Abstract
In this study, fourteen celastrol derivatives (1–14) were synthesised by esterification of celastrol at the 29th position. The in vitro anticancer activity of them was determined by the MTT assay. All the synthetic compounds showed significant antiproliferative activity against six cancer cells, with IC50 of the submicron molar level. The most promising compound (2) blocked the cell cycle in the G2 phase and inhibited the expression of VEGF and MMP-9 in gastric cancer cell line MGC-803. In flow cytometry analysis, compound 2 induced cancer cell apoptosis in a dose-dependent manner. In the mouse tumour xenograft model, compound 2 showed significant anti-tumour activity in vivo at the dosage of 2.5 mg/kg and 1 mg/kg, with a higher inhibition rate than 5-FU (10 mg/kg). What’s more, the anticancer mechanism involved in the inhibition of VEGF and the toxicity evaluation of compound 2 were also investigated.
Introduction
Recently, the global incidence and mortality of tumours are growing rapidly. The IARC of the WHO released the estimates of the global cancer burden data in 2020, that there were 19 292 789 new cancer cases and 9 958 133 cancer deaths globally in 2020.Citation1 Among the new cancer cases, there are 2.26 million cases of breast cancer in the world, becoming the largest cancer in the world. Malignant tumours seriously endanger people’s health and affect their normal lives. To this day, chemotherapy is still an extensive and effective method for tumour therapy.Citation2,Citation3 However, due to their inability to distinguish between normal and cancerous cells, serious toxic reactions are often caused.Citation4,Citation5 The severe adverse effects, low efficiency caused by drug resistance, and tumour persistency limited the clinical application of the available anticancer agents. The discovery of new anticancer drugs with higher potency and lower toxicity is needed urgently.
Natural plants have built the perfect defense system in the long evolutionary process, in which many toxic natural products were found and utilised to develop anticancer agents.Citation6 Natural products usually have the advantages of broad-spectrum effects, multiple targets, and chemical novelty, and the disadvantages of high toxicity, low solubility, and low activity.Citation7–9 Therefore, the structural modification of natural products to find potent and highly selective anticancer drugs is promising.
Celastrol comes from Tripterygium wilfordii Hook, traditional Chinese medicine of Celastraceae. It is a red needle-like crystal and belongs to quinone methyl pentacyclic triterpene structurely.Citation10 Celastrol has been reported anti-inflammatory,Citation11 antiviral,Citation12 immunomodulatory,Citation13 anticancer,Citation14,Citation15 and other pharmacological activities, of which the anticancer effect was particularly significant and elicited global concern. Although celastrol has broad-spectrum anticancer effects, it also accompanied drawbacks such as poor water solubility, and high toxicity.Citation16,Citation17 And its anticancer activity still needs to be improved. Up to now, there is no report on the marketing of celastrol as a monomer drug. Therefore, the structural modification of celastrol to improve its solubility and anticancer activity, and reduce its toxicity has become a research focus in the field of anticancer research.
Since Yang et al.Citation18 first reported in 2006 that celastrol can induce apoptosis of prostate cancer cells, which triggered the study on the anticancer mechanism of celastrol. Anti-angiogenesis,Citation19,Citation20 HIF-1α expression inhibition,Citation19 and anti-metastasisCitation21,Citation22 have been demonstrated to mediate the anticancer activity of celastrol. A large number of reports have suggested that the 29th carboxylic acid position of celastrol was easily modified,Citation23–26 and the derivatives displayed significant anticancer activity.
Based on the above information, fourteen celastrol derivatives modified on the 29th position of celastrol were synthesised in this study. In these derivatives, compounds 1–7 were designed to hold fatty alcohols of different lengths to improve the hydrophilicity of the molecule. Compounds 8–11 were designed to verify the importance of the terminal hydroxy for the anticancer activity of these derivatives. With the successful development of some indole derivatives as anticancer drugs, more and more research has focused on the design and synthesis of indole derivatives for anticancer activities.Citation27,Citation28 Several studies reported the combination of natural products with indole or azaindole, which led to an improvement in the antitumor activity. Therefore, compounds 12–14 linked by an indole or phthalimide were designed with the expectation of increasing the antitumor potency of celastrol. All the prepared compounds were structurally confirmed by NMR and MS. Their purities were determined by HPLC. Their in vitro anticancer activities were evaluated against six cancer cell lines (AGS, HCT-116, MGC-803, A549, BEL-7402, and HepG-2). What’s more, the in vivo antitumor activities, mechanism of action, as well toxicity of the representative compound 2 were also investigated.Citation29–31
Results and discussion
Chemistry
The esterification occurred at the 29th position of celastrol was used to provide the target compounds. As shown in the Scheme 1, compounds 1–14 were obtained by treating celastrol with different bromoalkanes in the presence of K2CO3 in DMF at room temperature. Before biological evaluation, all target compounds were characterised by 1H-NMR, 13C-NMR, and HR-MS. And the purity of the compound was determined by HPLC. The original spectra of these compounds were provided in the supplementary materials.
Water solubility is a key physicochemical property for drug candidates, especially for the natural products in tetracyclic triterpenoids.Citation32 In this study, one of our important aims is to improve the water solubility of celastrol. To verify the design rationality, the water solubility of compound 2 and celastrol was determined using HPLC. As listed in , the solubility of celastrol in water is 6.2 µg/mL. The water solubility of compound 2 is 11.8 µg/mL, which proved that the introduction of chain alcohol in the 29th position of celastrol could improve the water solubility of celastrol.
Table 1. The solubility of celastrol and compound 2.
In vitro anticancer activity
The vitro anticancer activities of target compounds (1–14) were evaluated against six human cancer cell lines (BEL-7402, AGS, HCT-116, HepG-2, MGC-803, and A549). The activity of celastrol was determined simultaneously as the positive control. As shown in , All of the target compounds showed significant antiproliferative effects with IC50 at the submicro-to-micro molar level. Among them, compounds 1, 2, 3, 4, 6, 9, and 13 showed lower IC50 values (0.58–0.77 µM) than celastrol (0.79 µM) in hepatocellular carcinoma cells (Bel7402). Compounds 2, 3, 5, 13, and 14 showed lower IC50 values (0.32–0.37 µM) than celastrol (0.39 µM) in human gastric cancer cells (AGS). Compounds 2 and 3 showed lower IC50 values (0.32–0.39 µM) than celastrol (0.43 µM) in human colorectal cancer cells (HCT116). Compounds 1, 2, 3, and 10 showed lower IC50 values (0.62–0.94 µM) than celastrol (1.23 µM) in human liver cancer cells (HepG-2). Compounds 2, 3, 4, and 13 showed lower IC50 values (0.27–0.34 µM) than celastrol (0.35 µM) in human gastric cancer cells (MGC803). Compounds 1, 2, 3, 7, 9, 10, and 14 showed lower IC50 values (3.06–4.30 µM) than celastrol (5.34 µM) in human lung cancer cells (A549). Overall, compounds 2 and 3 showed more potent antiproliferative activities in vitro than celastrol against all the test cancer cell lines.
Table 2. Antiproliferative efficacy of celastrol derivatives 1–14 in six human cancer cell lines.
Based on these in vitro antiproliferative results, significant patterns of structure-activity relationships were not established. But some trends are still visible. Compounds 1–7 were substituted by fatty alcohols of different carbon chain lengths. The carbon chain length seems to affect the antiproliferative effect with two or three carbon as the optimum. When the hydroxyl group of compound 2 was replaced by bromine, it provided compound 8, which exhibited a weaker antiproliferative effect than celastrol. When the hydroxyl group of compounds 2 or 3 was replaced by a hydrophobic ester, methoxy, or phenoxy group, it provided the compounds 9, 10, and 11, respectively. The antiproliferative effects were also reduced when compared to compounds 2 or 3. The hydrophobicity and steric size were the possible influencing factors when defining compound 11 as the weakest one among them. Compounds 12–14 were designed to obtain derivatives with better anticancer activity due to the indole always improving the activity of the natural product. However, it doesn’t work in this study. Compounds 12, 13, and 14 exhibited activities similar to that of celastrol.
Selectivity indexes between cancer cells and LO2 cells
Non-selective cytotoxicity is an important reason to limit the clinical application of celastrol. To investigate the cytotoxicity against a normal cell of the synthesised celastrol derivatives, and obtained their selectivity indexes between cancer cells and normal cells, the growth inhibition of compounds 1–14 and celastrol against human normal liver cells (LO2) were determined. Selectivity indexes between cancer cells and LO2 cells were calculated from their IC50 values. As shown in , all compounds showed significant cytotoxicity in the LO2 cell. Unfortunately, all compounds didn’t exhibit selectivity between cancer cells and human normal liver cell (LO2), with the selectivity indexes around one.
Table 3. In vitro antiproliferative activities of celastrol derivatives 1–14 against normal cell line (LO2) and selectivity index.
Compound 2 induced cancer cell cycle arrest
Cell cycle dysregulation and uncontrolled mitosis are important causes of the infinite proliferation of cancer cells.Citation33 Therefore, blocking the cell cycle and inhibiting the mitotic division of cells are considered to be important reasons for inhibiting cell proliferation. To explore the antiproliferative mechanism of compound 2, the effects of compound 2 on the cell cycle progression were explored by flow cytometry in the MGC-803 cell. The experimental results were shown in . After treating cells with compound 2 for 18 h, the number of cells in the G2 phase was increased in a concentration-dependent manner (from 15.05% to 37.01%), The number of cells in the G1 phase was decreased (from 64.36% to 44.77%). The proportion of cells in the S phase was almost unchanged. While celastrol reduced the proportion of G1 phase cells (from 63.37% to 52.96%) and increased the proportion of S phase cells (from 19.18% to 29.41%). The proportion of G2 phase cells remained almost unchanged. The above results showed that compound 2 can block the cell cycle in the G2 phase, while celastrol blocks the cell cycle in the S phase.
Compound 2 induced cancer cell apoptosis
The ability of compound 2 to induce cancer cell apoptosis was also investigated by the flow cytometry analysis. MGC-803 cells were treated with vehicle (0.1% DMSO), celastol (0.1 µM, 0.5 µM, 1 µM), and compound 2 (0.1 µM, 0.5 µM, 1 µM), respectively for 18 h. And then cells were collected and stained with Annexin V-FITC and PI. The cell apoptosis situations were shown in . Compared with the blank control, the overall apoptosis rate (early apoptosis + late apoptosis) of cells treated with compound 2 and celastrol both increased in a dose-dependent manner, though there was no significant difference (the overall apoptosis rate from 8.2 to 13% and 9.8%, respectively). This result indicates that apoptosis only played a weak role for compound 2 and celastrol in inhibiting the proliferation of MGC-803.
Compound 2 inhibited the expression of VEGF
Tumour cells can secrete more growth factors such as vascular endothelial growth factor (VEGF) when they grow and proliferate, which can promote the formation and metastasis of tumour blood vessels. Therefore, many anti-tumour drugs resist cancer by inhibiting the production or activity of VEGF and then inhibiting tumour angiogenesis.Citation34 It has been reported that celastrol inhibited the secretion of VEGF in endothelial cells and exhibited an antiangiogenesis effect.Citation20 Therefore, in order to verify whether the anti-tumour effect of the synthesised celastrol derivatives is related to its inhibition of VEGF, the effects of compound 2 and celastrol on the expression of VEGF in gastric cancer cell MGC-803 were determined using ELISA.
As shown in , compound 2 and celastrol inhibited the expression of VEGF in a concentration-dependent manner regardless of whether the cell survival environment is hypoxia or not. Under the condition of CoCl2-induced hypoxia, compound 2 treated at 0.1 µM, 0.5 µM, and 1 µM downregulated the VEGF expression significantly from 568.1 pg/ml to 430.8 pg/ml, 397.5 pg/ml, and 328.8 pg/ml, respectively. Under normal oxygen conditions, compound 2 treated at 0.1 µM, 0.5 µM, and 1 µM downregulated the VEGF expression from 531.5 pg/ml to 514.1 pg/ml, 398.1 pg/ml, and 352.1 pg/ml, respectively. These results suggested the inhibition of the VEGF played an important role in the antiproliferative activity of compound 2 and celastrol against gastric cancer cell line MGC-803.
Figure 3. Compound 2 and celastrol inhibited the expression quantity of VEGF in MGC-803 cells. (A) The effects of compound 2, and Celastrol (0.1 µM, 0.5 µM, 1 µM) on the secretion of VEGF in Hypoxia-induced MGC-803. (B) The effects of compound 2, and Celastrol (0.1 µM, 0.5 µM, 1 µM) on the secretion of VEGF in MGC-803. Data are presented as ng.ml−1 and were analysed using one-way ANOVA with Graphpad Prism 7. **p < 0.01, ***p < 0.001 when compared to control group (1%DMSO) (n ≥ 3).
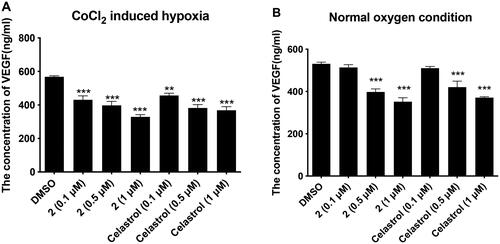
Compound 2 inhibited the expression of MMP-9
MMP-9 is a major proteolytic enzyme involved in the degradation of the basement membrane, which is also involved in the destruction of the histological barrier of tumour cell invasion and plays a key role in tumour invasion and metastasis.Citation35 In addition, MMP-9 participates in tumour angiogenesis by regulating the release of VEGF. In order to further understand the anti-tumour mechanism of the celastrol derivative 2 and its ability to inhibit the invasion and metastasis of tumour cells, the effects of compound 2 and celastrol on the expression of MMP-9 in gastric cancer cell MGC-803 were determined using ELISA.
As shown in , compound 2 and celastrol inhibited the expression of MMP-9 in MGC-803 cells in a concentration-dependent manner. When the cells were treated with compound 2 at 0.5 µM and 1 µM, the MMP-9 expression was downregulated significantly from 18.6 ng/ml to 10.04 ng/ml and 9.64 ng/ml, respectively. At the same concentration, compound 2 showed higher inhibition than that of celastol. These results further explained the antiproliferative mechanism of compound 2 via inhibiting the VEGF pathway and also indicated that compound 2 has the potential to inhibit tumour invasion and metastasis.
Figure 4. The effects of compound 2 (0.1 µM, 0.5 µM, 1 µM) and celastrol (0.1 µM, 0.5 µM, 1 µM) on the secretion of of MMP-9 in MGC-803. Data are presented as ng ml−1 and were analysed using one-way ANOVA with Graphpad Prism 7. **p < 0.01, ***p < 0.001 when compared to control group (0.1%DMSO) (n ≥ 3).
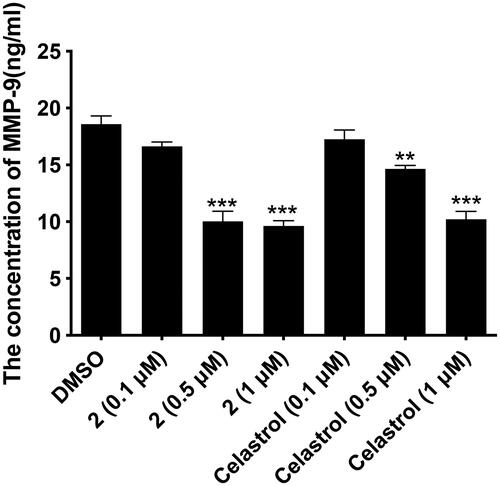
In vivo anticancer effect of compound 2 in nude mice
Compound 2, which exhibited excellent antiproliferative activity against cancer cells in vitro, was subjected to in vivo antitumor activity evaluation. The nude mice were inoculated with HCT116 cancer cells to establish a tumour model in the mice. The tumour mice were randomly divided into four groups: model control group, positive drug group (5-fluorouracil (5-FU), 10 mg/kg), high-dose compound 2 group (2.5 mg/kg), and low-dose compound 2 group (1 mg/kg). After treating for 20 days, the change in tumour volume of mice in each group was recorded and shown in . It can be seen that the tumour volume of mice in the control group increased with time pass. The tumour volume of mice in the compound 2 and 5-FU groups also showed an increasing trend. But the growth rate was significantly slower than the model group. At the end of the administration, the tumour volume of mice treated with compound 2 (2.5 mg/kg, 1 mg/kg) and 5-FU were both significantly smaller than that of the control (p < 0.01, and p < 0.01).
Figure 5. The effects of compound 2 (1 mg/kg, 2.5 mg/kg) and 5-FU (10 mg/kg) on the tumour volume of mice. *p < 0.05, and **p < 0.01 compared with Control group.
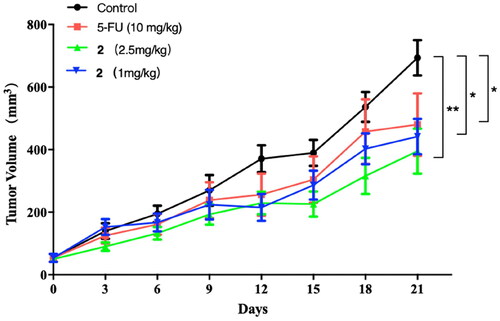
At the end of the administration, the mice were sacrificed and the tumour was weighed. As shown in , the tumour weight of mice treated with compound 2 (2.5 mg/kg, 1 mg/kg) and 5-FU was significantly lower than that in the control group (p < 0.001). The tumour growth inhibition rates of the 5-FU group, high-dose compound 2 group, and low-dose compound 2 group, were 37.02%, 55.82%, and 50.83%, respectively. These results indicate that compound 2 exhibited higher antitumor abilities than 5-FU even at lower doses. The mice treated with 1 mg/kg of compound 2 showed no significant changes of body weight during the treatment period. But the body weight of the mice treated with 2.5 mg/kg of compound 2 showed a downward trend. And the mental state of the mice also became depressed. This indicates the toxicity of compound 2 ().
ELISA detection of TNF- α
Tumour necrosis factor alpha (TNF-α), a major inflammatory cytokine, is involved in the maintenance and homeostasis of the immune system, inflammation, and host defense. The critical pathogenic role of TNF-α in inflammatory disorders has been well established. Increasing evidence suggests that tumour genesis and growth are also related to TNF-α.Citation36 On the one hand, it can inhibit the growth of tumour cells by inducing apoptosis of cancer cells. But on the other hand, it can also stimulate the proliferation, survival, migration, and angiogenesis of most cancer cells that are resistant to TNF-induced cytotoxicity, leading to the development of tumours. It is both a target and a therapeutic in malignant diseases.Citation37
Figure 6. The effects of compound 2 (1 mg/kg, 2.5 mg/kg) and 5-FU (10 mg/kg) on the tumour weight and body weight of mice. (A) Compound 2 and 5-FU inhibited the growth of tumours in mice. ***p < 0.001, and ****p < 0.0001 compared with the control group, ##p < 0.01 when comparing the 5-FU group and high dose group of compound 2; (B) Body weight changes of mice during administration.
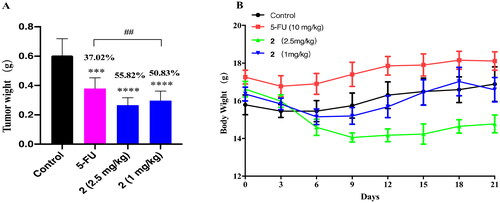
In order to better understand the anti-tumour mechanism of celastrols, the TNF-α content of the above tumour nude mice in the in vivo assay was detected by ELISA. As shown in , 5-FU increased the TNF-α content significantly when compared to the control. Oppositely, compound 2 decreased the TNF-α content significantly in a dose-dependent manner. These results indicated that compound 2 might display anti-tumour action via some mechanism or pathway different from 5-FU, such as inhibiting HIF-1α/VEGF pathway.Citation38
Haematoxylin-eosin staining (HE) analysis
To further explore the antitumor effect of compound 2, HE analysis was carried out to assess the morphological changes in tumour tissue. The results were illustrated in . In the tumorigenic control group, the tissue structure was disordered, the cell shape was irregular, the arrangement was tight, and the blood vessels were rich. Compared with the control group, the tumour tissue structure was seriously damaged and the cell necrosis was obvious in the compound 2 and 5-FU treated groups. The degree of cell necrosis was more serious in the high dose of compound 2. These results suggest that compound 2 has the ability to kill tumour cells and destroy tumour tissue structure, and its influence on cell morphology is stronger than that of 5-FU.
Immunohistochemical detection of VEGF expression
Based on the previous in vitro tests, we found that the inhibition of the VEGF played an important role in the antiproliferative activities of compound 2. To further verify this antitumor mechanism, the expression level of VEGF in the tumour tissue was detected by immunohistochemistry. The effects of compound 2 (1 mg/kg, 2.5 mg/kg) and 5-FU (10 mg/kg) on the VEGF expression in tumour mice were shown in . The VEGF expression coloured by DBA was indicated by the brown-yellow area. The VEGF expression level was quantitatively analysed by Image J. As presented in , compound 2 (1 mg/kg, 2.5 mg/kg) and 5-FU (10 mg/kg) both downregulated the VEGF expression significantly (****p < 0.0001) when compared to the control group. And the regulation from compound 2 was dose-dependent.
Western blot analysis of VEGF expression
Accompanied by the immunohistochemical detection of VEGF expression, the western blot analysis of VEGF expression was also carried out. As shown in , compound 2 (1 mg/kg, 2.5 mg/kg) and 5-FU (10 mg/kg) both downregulated the VEGF expression significantly (*p < 0.05, **p < 0.01) in the western blot analysis. This result further supports the involvement of VEGF in the antitumor activity of compound 2. Celastrols synthesised in this study might inhibit tumour angiogenesis by downregulating the VEGF and then block the tumour growth and metastasis.
Toxicity of compound 2 in the zebrafish model
The embryotoxicity of zebrafish was shown in . The death rate reached 50% when the embryo was exposed to celastrol at a concentration of 0.2 μM, and the embryo was all dead at a concentration of 0.2 μM or higher. At concentrations of 0.1 μM, compound 2 caused no acute toxicity for zebrafish embryos. When the concentration raised to 0.5 μM, the mortality began to increase in a gradient. On the fourth day, the mortality reached 100%. As the concentration of compound 2 increased, the death of the embryo also accelerated. The death rate reached 100% on the first day when it was exposed to 0.5 μM of compound 2. It can be seen that the embryotoxicity of compound 2 was greater than that of celastrol.
The hatching rate of zebrafish was shown in . For celastrol, the incubation rate under exposure concentrations of 0.1, 0.15, and 0.2 μM were more than 75%. The incubation rate was zero when the eggs were exposed to celastrol at 0.3 or 0.5 μM. When exposed to 0.15 μM of compound 2, the incubation rate was 25%. And no eggs hatched when exposed to 0.2 μM of compound 2.
Figure 13. Death rate of adult zebrafish treated with celastrol (abbreviated as C) and compound 2 (abbreviated as T) analysed at 24 and 72 hpf.
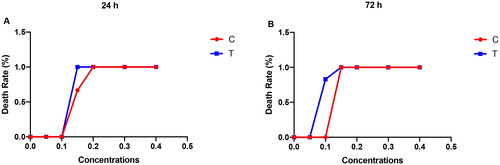
As shown in , celastrol and compound 2 both caused acute toxicity in zebrafish larvae at concentrations higher than 0.1 μM. Mortality of larvae increased with increasing concentration and exposure time. All zebrafish dead when exposed to compound 2 for 24 h at the concentration of 0.15 μM. And the 100% mortality concentration decreased to 0.1 μM when exposed for 72 h. Comparing the toxicity of compound 2 and celastrol, it can be seen that the toxicity of compound 2 is slightly higher than that of celastrol.
Conclusion
In recent years, many reports were focused on the development and research of celastrol and its derivatives as anticancer agents. The main purpose of these studies is to reduce its toxicity, improve its water solubility/potency by modification, and elucidate its anticancer mechanism. Based on the same purpose, fourteen celastrol derivatives were designed, synthesised, and evaluated for their anticancer properties in this study. All the target compounds showed potent inhibitory effects against gastric cancer cells, liver cancer cells, lung cancer cells, and colon cancer cells in vitro at the submicro-to-micro molar level. Compound 2, with the IC50 of 0.33 μM against gastric cancer cells AGS and colon cancer cells HCT-116, was the most promising one in this work. Compound 2 blocked the cell cycle in the G2 phase, and inhibited the expression of VEGF and MMP-9 in the MGC-803 cells. In the in vivo anti-tumour activity assay, compound 2 significantly inhibited tumour growth in tumour mice, with a higher inhibition rate than 5-FU at a lower dose. Immunohistochemical and WB analysis indicated that compound 2 could significantly reduce the level of VEGF in tumour mice, which suggested that the antitumor activity of compound 2 was probably due to the down-regulation of VEGF level, then inhibiting the angiogenesis, and ultimately inhibiting the growth and proliferation of tumour. The water solubility of compound 2 is higher than that of the parent molecule celastrol, which may be the reason for its better anticancer activity. Unfortunately, compound 2 showed greater toxicity than celastrol in the zebrafish model.
To sum up, a series of celastrol derivatives were designed and synthesised. Improved water solubility and anticancer potency (in vitro and in vivo) were achieved, though the toxicity increased with the anticancer potency. The inhibition of VEGF was involved in their anticancer activity. The follow-up research will focus on the research of the anticancer mechanism, such as the regulation of HIF-1 and JAK-STSR pathways. It is also one of our pursuing to obtain more efficient celastrol derivatives by improving the VEGF inhibitory activity via hybridisation.
Experimental section
Chemistry part
Celastrol was provided by Chengdu Biopurify Phytochemicals Ltd. with a purity of 99%. Other chemical reagents were purchased from Macklin reagent. In all reactions, thin-layer chromatography (TLC) was used for monitoring the reaction process. After purification, products were sent to the analysis centre for structure confirmation. 1H NMR and 13C NMR spectrums were measured on a Bruker NMR spectrometer. HR-MS was measured on a Xevo G2-XS QTof mass spectrometer. The purity was measured on an Agilent 1260 HPLC.
General procedure for the preparation of celastrol dericatives (1-14)
A mixture of celastrol (50 mg, 0.1 mmol), K2CO3 (55 mg, 0.4 mmol), and bromoalkene (0.4 mmol) in DMF (5 ml) was stirred at 25oC for 2–12h. The accurate reaction time depended on the reaction progress monitored by thin-layer chromatography. After the reaction finished, 20 ml of water was added to the mixture, and it was put into the refrigerator for 30 min. Then it was extracted by ethyl acetate for 3 times. The combined organic layer was washed with saturated salt water for 3 times, dried with anhydrous MgSO4, filtered, and evaporated in a vacuum. The resulting residue was purified using silica gel column chromatography (Hexane/EtOAc, 90:10–50:50) to provide the target compound 1–14.
Spectral data and physicochemical properties of celastrol dericatives (1–14)
2-Hydroxyethyl (2 R,4aS,6aS,12bR,14aS,14bR)-10-hydroxy-2,4a,6a,9,12b,14a-hexamethyl-11-oxo-1,2,3,4,4a,5,6,6a,11,12b,13,14,14a,14b-tetradecahydropicene-2-carboxylate (1) Red solid; yield 46%; m.p. 82 °C–84 °C. 1H-NMR (CDCl3, 300 MHz): δ 7.04 (d, J = 7.1 Hz, 1H), 7.01 (s, 1H), 6.54 (s, 1H), 6.36 (d, J = 7.2 Hz, 1H), 4.00–4.16 (m, 2H), 3.80 (t, J = 4.5 Hz, 2H), 2.48 (d, J = 15.6 Hz, 1H), 2.22 (s, 3H), 1.63-2.16 (m, 14H), 1.47 (s, 3H), 1.28 (s, 3H), 1.23 (s, 3H), 1.12 (s, 3H), 0.59 (s, 3H). 13C NMR (75 MHz, CDCl3) δ 178.7, 178.4, 170.1, 164.8, 146.0, 134.2, 127.4, 119.6, 118.2, 117.2, 66.1, 61.0, 45.0, 44.3, 42.9, 40.5, 39.5, 38.3, 36.4, 34.8, 33.5, 32.8, 31.6, 30.8, 30.6, 29.8, 29.7, 28.6, 21.6, 18.5, 10.3. ESI-HRMS (m/z): calcd for C31H43O5+ ([M + H]+): 495.3105; found: 495.3106. Purity: 96.5134% by HPLC (A: 0.1% Phosphoric acid in water: B: Acetonitrile, grade: B, 75%–90%), tR = 11.807 min, λ = 254 nm.
3-Hydroxypropyl (2 R,4aS,6aS,12bR,14aS,14bR)-10-hydroxy-2,4a,6a,9,12b,14a-hexamethyl-11-oxo-1,2,3,4,4a,5,6,6a,11,12b,13,14,14a,14b-tetradecahydropicene-2-carboxylate (2) Red solid; yield 54%; m.p. 92 °C–94 °C. 1H-NMR (CDCl3, 300 MHz): δ 7.04 (d, J = 7.1 Hz, 1H), 7.01 (s, 1H), 6.55 (s, 1H), 6.36 (d, J = 7.1 Hz, 1H), 4.12–4.20 (m, 1H), 3.99-4.05 (m, 1H), 3.69 (t, J = 6.0 Hz, 2H), 2.44 (d, J = 15.6 Hz, 1H), 2.22 (s, 3H), 1.59–2.11 (m, 16H), 1.46 (s, 3H), 1.28 (s, 3H), 1.20 (s, 3H), 1.11 (s, 3H), 0.57 (s, 3H). 13C NMR (75 MHz, CDCl3) δ 178.6, 178.4, 170.1, 164.8, 146.0, 134.2, 127.4, 119.5, 118.2, 117.3, 61.5, 59.4, 45.1, 44.3, 42.9, 40.5, 39.4, 38.3, 36.4, 34.8, 33.5, 32.8, 31.6, 30.8, 30.5, 29.8, 29.7, 28.6, 21.6, 18.5, 14.1, 10.3. ESI-HRMS (m/z): calcd for C32H45O5+ ([M + H]+): 509.3262; found: 509.3265. Purity: 98.0580% by HPLC (A: 0.1% Phosphoric acid in water: B: Acetonitrile, grade: B, 80-95%), tR = 11.230 min, λ = 254 nm.
4-Hydroxybutyl (2 R,4aS,6aS,12bR,14aS,14bR)-10-hydroxy-2,4a,6a,9,12b,14a-hexamethyl-11-oxo-1,2,3,4,4a,5,6,6a,11,12b,13,14,14a,14b-tetradecahydropicene-2-carboxylate (3) Red solid; yield 57%; m.p. 100 °C–101 °C. 1H-NMR (CDCl3, 500 MHz): δ 7.02 (d, J = 7.2 Hz, 1H), 6.96 (s, 1H), 6.53 (s, 1H), 6.35 (d, J = 7.2 Hz, 1H), 3.97–4.01 (m, 1H), 3.87-3.92 (m, 1H), 3.67 (t, J = 6.2 Hz, 2H), 2.44 (d, J = 15.8 Hz, 1H), 2.21 (s, 3H), 1.59–2.19 (m, 18H), 1.45 (s, 3H), 1.26 (s, 3H), 1.18 (s, 3H), 1.10 (s, 3H), 0.56 (s, 3H). 13C NMR (126 MHz, CDCl3) δ 178.4, 178.3, 170.1, 164.8, 146.0, 134.1, 127.4, 119.5, 118.2, 117.2, 64.3, 62.4, 45.1, 44.3, 43.0, 40.4, 39.4, 38.3, 36.4, 34.8, 33.5, 32.8, 31.6, 30.8, 30.6, 29.7, 29.3, 28.7, 24.9, 21.6, 18.5, 14.1, 10.3. ESI-HRMS (m/z): calcd for C33H47O5+ ([M + H]+): 523.3418; found: 523.3422. Purity: 99.4736% by HPLC (A: 0.1% Phosphoric acid in water: B: Acetonitrile, grade: B, 75-85%), tR = 17.179 min, λ = 254 nm.
5-Hydroxypentyl (2 R,4aS,6aS,12bR,14aS,14bR)-10-hydroxy-2,4a,6a,9,12b,14a-hexamethyl-11-oxo-1,2,3,4,4a,5,6,6a,11,12b,13,14,14a,14b-tetradecahydropicene-2-carboxylate (4) Red solid; yield 44%; m.p. 90 °C–91 °C. 1H-NMR (CDCl3, 300 MHz): δ 7.05 (d, J = 7.1 Hz, 1H), 6.98 (s, 1H), 6.56 (s, 1H), 6.37 (d, J = 7.1 Hz, 1H), 3.89–3.97 (m, 2H), 3.66 (t, J = 6.1 Hz, 2H), 2.46 (d, J = 15.7 Hz, 1H), 2.23 (s, 3H), 1.58–2.11 (m, 20 H), 1.47 (s, 3H), 1.28 (s, 3H), 1.19 (s, 3H), 1.12 (s, 3H), 0.56 (s, 3H). 13C NMR (126 MHz, CDCl3) δ 178.3, 178.2, 170.4, 164.9, 146.1, 134.3, 127.4, 119.5, 118.2, 117.4, 64.5, 62.6, 45.1, 44.3, 42.9, 40.4, 39.4, 38.3, 36.4, 34.7, 33.6, 32.8, 32.3, 31.6, 30.7, 30.6, 29.9, 29.6, 28.7, 28.3, 22.5, 21.6, 18.4, 10.3. ESI-HRMS (m/z): calcd for C34H49O5+ ([M + H]+): 537.3575; found: 537.3576. Purity: 95.3441% by HPLC (A: 0.1% Phosphoric acid in water: B: Acetonitrile, grade: B, 85-95%), tR = 12.026 min, λ = 254 nm.
6-Hydroxyhexyl (2 R,4aS,6aS,12bR,14aS,14bR)-10-hydroxy-2,4a,6a,9,12b,14a-hexamethyl-11-oxo-1,2,3,4,4a,5,6,6a,11,12b,13,14,14a,14b-tetradecahydropicene-2-carboxylate (5) Red solid; yield 49%; m.p. 78 °C–79 °C. 1H-NMR (CDCl3, 400 MHz): δ 7.08 (d, J = 7.0 Hz, 1H), 6.98 (s, 1H), 6.61 (s, 1H), 6.38 (d, J = 7.1 Hz, 1H), 3.87–3.91 (m, 2H), 3.57–3.67 (m, 2H), 2.45 (d, J = 15.8 Hz, 1H), 2.23 (s, 3H), 1.52–2.06 (m, 18 H), 1.46 (s, 3H), 1.33–1.40 (m, 4H), 1.28 (s, 3H), 1.19 (s, 3H), 1.11 (s, 3H), 0.56 (s, 3H). 13C NMR (101 MHz, CDCl3) δ 178.3, 178.3, 171.0, 165.2, 146.1, 134.9, 127.3, 119.3, 118.3, 117.8, 64.8, 62.6, 45.1, 44.3, 43.0, 40.4, 39.4, 38.2, 36.3, 34.7, 33.8, 33.0, 32.7, 31.6, 30.7, 30.5, 29.9, 29.5, 28.6, 28.4, 26.4, 25.6, 21.5, 18.3, 10.3. ESI-HRMS (m/z): calcd for C35H51O5+ ([M + H]+): 551.3731; found: 551.3731. Purity: 96.5510% by HPLC (A: 0.1% Phosphoric acid in water: B: Acetonitrile, grade: B, 80-95%), tR = 15.809 min, λ = 254 nm.
7-Hydroxyheptyl (2 R,4aS,6aS,12bR,14aS,14bR)-10-hydroxy-2,4a,6a,9,12b,14a-hexamethyl-11-oxo-1,2,3,4,4a,5,6,6a,11,12b,13,14,14a,14b-tetradecahydropicene-2-carboxylate (6) Red solid; yield 43%; m.p. 68 °C–70 °C. 1H-NMR (CDCl3, 300 MHz): δ 7.07 (d, J = 7.1 Hz, 1H), 6.97 (s, 1H), 6.57 (s, 1H), 6.38 (d, J = 7.1 Hz, 1H), 3.86–3.95 (m, 2H), 3.67 (t, J = 6.3 Hz, 2H), 2.45 (d, J = 15.7 Hz, 1H), 2.24 (s, 3H), 1.55-2.06 (m, 16 H), 1.47 (s, 3H), 1.39–1.43 (m, 6H), 1.28 (s, 3H), 1.19 (s, 3H), 1.12 (s, 3H), 0.85–0.92 (m, 2 H), 0.57 (s, 3H). 13C NMR (126 MHz, CDCl3) δ 178.3, 178.2, 170.5, 165.1, 146.1, 134.6, 127.4, 119.3, 118.2, 117.5, 64.7, 62.7, 45.1, 44.4, 43.1, 40.4, 39.5, 38.2, 36.4, 34.8, 33.7, 32.9, 32.7, 31.6, 30.7, 30.5, 29.9, 29.7, 29.4, 28.6, 28.5, 26.5, 26.1, 21.6, 18.3, 10.3. ESI-HRMS (m/z): calcd for C36H53O5+ ([M + H]+): 565.3888; found: 565.3889. Purity: 95.8273% by HPLC (A: 0.1% Phosphoric acid in water: B: Acetonitrile, grade: B, 85%–95%), tR = 16.451 min, λ = 254 nm.
8-Hydroxyoctyl (2 R,4aS,6aS,12bR,14aS,14bR)-10-hydroxy-2,4a,6a,9,12b,14a-hexamethyl-11-oxo-1,2,3,4,4a,5,6,6a,11,12b,13,14,14a,14b-tetradecahydropicene-2-carboxylate (7) Red solid; yield 43%; m.p. 66 °C–68 °C. 1H-NMR (CDCl3, 300 MHz): δ 7.07 (d, J = 7.2 Hz, 1H), 7.02 (s, 1H), 6.55 (s, 1H), 6.38 (d, J = 7.2 Hz, 1H), 3.83–3.96 (m, 2H), 3.65 (t, J = 6.2 Hz, 2H), 2.44 (d, J = 15.7 Hz, 1H), 2.23 (s, 3H), 1.57-2.20 (m, 16 H), 1.47 (s, 3H), 1.34-1.38 (m, 8H), 1.28 (s, 3H), 1.19 (s, 3H), 1.11 (s, 3H), 0.84–0.91 (m, 2 H), 0.56 (s, 3H). 13C NMR (101 MHz, CDCl3) δ 178.3, 178.3, 170.5, 165.0, 146.1, 134.6, 127.3, 119.3, 118.2, 117.5, 64.6, 62.7, 45.1, 44.3, 43.1, 40.4, 39.5, 38.3, 36.4, 34.8, 33.6, 32.8, 32.7, 31.6, 30.7, 30.5, 29.9, 29.6, 29.3, 29.2, 28.6, 28.3, 26.1, 25.7, 21.6, 18.4, 10.3. ESI-HRMS (m/z): calcd for C37H55O5+ ([M + H]+): 579.4044; found: 579.4046. Purity: 97.9517% by HPLC (A: 0.1% Phosphoric acid in water: B: Acetonitrile, grade: B, 95%–100%), tR = 14.867 min, λ = 254 nm.
3-Bromopropyl (2 R,4aS,6aS,12bR,14aS,14bR)-10-hydroxy-2,4a,6a,9,12b,14a-hexamethyl-11-oxo-1,2,3,4,4a,5,6,6a,11,12b,13,14,14a,14b-tetradecahydropicene-2-carboxylate (8) Red solid; yield 43%; m.p. 73 °C–75 °C. 1H-NMR (CDCl3, 400 MHz): δ 7.03 (d, J = 7.1 Hz, 1H), 7.02 (s, 1H), 6.55 (s, 1H), 6.36 (d, J = 7.1 Hz, 1H), 4.11-–4.17 (m, 1H), 3.97–4.03 (m, 1H), 3.47 (t, J = 6.4 Hz, 2H), 2.42 (d, J = 15.8 Hz, 1H), 2.22 (s, 3H), 1.53–2.16 (m, 14 H), 1.46 (s, 3H), 1.27 (s, 3H), 1.20 (s, 3H), 1.11 (s, 3H), 0.85-0.91 (m, 2 H), 0.56 (s, 3H). 13C NMR (101 MHz, CDCl3) δ 178.3, 178.1, 169.9, 164.7, 146.0, 134.1, 127.4, 119.6, 118.2, 117.2, 62.0, 45.0, 44.2, 42.9, 40.5, 39.4, 38.3, 36.3, 34.8, 33.5, 32.8, 31.6, 31.4, 30.8, 30.5, 29.8, 29.7, 29.4, 28.6, 21.7, 18.6, 10.3. ESI-HRMS (m/z): calcd for C32H44BrO4+ ([M + H]+): 571.2417; found: 571.2418. Purity: 98.2356% by HPLC (A: 0.1% Phosphoric acid in water: B: Acetonitrile, grade: B, 85%–95%), tR = 15.198 min, λ = 254 nm.
2-Acetoxyethyl (2 R,4aS,6aS,12bR,14aS,14bR)-10-hydroxy-2,4a,6a,9,12b,14a-hexamethyl-11-oxo-1,2,3,4,4a,5,6,6a,11,12b,13,14,14a,14b-tetradecahydropicene-2-carboxylate (9) Red solid; yield 67%; m.p. 78 °C–80 °C. 1H-NMR (CDCl3, 400 MHz): δ 7.03 (d, J = 7.2 Hz, 1H), 7.02 (s, 1H), 6.52 (s, 1H), 6.36 (d, J = 7.2 Hz, 1H), 4.19–4.30 (m, 3H), 4.00–4.07 (m, 1H), 2.43 (d, J = 15.7 Hz, 1H), 2.22 (s, 3H), 2.07 (s, 3H), 1.52–1.94 (m, 12 H), 1.46 (s, 3H), 1.27 (s, 3H), 1.19 (s, 3H), 1.11 (s, 3H), 0.85–0.90 (m, 2 H), 0.56 (s, 3H). 13C NMR (101 MHz, CDCl3) δ 178.3, 178.1, 170.6, 169.9, 164.7, 146.0, 134.1, 127.4, 119.6, 118.2, 117.1, 62.2, 61.9, 45.0, 44.2, 42.9, 40.5, 39.4, 38.3, 36.4, 34.7, 33.6, 32.7, 31.6, 30.8, 30.5, 29.8, 29.7, 28.6, 21.6, 20.8, 18.6, 10.3. ESI-HRMS (m/z): calcd for C33H45O6+ ([M + H]+): 537.3211; found: 537.3210. Purity: 98.4626% by HPLC (A: 0.1% Phosphoric acid in water: B: Acetonitrile, grade: B, 75%–95%), tR = 14.832 min, λ = 254 nm.
3-Methoxypropyl (2 R,4aS,6aS,12bR,14aS,14bR)-10-hydroxy-2,4a,6a,9,12b,14a-hexamethyl-11-oxo-1,2,3,4,4a,5,6,6a,11,12b,13,14,14a,14b-tetradecahydropicene-2-carboxylate (10) Red solid; yield 69%; m.p. 66 °C–68 °C. 1H-NMR (CDCl3, 400 MHz): δ 7.04 (d, J = 7.2 Hz, 1H), 7.03 (s, 1H), 6.54 (s, 1H), 6.36 (d, J = 7.2 Hz, 1H), 4.04–4.10 (m, 1H), 3.90–3.96 (m, 1H), 3.44 (t, J = 6.2 Hz, 2H), 3.32 (s, 3H), 2.43 (d, J = 15.7 Hz, 1H), 2.22 (s, 3H), 1.52–2.05 (m, 16 H), 1.46 (s, 3H), 1.27 (s, 3H), 1.18 (s, 3H), 1.11 (s, 3H), 0.55 (s, 3H). 13C NMR (101 MHz, CDCl3) δ 178.3, 178.2, 170.1, 164.8, 146.0, 134.2, 127.4, 119.5, 118.2, 117.2, 69.2, 61.6, 58.7, 45.1, 44.3, 43.0, 40.4, 39.4, 38.3, 36.4, 34.8, 33.5, 32.8, 31.6, 30.8, 30.5, 29.8, 29.7, 28.7, 28.6, 21.6, 18.5, 10.3. ESI-HRMS (m/z): calcd for C33H47O5+ ([M + H]+): 523.3418; found: 523.3417. Purity: 96.9321% by HPLC (A: 0.1% Phosphoric acid in water: B: Acetonitrile, grade: B, 85%–100%), tR = 14.013 min, λ = 254 nm.
3-Phenoxypropyl (2 R,4aS,6aS,12bR,14aS,14bR)-10-hydroxy-2,4a,6a,9,12b,14a-hexamethyl-11-oxo-1,2,3,4,4a,5,6,6a,11,12b,13,14,14a,14b-tetradecahydropicene-2-carboxylate (11) Red solid; yield 54%; m.p. 72 °C–73 °C. 1H-NMR (CDCl3, 400 MHz): δ 7.28 (t, J = 7.7 Hz, 2H), 7.09 (s, 1H), 7.04 (d, J = 7.1 Hz, 1H), 6.96 (t, J = 7.4 Hz, 1H), 6.87 (d, J = 8.0 Hz, 2H), 6.53 (s, 1H), 6.31 (d, J = 7.1 Hz, 1H), 4.11–4.24 (m, 2H), 4.04 (t, J = 6.7 Hz, 2H), 2.44 (d, J = 15.7 Hz, 1H), 2.24 (s, 3H), 1.52-2.12 (m, 16 H), 1.44 (s, 3H), 1.25 (s, 3H), 1.19 (s, 3H), 1.10 (s, 3H), 0.54 (s, 3H). 13C NMR (101 MHz, CDCl3) δ 178.4, 178.3, 170.1, 164.7, 158.7, 146.0, 134.3, 129.5, 127.3, 120.8, 119.6, 118.2, 117.3, 114.3, 64.0, 61.2, 45.0, 44.2, 42.9, 40.5, 39.4, 38.2, 36.4, 34.8, 33.5, 32.9, 31.6, 30.9, 30.5, 29.8, 29.7, 28.5, 28.4, 21.6, 18.5, 10.3. ESI-HRMS (m/z): calcd for C38H49O5+ ([M + H]+): 585.3575; found: 585.3576. Purity: 98.2014% by HPLC (A: 0.1% Phosphoric acid in water: B: Acetonitrile, grade: B, 75%–99%), tR = 17.531 min, λ = 254 nm.
3-(1H-indol-1-yl)propyl (2 R,4aS,6aS,12bR,14aS,14bR)-10-hydroxy-2,4a,6a,9,12b,14a-hexamethyl-11-oxo-1,2,3,4,4a,5,6,6a,11,12b,13,14,14a,14b-tetradecahydropicene-2-carboxylate (12) Red solid; yield 57%; m.p0.108 °C–110 °C. 1H-NMR (CDCl3, 400 MHz): δ 7.65 (d, J = 7.9 Hz, 1H), 7.35 (d, J = 8.2 Hz, 1H), 7.23 (t, J = 7.6 Hz, 1H), 7.12 (t, J = 7.6 Hz, 1H), 7.08 (d, J = 3.2 Hz, 1H), 7.04 (d, J = 7.2 Hz, 1H), 7.01 (s, 1H), 6.54 (s, 1H), 6.52 (d, J = 3.2 Hz, 1H), 6.36 (d, J = 7.2 Hz, 1H), 4.24 (t, J = 6.8 Hz, 2H), 4.15 (q, J = 7.1 Hz, 1H), 3.99–4.05 (m, 1H), 3.88-3.93 (m, 1H), 2.45 (d, J = 15.7 Hz, 1H), 2.23 (s, 3H), 1.55-2.18 (m, 17 H), 1.47 (s, 3H), 1.29 (s, 3H), 1.24 (s, 3H), 1.14 (s, 3H), 0.56 (s, 3H). 13C NMR (101 MHz, CDCl3) δ 178.4, 178.1, 169.8, 164.6, 146.0, 135.8, 134.1, 128.7, 127.6, 127.4, 121.6, 121.1, 119.6, 119.5, 118.2, 117.1, 109.1, 101.6, 61.4, 60.4, 45.0, 44.2, 42.9, 40.5, 39.4, 38.2, 36.3, 34.8, 33.5, 32.9, 31.6, 30.9, 30.6, 29.8, 29.7, 29.3, 28.6, 21.6, 18.6, 10.3. ESI-HRMS (m/z): calcd for C40H50NO4+ ([M + H]+): 608.3734; found: 608.3746. Purity: 93.2766% by HPLC (A: 0.1% Phosphoric acid in water: B: Acetonitrile, grade: B, 85%–95%), tR = 17.510 min, λ = 254 nm.
3-(3-Formyl-1H-indol-1-yl)propyl (2 R,4aS,6aS,12bR,14aS,14bR)-10-hydroxy-2,4a,6a,9,12b,14a-hexamethyl-11-oxo-1,2,3,4,4a,5,6,6a,11,12b,13,14,14a,14b-tetradecahydropicene-2-carboxylate (13) Red solid; yield 51%; m.p. 102 °C–104 °C. 1H-NMR (CDCl3, 500 MHz): δ 10.01 (s, 1H), 8.31 (d, J = 7.2 Hz, 1H), 7.71 (s, 1H), 7.32-7.36 (m, 3H), 7.01 (d, J = 7.1 Hz, 1H), 6.96 (s, 1H), 6.50 (s, 1H), 6.33 (d, J = 7.2 Hz, 1H), 4.28 (t, J = 7.1 Hz, 2H), 3.96-4.06 (m, 2H), 2.38 (d, J = 15.7 Hz, 1H), 2.21 (s, 3H), 1.57–2.18 (m, 16 H), 1.45 (s, 3H), 1.26 (s, 3H), 1.19 (s, 3H), 1.11 (s, 3H), 0.52 (s, 3H). 13C NMR (126 MHz, CDCl3) δ 184.4, 178.3, 178.1, 169.6, 164.6, 146.0, 137.8, 137.0, 134.0, 127.5, 125.6, 124.2, 123.1, 122.4, 119.6, 118.5, 118.2, 117.1, 109.7, 61.2, 60.4, 45.0, 44.2, 42.9, 40.5, 39.4, 38.2, 36.3, 34.8, 33.5, 32.9, 31.6, 30.8, 30.6, 29.8, 29.7, 28.9, 28.6, 21.6, 18.7, 10.3. ESI-HRMS (m/z): calcd for C41H50NO5+ ([M + H]+): 636.3684; found: 636.3685. Purity: 93.5805% by HPLC (A: 0.1% Phosphoric acid in water: B: Acetonitrile, grade: B, 85%–95%), tR = 13.466 min, λ = 254 nm.
3-(1,3-Dioxo-1,3,3a,7a-tetrahydro-2H-isoindol-2-yl)propyl (2 R, 4aS, 6aS, 12bR, 14aS, 14bR)-10-hydroxy-2,4a,6a,9,12b,14a-hexamethyl-11-oxo-1,2,3,4,4a,5,6,6a,11,12b,13,14,14a,14b-tetradecahydropicene-2-carboxylate (14) Red solid; yield 58%; m.p. 90 °C–91 °C. 1H-NMR (CDCl3, 500 MHz): δ 7.83–7.86 (m, 2H), 7.72–7.74 (m, 2H), 7.09 (s, 1H), 7.03 (d, J = 7.1 Hz, 1H), 6.54 (s, 1H), 6.36 (d, J = 7.1 Hz, 1H), 3.92–4.15 (m, 3H), 3.76-3.81 (m, 2H), 2.42 (d, J = 15.7 Hz, 1H), 2.21 (s, 3H), 1.57–2.10 (m, 15 H), 1.46 (s, 3H), 1.26 (s, 3H), 1.19 (s, 3H), 1.09 (s, 3H), 0.54 (s, 3H). 13C NMR (101 MHz, CDCl3) δ 178.3, 178.1, 170.1, 168.2, 164.7, 146.0, 134.2, 134.0, 132.0, 127.4, 123.3, 119.6, 118.1, 117.2, 61.7, 45.1, 44.3, 43.0, 40.4, 39.5, 38.2, 36.4, 35.1, 34.8, 33.5, 32.7, 31.6, 30.8, 30.5, 29.8, 29.6, 28.6, 27.7, 21.6, 18.5, 10.3. ESI-HRMS (m/z): calcd for C40H48NO6+ ([M + H]+): 638.3476; found: 638.3477. Purity: 98.5137% by HPLC (A: 0.1% Phosphoric acid in water: B: Acetonitrile, grade: B, 85%–95%), tR = 15.169 min, λ = 254 nm.
Water solubility determination
The water solubility of compound 2 and celastrol was determined using HPLC. A series solutions of compound 2 with different concentrations (2.5 µg/mL, 10 µg/mL, 40 µg/mL, 160 µg/mL, and 640 µg/mL) in MeOH were prepared and sonicated for 10 min at 37 °C. The solutions were subjected to the HPLC to obtain the absorption peaks of compound 2 and the peak aeras were recorded. Each solution was injected three times, and a regression equation was obtained by regression analysis between the average peak area and the corresponding concentration. In the same condition, a supersaturated solution of compound 2 in water was prepared. It was standed alone for 1 h and the upper clear liquid was filtered with a microporous membrane follewed by the injection to the HPLC. The peak area was obtained and used to calculate the water solubility according to the regression equation. The water solubility of celastrol was measured by the same method.
Evaluation of anti-tumour activity and exploration of the mechanism of action
Cell culture and animals
All the human cancer cells and normal cells used in this experiment were purchased from American Type Culture Collection. The used cells include gastric cancer cell MGC-803, gastric cancer cell AGS, liver cancer cell BEL-7402, liver cancer cell HepG-2, lung cancer cell A549, colon cancer cell HCT-116, and a normal liver cell LO2. Cells were cultured in RPMI-1640 medium or DMEM medium supplemented with 10% foetal bovine serum (Gibco, US) and 1% penicillin.
The 8-week-old female Balb/c nude mice were purchased from GemPharmatech Co., Ltd., and maintained under 12 h light/dark conditions at 22 °C–24 °C and 40%–70% humidity. Procedures involving animals were performed according to the Guide for the Care and Use of Laboratory Animals (8th Edition, National Academies Press, Washington, DC), and was approved by the local animal ethics committee (Institutional Animal Ethics Committee of Jinggangshan University, approval number: 202208003).
Antiproliferative activities evaluation with MTT assay
Antiproliferative activities of compounds 1–14 as well the celastrol were investigated by the MTT assay. Cells was cultured in 96-well plates to approximately 60%–75% confluency, and then treated by the test compound with three concentrations (1, 10, and 50 μM), respectively. Three wells were set in parallel. After incubation for 48 h, the culture medium was changed, and 15 μl of MTT solution (2 mg/mL) was added. After 4 h of incubation, the MTT solution was removed and 150 ml of DMSO was added each well. The 96-well plate was shaken on a microplate oscillator for 5 min to ensure that the generated methylzan was completely dissolved. The absorbance at 492 nm was read by a microplate reader. The inhibition rate was calculated according to the measured absorbance value. Based on the inhibition rate of the three concentrations, five concentrations were reset and retested to obtain the inhibition rate. A fitting logarithmic curve was generated based on the obtained inhibition rates and the according concentration and was used to calculate the half inhibitory concentration (IC50). To comparing the safety of these compounds, the selectivity index (SI) was calculated as the ratio between IC50 in cancer cells and normal cells LO2.
Cell cycle analysis with flow cytometry
The MGC-803 cells in the exponential phase were seeded in a 6-well plate with approximately 1 × 106 cells per well. When the cells were cultured to approximately 60%–75% confluency, it was treated by compound 2 in different concentrations (0.1 µM, 0.5 µM, and 1 µM), and incubated for 24 h. 0.1% DMSO was used as the blank control, and celastrol was used as the positive control. The cells were collected, washed twice with cooled PBS, fixed with cooled 75% ethanol, and stay overnight at − 20 °C. On the second day, the fixed cells were centrifuged at 4 °C at 2000 rpm for 15 min, washed twice with cooled PBS, and then added 500ul of DNA staining solution (0.05 mg/ml PI, 0.2% Triton X-100, and 0.02 mg/ml RNase A) to stain the cells at room temperature in dark for 15-20min. The cell cycle distribution analysis was measured by flow cytometry (Becton-Dickinson, Franklin Lakes, NJ, USA). The percentage of cells in the G1, S, and G2/M phases of the cell cycle were calculated using the ModFit LT version 4.0 software package (Verity Software, Topsham, ME, USA).Citation39
Apoptosis assay with flow cytometry
The MGC-803 cells in the exponential phase were seeded in a 6-well plate with approximately 1 × 106 cells per well. When the cells were cultured to approximately 70-80% confluency, it was treated by compound 2 in different concentrations (0.1 µM, 0.5 µM, and 1 µM), and incubated for 24 h. 0.1% DMSO was used as the blank control, and celastrol was used as the positive control. The cells were collected, stained, and analysed for apoptosis on a flow cytometer.Citation40
ELISA for the expression of VEGF
Expression of VEGF in the MGC-803 cells was detected using a hVEGF ELISA Kit. Cells cultured with different conditions (1% O2: induced by 100 µM CoCl2 · 6H2O, 21% O2) were treated with compound 2 in different concentrations (0.1 µM, 0.5 µM, 1 µM) for 24 h. 0.1% DMSO was used as the blank control, and celastrol was used as the positive control. The cell culture supernatant was collected and submitted to centrifugation (1000 × G) under 4 °C for 20 min. The VEGF expression level was determined according to the instructions of Elisa Kit.Citation41
ELISA for the expression of MMP-9
Expression of VEGF in the MGC-803 cells was detected using a hMMP-9 ELISA Kit. Cells cultured with different conditions (1% O2: induced by 100 µM CoCl2 · 6H2O, 21% O2) were treated with compound 2 in different concentrations (0.1 µM, 0.5 µM, 1 µM) for 24 h. 0.1% DMSO was used as the blank control, and celastrol was used as the positive control. The cell culture supernatant was collected and submitted to centrifugation (1000 × G) under 4 °C for 20 min. The MMP-9 expression level was determined according to the instructions of Elisa Kit.Citation42
In vivo anticancer effect evaluation in nude mice
Logarithmic HCT116 cells were adjusted to the density of 1 × 108/mL and injected into the nude mice’s right armpit with a volume of 0.1 ml per mouse. The inoculated mice were randomly divided into four groups with six mice per group. The 5% DMSO in soybean oil was used as the vehicle for the tested drugs. When the tumour volume of the mice increased to about 100 mm3, the drug groups were given compound 2 (1 mg/kg and 2.5 mg/kg), and positive control 5-FU (20 mg/kg) intraperitoneally with an injection volume of 5 ml/kg. The negative control group was injected with the same vehicle. The mice were treated once two days for 21 consecutive days. The body weight and long and short diameters of the tumour body were measured every 3 d. On day 16, the mice were sacrificed and the tumours were weighed. A vernier calliper was used to measure the tumour size. The following formula was used to calculate the tumour volume (mm3): tumour volume = (A × B2)/2, (A is the length, B is the width). Tumour inhibition rate (%) = (1 − average tumour weight in the treated group/average tumour weight in the control group) × 100%. Subsequently, tumour tissue was used for the ELISA detection of TNF- α, immunohistochemical detection of VEGF, HE analysis, and western blot analysis of VEGF.
ELISA detection of TNF-α
After the execution of tumour mice in each group, the serum was obtained and placed in the serum-separating tube for 2 h. The serum was centrifuged (1000 × g) for 20 min, and the supernatant was stored at −80 °C. Mouse TNF- α ELISA kit (MM-0132M1, Jiangsu Enzyme Immunity Industry Co., Ltd.) was used to detect the TNF- α level. Six serum samples in each group and three wells for each sample were tested. The effects of compound 2 and 5-FU on the TNF- α level of tumour mice were analysed.
Haematoxylin-eosin staining (HE) analysis
HE analysis was carried out to assess the morphological changes in tumour tissue. After the execution of tumour mice in each group, the tumour tissues were collected. It was fixed with formalin solution, routinely dehydrated, transparent, embedded, sectioned, xylene dewaxing, gradient ethanol hydration, stained with haematoxylin- eosin (HE), gradient alcohol dehydrated, and finalised. The slice was observed for the pathological changes of mouse tumours under an optical microscope (BX43, Olympus). The nucleus is blue and the cytoplasm is red in the photograph.
Immunohistochemical detection of VEGF expression
The expression level of VEGF in the tumour tissue was detected by immunohistochemistry. After the sections of tumour tissue were baked, dewaxed, and hydrated, the antigen was repaired with citric acid buffer. After being blocked by 5% BSA antigen, the primary antibody was incubated with VEGF (1:100) at 4 °C overnight and then incubated with horseradish enzyme-labeled goat anti-rabbit IgG (H + L) (1:100). After being coloured by DAB, re-stained with haematoxylin, dehydrated and transparent, the sealed slice was observed under a microscope (BX43, Olympus). The nucleus stained with haematoxylin was blue, and the positive expression of VEGF was brown. The expression level of VEGF was quantitatively analysed by ImageJ software.
Western blot analysis of VEGF expression
After the execution of tumour mice in each group, a certain amount of tumour tissue was mixed with RIPA lysate, ground with a tissue grinder to extract the total tissue protein. The mixture was centrifuged (12 000 r/min) at 4 °C for 10 min. The supernatant was used to quantify the total protein with BCA protein quantitative kit. After denaturing the protein sample, the protein samples were separated by sodium dodecyl benzene sulphonate gel electrophoresis (SDS-PAGE) for 1.5 h and transferred to a PVDF Hybond-P membrane (Millipore, Billerica, MS, USA). The membranes were blocked with PBS containing 5% non-fat milk and then incubated with primary antibodies against VEGF and GAPDH overnight at 4 °C. The PVDF membranes were next incubated with secondary antibodies for 2 h. The PVDF membranes were soaked with luminescent liquid and then developed in an ultra-high sensitivity chemiluminescence imaging system. The expression level of VEGF was quantitatively analysed by ImageJ software.
Toxicity evaluation by zebrafish model
Embryonic acute toxicity test
Normal-developed zebrafish embryos at 6hpf were selected under a microscope and fed in a 24‐well plate, with six eggs per well.Citation43 The exposure concentrations of celastrol were 0.1, 0.2, 0.5, 1, and 2 μM. The exposure concentrations of compound 2 were 0.1, 0.15, 0.2, 0.3, and 0.5 μM. 0.5% DMSO was used as the blank control. Exposure continued for three days. The incubation rate and mortality were observed and recorded every 24 h. The hatching is recorded by the shell without the egg’s membrane. Mortality is recorded by the cardiac arrest of the embryo and larva. Incubation rate = (number of hatching embryos/number of the total embryo) × 100%; Mortality rate = (number of dead embryos/number of total larva) × 100%.
Adult fish acute toxicity test
Normal-developed larvae at 3 dpf were selected under a microscope and fed in a 24‐well plate, with six eggs per well. The exposure concentrations of celastrol and compound 2 were 0.1, 0.2, 0.3, 0.4, and 0.5 μM. The mortality rate was recorded at 24 h and 72 h.
Supplemental Material
Download PDF (4.4 MB)Disclosure statement
The authors report no conflicts of interest.
Additional information
Funding
References
- Sung H, Ferlay J, Siegel RL, Laversanne M, Soerjomataram I, Jemal A, Bray F. Global cancer statistics 2020: GLOBOCAN estimates of incidence and mortality worldwide for 36 cancers in 185 countries. CA Cancer J Clin. 2021;71(3):209–249.
- Marra A, Curigliano G. Adjuvant and neoadjuvant treatment of triple-negative breast cancer with chemotherapy. Cancer J. 2021;27(1):41–49.
- Lu M, Zheng J, Xu N, Lin H, Wan S. Postoperative chemotherapy as adjuvant treatment for endometrioid adenocarcinoma: early stage vs late stage. Cancer Chemother Pharmacol. 2019;84(2):299–305.
- Bray F, Møller B. Predicting the future burden of cancer. Nat Rev Cancer. 2006;6(1):63–74.
- Bekaii-Saab T, Kim R, Kim TW, O’Connor JM, Strickler JH, Malka D, Sartore-Bianchi A, Bi F, Yamaguchi K, Yoshino T, et al. Third- or later-line therapy for metastatic colorectal cancer: reviewing best practice. Clin Colorectal Cancer. 2022;21(4):e117–e129.
- Yang L, Wen KS, Ruan X, Zhao YX, We F, Wang Q. Response of plant secondary metabolites to environmental factors. Molecules. 2018; 23(4):762.
- Monneret C. Impact actuel des produits naturels sur la découverte de nouveaux médicaments anticancéreux [Current impact of natural products in the discovery of anticancer drugs]. Ann Pharm Fr. 2010;68(4):218–232.
- Deng LJ, Qi M, Li N, Lei YH, Zhang DM, Chen JX. Natural products and their derivatives: Promising modulators of tumor immunotherapy. J Leukoc Biol. 2020;108(2):493–508.
- Kubczak M, Szustka A, Rogalińsk M. Molecular targets of natural compounds with anti-cancer properties. Int J Mol Sci. 2021; 22(24):13659.
- Kannaiyan R, Shanmugam MK, Sethi G. Molecular targets of celastrol derived from Thunder of God Vine: potential role in the treatment of inflammatory disorders and cancer. Cancer Lett. 2011;303(1):9–20.
- Luo D, Guo Y, Cheng Y, Zhao J, Wang Y, Rong J. Natural product celastrol suppressed macrophage M1 polarization against inflammation in diet-induced obese mice via regulating Nrf2/HO-1, MAP kinase and NF-κB pathways. Aging. 2017;9(10):2069–2082.
- Petronelli A, Pannitteri G, Testa U. Triterpenoids as new promising anticancer drugs. Anticancer Drugs. 2009;20(10):880–892.
- Narayan V, Ravindra KC, Chiaro C, Cary D, Aggarwal BB, Henderson AJ, Prabhu KS. Celastrol inhibits Tat-mediated human immunodeficiency virus (HIV) transcription and replication. J Mol Biol. 2011;410(5):972–983.
- Peng B, Xu L, Cao F, Wei T, Yang C, Uzan G, Zhang D. HSP90 inhibitor, celastrol, arrests human monocytic leukemia cell U937 at G0/G1 in thiol-containing agents reversible way. Mol Cancer. 2010;9:79.
- Salvador JAR, Leal AS, Valdeira AS, Gonçalves BMF, Alho DPS, Figueiredo SAC, Silvestre SM, Mendes VIS. Oleanane-, ursane-, and quinone methide friedelane-type triterpenoid derivatives: Recent advances in cancer treatment. Eur J Med Chem. 2017;142:95–130.
- Tang K, Huang Q, Zeng J, Wu G, Huang J, Pan J, Lu W. Design, synthesis and biological evaluation of C6-modified celastrol derivatives as potential antitumor agents. Molecules. 2014;19(7):10177–10188.
- Han X, Sun S, Zhao M, Cheng X, Chen G, Lin S, Guan Y, Yu X. Celastrol stimulates hypoxia-inducible factor-1 activity in tumor cells by initiating the ROS/Akt/p70S6K signaling pathway and enhancing hypoxia-inducible factor-1α protein synthesis. PLOS One. 2014;9(11):e112470.
- H, Yang H, D, Chen D, QC, Cui X, Yuan QP. Dou Celastrol, a triterpene extracted from the Chinese “Thunder of God Vine,” is a potent proteasome inhibitor and suppresses human prostate cancer growth in nude mice. Cancer Res. 2006;66(9):4758–4765.
- Huang L, Zhang Z, Zhang S, Ren J, Zhang R, Zeng H, Li Q, Wu G. Inhibitory action of Celastrol on hypoxia-mediated angiogenesis and metastasis via the HIF-1α pathway. Int J Mol Med. 2011;27(3):407–415.
- Huang S, Tang Y, Cai X, Peng X, Liu X, Zhang L, Xiang Y, Wang D, Wang X, Pan T. Celastrol inhibits vasculogenesis by suppressing the VEGF-induced functional activity of bone marrow-derived endothelial progenitor cells. Biochem Biophys Res Commun. 2012;423(3):467–472.
- Kang H, Lee M, Jang SW. Celastrol inhibits TGF-β1-induced epithelial-mesenchymal transition by inhibiting Snail and regulating E-cadherin expression. Biochem Biophys Res Commun. 2013;437(4):550–556.
- Kashyap D, Sharma A, Tuli HS, Sak K, Mukherjee T, Bishayee A. Molecular targets of celastrol in cancer: recent trends and advancements. Crit Rev Oncol Hematol. 2018;128:70–81.
- Tang WJ, Wang J, Tong X, Shi JB, Liu XH, Li J. Design and synthesis of celastrol derivatives as anticancer agents. Eur J Med Chem. 2015;95:166–173.
- Jiang F, Wang HJ, Bao QC, Wang L, Jin YH, Zhang Q, Jiang D, You QD, Xu XL. Optimization and biological evaluation of celastrol derivatives as Hsp90-Cdc37 interaction disruptors with improved druglike properties. Bioorg Med Chem. 2016;24(21):5431–5439.
- Hou W, Liu B, Xu H. Celastrol: Progresses in structure-modifications, structure-activity relationships, pharmacology and toxicology. Eur J Med Chem. 2020;189:112081.
- Bai X, Fu RJ, Zhang S, Yue SJ, Chen YY, Xu DQ, Tang YP. Potential medicinal value of celastrol and its synthesized analogues for central nervous system diseases. Biomed Pharmacother. 2021;139:111551.
- Han Y, Dong W, Guo Q, Li X, Huang L. The importance of indole and azaindole scaffold in the development of antitumor agents. Eur J Med Chem. 2020;203:112506.
- Wan Y, Li Y, Yan C, Yan, Tang MZ. Indole: a privileged scaffold for the design of anti-cancer agents. Eur J Med Chem. 2019;183:111691.
- Tian DL, Liang CP, Liang J, Chen H. Synthesis and antitumor activity of novel indole podophyllotoxin derivatives. Zhongguo Zhong Yao Za Zhi. 2019;44(12):2532–2537.
- Sang YL, Zhang WM, Lv PC, Zhu HL. Indole-based, antiproliferative agents targeting tubulin polymerization. Curr Top Med Chem. 2017;17(2):120–137.
- Han L, Li T, Miao D, Lee J, Xiao S, Piao H, Zhao Y. Design, synthesis and cytotoxicity evaluation of novel indole derivatives of panaxadiol. Chem Biodivers. 2022;19(8):e202200372.
- Berlinck RGS, Crnkovic CM, Gubiani JR, Bernardi DI, Ióca LP, Quintana-Bulla JL. The isolation of water-soluble natural products – challenges, strategies and perspectives. Nat Prod Rep. 2022;39(3):596–669.
- Joshi G, Singh PK, Negi A, Rana A, Singh S, Kumar R. Growth factors mediated cell signalling in prostate cancer progression: implications in discovery of anti-prostate cancer agents. Chem Biol Interact. 2015;240:120–133.
- Qi S, Deng S, Lian Z, Yu K. Novel drugs with high efficacy against tumor angiogenesis. Int J Mol Sci. 2022;23:6934.
- Song Z, Wang J, Su Q, Luan M, Chen X, Xu X. The role of MMP-2 and MMP-9 in the metastasis and development of hypopharyngeal carcinoma. Braz J Otorhinolaryngol. 2021;87(5):521–528.
- Anderson GM, Nakada MT, DeWitte M. Tumor necrosis factor-alpha in the pathogenesis and treatment of cancer. Curr Opin Pharmacol. 2004;4(4):314–320.
- Roșu MC, Mihnea PD, Ardelean A, Moldovan SD, Popețiu RO, Totolici BD. Clinical significance of tumor necrosis factor-alpha and carcinoembryonic antigen in gastric cancer. J Med Life. 2022;15(1):4–6.
- Zhao K, Jiang Y, Zhang J, Shi J, Zheng P, Yang C, Chen Y. Celastrol inhibits pathologic neovascularization in oxygen-induced retinopathy by targeting the miR-17-5p/HIF-1α/VEGF pathway. Cell Cycle. 2022;21(19):2091–2108.
- Ma J, Li J, Tian YS. Synthesis and bioactivity evaluation of 2,3-diaryl acrylonitrile derivatives as potential anticancer agents. Bioorg Med Chem Lett. 2017;27(1):81–85.
- Zhang HJ, Zhang GR, Piao HR, Quan ZS. Synthesis and characterisation of celastrol derivatives as potential anticancer agents. J Enzyme Inhib Med Chem. 2017;33(1):190–198.
- Rosen R, Vagaggini T, Chen Y, Hu DN. Zeaxanthin inhibits hypoxia-induced VEGF secretion by RPE cells through decreased protein levels of hypoxia-inducible factors-1α. Biomed Res Int. 2015;2015:687386.
- Ward MP, Spiers JP. Protein phosphatase 2A regulation of markers of extracellular matrix remodelling in hepatocellular carcinoma cells: functional consequences for tumour invasion. Br J Pharmacol. 2017;174(10):1116–1130.
- Wang G, Xiao Q, Wu Y, Wei YJ, Jing Y, Cao XR, Gong ZN. Design and synthesis of novel celastrol derivative and its antitumor activity in hepatoma cells and antiangiogenic activity in zebrafish. J Cell Physiol. 2019;234(9):16431–16446.