C, Innocenti A, Scozzafava A, Supuran CT. Carbonic anhydrase activators: kinetic and X-ray crystallographic study for the interaction of D- and L-tryptophan with the mammalian isoforms I-XIV. Bioorg Med Chem. 2008;16(18):8373–8378.
- Temperini C, Innocenti A, Scozzafava A, Mastrolorenzo A, Supuran CT. Carbonic anhydrase activators: L-adrenaline plugs the active site entrance of isozyme II, activating better isoforms I, IV, VA, VII, and XIV. Bioorg Med Chem Lett. 2007;17(3):628–635.
- Dave K, Ilies MA, Scozzafava A, Temperini C, Vullo D, Supuran CT. An inhibitor-like binding mode of a carbonic anhydrase activator within the active site of isoform II. Bioorg Med Chem Lett. 2011;21(9):2764–2768.
- Sun MK, Alkon DL. Pharmacological enhancement of synaptic efficacy, spatial learning and memory through carbonic anhydrase activation in rats. J Pharmacol Exp Ther. 2001;23:83–89.
- Meier-Ruge W, Iwangoff P, Reichlmeier K. Neurochemical enzyme changes in Alzheimer’s and Pick’s disease. Arch Gerontol Geriatr. 1984;3(2):161–165.
- Canto de Souza L, Provensi G, Vullo D, Carta F, Scozzafava A, Costa A, Schmidt SD, Passani MB, Supuran CT, Blandina P. Carbonic anhydrase activation enhances object recognition memory in mice through phosphorylation of the extracellular signal-regulated kinase in the cortex and the hippocampus. Neuropharmacology. 2017;118:148–156.
- Giovannini MG, Efoudebe M, Passani MB, Baldi E, Bucherelli C, Giachi F, Corradetti R, Blandina P. Improvement in fear memory by histamine-elicited ERK2 activation in hippocampal CA3 cells. J Neurosci. 2003;23(27):9016–9023.
- Schmidt SD, Costa A, Rani B, Nachtigall EG, Passani MB, Carta F, Nocentini A, De Carvalho Myskiw J, Furini CRG, Supuran CT, et al. The role of carbonic anhydrases in extinction of contextual fear memory. Proc Natl Acad Sci U S A. 2020;117(27):16000–16008.
- Sun MK, Alkon DL. Carbonic anhydrase gating of attention: memory therapy and enhancement. Trends Pharmacol Sci. 2002;23(2):83–89.
- Provensi G, Nocentini A, Passani MB, Blandina P, Supuran CT. Activation of carbonic anhydrase isoforms involved in modulation of emotional memory and cognitive disorders with histamine agonists, antagonists and derivatives. J Enzyme Inhib Med Chem. 2021;36(1):719–726.
- Angeli A, Vaiano F, Mari F, Bertol E, Supuran CT. Psychoactive substances belonging to the amphetamine class potently activate brain carbonic anhydrase isoforms VA, VB, VII, and XII. J Enzyme Inhib Med Chem. 2017;32(1):1253–1259.
- Casini A, Caccia S, Scozzafava A, Supuran CT. Carbonic anhydrase activators. The selective serotonin reuptake inhibitors fluoxetine, sertraline and citalopram are strong activators of isozymes I and II. Bioorg Med Chem Lett. 2003;13(16):2765–2768.
- Abbate F, Coetzee A, Casini A, Ciattini S, Scozzafava A, Supuran CT. Carbonic anhydrase inhibitors: X-ray crystallographic structure of the adduct of human isozyme II with the antipsychotic drug sulpiride. Bioorg Med Chem Lett. 2004;14(2):337–341.
- Erzengin M, Bilen C, Ergun A, Gencer N. Antipsychotic agents screened as human carbonic anhydrase I and ii inhibitors. Arch Physiol Biochem. 2014;120(1):29–33.
- Simons FER. Advances in H1-antihistamines. N Engl J Med. 2004;351(21):2203–2217.
- Spitzmaul G, Gumilar F, Dilger JP, Bouzat C. The local anaesthetics proadifen and adiphenine inhibit nicotinic receptors by different molecular mechanisms. Br J Pharmacol. 2009;157(5):804–817.
- Ohlow MJ, Moosmann B. Phenothiazine: the seven lives of pharmacology’s first lead structure. Drug Discov Today. 2011;16(3-4):119–131.
- Goldenberg H, Fishman V, Heaton A, Burnett R. A detailed evaluation of promazine metabolism. Proc Soc Exp Biol Med. 1964;115:1044–1051.
- Walkenstein SS, Seifter J. Fate, distribution and excretion of S35 promazine. J Pharmacol Exp Ther. 1959;125(4):283–286.
- Blackburn T, Wasley J. 6.03 - Affective disorders: depression and bipolar disorders. In: Taylor JB and Triggle DJ editors. Comprehensive Medicinal Chemistry II. Oxford: Elsevier; 2007.
- Khalifah RG. The carbon dioxide hydration activity of carbonic anhydrase I. Stop-flow kinetic studies on the native human isoenzymes B and C. J Biol Chem. 1971;246(8):2561–2573.
- Vullo D, Nishimori I, Scozzafava A, Supuran CT. Carbonic anhydrase activators: activation of the human cytosolic isozyme iii and membrane-associated isoform IV with amino acids and amines. Bioorg Med Chem Lett. 2008;18(15):4303–4307.
- Vullo D, Nishimori I, Innocenti A, Scozzafava A, Supuran CT. Carbonic anhydrase activators: an activation study of the human mitochondrial isoforms VA and VB with amino acids and amines. Bioorg Med Chem Lett. 2007;17(5):1336–1340.
- Parkkila S, Vullo D, Puccetti L, Parkkila AK, Scozzafava A, Supuran CT. Carbonic anhydrase activators: activation of isozyme XIII with amino acids and amines. Bioorg Med Chem Lett. 2006;16(15):3955–3959.
- Nishimori I, Onishi S, Vullo D, Innocenti A, Scozzafava A, Supuran CT. Carbonic anhydrase activators: the first activation study of the human secretory isoform VI with amino acids and amines. Bioorg Med Chem. 2007;15(15):5351–5357.
- Pastorekova S, Vullo D, Nishimori I, Scozzafava A, Pastorek J, Supuran CT. Carbonic anhydrase activators: activation of the human tumor-associated isozymes IX and XII with amino acids and amines. Bioorg Med Chem. 2008;16(7):3530–3536.
- Blandina P, Provensi G, Passsani MB, Capasso C, Supuran CT. Carbonic anhydrase modulation of emotional memory. Implications for the treatment of cognitive disorders. J Enzyme Inhib Med Chem. 2020;35(1):1206–1214.
- Abbott NJ. Inflammatory mediators and modulation of blood-brain barrier permeability. Cell Mol Neurobiol. 2000;20(2):131–147.
- Sadiq MW, Borgs A, Okura T, Shimomura K, Kato S, Deguchi Y, Jansson B, Bjorkman S, Terasaki T, Hammarlund-Udenaes M. Diphenhydramine active uptake at the blood-brain barrier and its interaction with oxycodone in vitro and in vivo. J Pharm Sci. 2011;100(9):3912–3923.
- Simons FE, Simons KJ. H1 antihistamines: current status and future directions. World Allergy Organ J. 2008;1(9):145–155.
- Michelot J, Moreau MF, Veyre A, Labarre P, Meyniel G. Brain uptake of labelled adiphenine in rats. Biopharm Drug Dispos. 1986;7(2):197–206.
- Michelot J, Moreau MF, Veyre A, Labarre P, Meyniel G. Adiphenine plasma levels and blood-brain barrier crossing in the rat. Eur J Drug Metab Pharmacokinet. 1985;10(4):273–278.
- Cassini A, Högberg LD, Plachouras D, Quattrocchi A, Hoxha A, Simonsen GS, Colomb-Cotinat M, Kretzschmar ME, Devleesschauwer B, Cecchini M, Burden of AMR Collaborative Group, et al. Attributable deaths and disability-adjusted life-years caused by infections with antibiotic-resistant bacteria in the eu and the european economic area in 2015: a population-level modelling analysis. Lancet Infect Dis. 2019;19(1):56–66.
- Cantisani C, Ricci S, Grieco T, Paolino G, Faina V, Silvestri E, Calvieri S. Topical promethazine side effects: our experience and review of the literature. Biomed Res Int. 2013;2013:151509.
- Daniel W, Syrek M, Janczar L, Boksa J. The pharmacokinetics of promazine and its metabolites after acute and chronic administration to rats- A comparison with the pharmacokinetics of imipramine. Pol J Pharmacol. 1995;47(2):127–136.
- Mahju MA, Maickel RP. Accumulation of phenothiazine tranquilizers in rat brain and plasma after repeated dosage. Biochem Pharmacol. 1969;18(12):2701–2710.
- Alfredsson G, Wiesel F-A, Skett P. Levels of chlorpromazine and its active metabolites in rat brain and the relationship to central monoamine metabolism and prolactin secretion. Psychopharmacology (Berl)). 1977;53(1):13–18.
- Collard JF, Maggs R. Clinical trial of acepromazine maleate in chronic schizophrenia. Br Med J. 1958;1(5085):1452–1454.
- Papich MG. Propiopromazine hydrochloride. In: Papich MG editor. Saunders handbook of veterinary drugs 4th ed. St. Louis: W.B. Saunders; 2016.
- Sallee FR, Pollock BG. Clinical pharmacokinetics of imipramine and desipramine. Clin Pharmacokinet. 1990;18(5):346–364.
- Hrdina PD, Dubas TC. Brain distribution and kinetics of desipramine in the rat. Can J Physiol Pharmacol. 1981;59(2):163–167.
- Glotzbach RK, Preskorn SH. Brain concentrations of tricyclic antidepressants: single-dose kinetics and relationship to plasma concentrations in chronically dosed rats. Psychopharmacology (Berl)). 1982;78(1):25–27.
- Uhr M, Grauer MT, Yassouridis A, Ebinger M. Blood–brain barrier penetration and pharmacokinetics of amitriptyline and its metabolites in P-glycoprotein (ABCB1AB) knock-out mice and controls. J Psychiatr Res. 2007;41(1-2):179–188.
- Nielsen M, Eplov L, Scheel-Krüger J. Protriptyline induced inhibition of the in vivo 3H-noradrenaline synthesis from 3H-L-dopa in the rat brain. Naunyn Schmiedebergs Arch Pharmacol. 1974;285(1):15–28.
- Uhr M, Grauer MT, Holsboer F. Differential enhancement of antidepressant penetration into the brain in mice with ABCB1AB (MDR1AB) P-glycoprotein gene disruption. Biol Psychiatry. 2003;54(8):840–846.