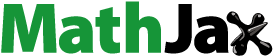
Abstract
Despite the crucial role of CDK2 in tumorigenesis, few inhibitors reached clinical trials for managing lung cancer, the leading cause of cancer death. Herein, we report combinatorial stereoselective synthesis of rationally designed spiroindeno[1,2-b]quinoxaline-based CDK2 inhibitors for NSCLC therapy. The design relied on merging pharmacophoric motifs and biomimetic scaffold hopping into this privileged skeleton via cost‐effective one-pot multicomponent [3 + 2] cycloaddition reaction. Absolute configuration was assigned by single crystal x-ray diffraction analysis and reaction mechanism was studied by Molecular Electron Density Theory. Initial MTT screening of the series against A549 cells and normal lung fibroblasts Wi-38 elected 6b as the study hit regarding potency (IC50 = 54 nM) and safety (SI = 6.64). In vitro CDK2 inhibition assay revealed that 6b (IC50 = 177 nM) was comparable to roscovitine (IC50 = 141 nM). Docking and molecular dynamic simulations suggested that 6b was stabilised into CDK2 cavity by hydrophobic interactions with key aminoacids.
Introduction
Cyclin-dependent kinase 2 (CDK2) is a key mediator of multiple oncogenic signalling pathways. Its activity is crucial for loss of proliferative control during tumorigenesisCitation1. Initial interest in drugging CDK2 as anticancer target was tempered by realising that CDK2 inhibition using antisense oligonucleotides, anti-CDK2 shRNA or a dominant-negative CDK2 failed to arrest cancer cells proliferationCitation2. These methods resulted in ablation of CDK2 expression at the protein level, possibly allowing compensation by other related CDKs, and therefore they are likely to exert different effects than acute CDK2 kinase inhibition using small molecules. Further examination of various human cancers with defined molecular features for CDK2 inhibition susceptibility has unveiled the optimum scope of CDK2 targeting. For example, in cancers with amplified Cyclin E1 (CCNE1) expression such as ovarian cancerCitation3 and non-small cell lung cancer cells (NSCLC) with special focus on KRAS mutated subtype, the most frequently mutated oncogenes in human NSCLCCitation4,Citation5. Along these observations, CDK2 knockout animals showed no apparent abnormalities pointing to the conclusion that CDK2 inhibitors might have preferentially targeted tumour cells, sparing the normal tissuesCitation6. Evidences supporting the therapeutic role of CDK2 inhibition in cancer has also been manifested by several combination studiesCitation7–11. Interestingly, CDK2 inhibition could revert the acquired resistance to CDK4/6 inhibitorsCitation12,Citation13. It is worth mentioning that the therapeutic applications of CDK2 inhibitors are also expanding beyond oncology into other clinical settings including infectious diseases Citation14 and neurodegenerative disordersCitation15. However, few inhibitors reached clinical studies as anticancer agents of which some have been discontinued due to the associated side effects (e.g. AZD5438, SNS-032 and R547) or failure to achieve the acceptable clinical outcome (e.g. AG-024322)Citation16,Citation17. Alvocidib (flavopiridol)Citation18,Citation19, the first clinical-stage CDK inhibitor induces G1 and G2 cell cycle arrest due to inhibition of CDK2/4 and CDK1 activities, respectively. It has been studied clinically, as a single agent or in combinations, but demonstrated unsatisfactory safety and efficacy. Seliciclib (roscovitine)Citation20,Citation21, a purine analog and the second inhibitor to reach clinical trials, was identified as a pan-CDK inhibitor. Despite the ongoing reasearch introducing other lead CDK2 inihibitors for clinical managment of different types of cancers, relatively few studies were focusing on halting lung cancerCitation22, the leading cause of cancer death, accounting for over 2 million deaths worldwide in 2020Citation23. For instance, the next-generation CDK2/9 inhibitors, CCT68127 and CYC065, induced apoptosis through anaphase catastrophe in lung cancer cell linesCitation24. When administered to quiescent NSCLC cells, the CDK2 inhibitor SNS-032 increased the tumour radiosensitivityCitation25. AZD5438, also enhanced the radiosensitivity of NSCLCCitation26.
Most recently, our group has introduced series of spirooxindole-based CDK2 inhibitors surpassing roscovitine, via cost‐effective single‐step multicomponent [3 + 2] cycloaddition (32CA) reaction allowing easy access to combinatorial libraries. These leads exhibited efficient tumour-selective cytotoxic activities against breast and liver cancersCitation27. Inspired by these findings, we speculated that combination of CDK2 inhibitors pharmacophores as well as motifs from efficient anti-lung cancer agents into this multifunctionalized privileged skeleton could facilitate the discovery of new lead molecules for targeting NSCLC. Accordingly, herein we reported combinatorial stereoselective synthesis of rationally designed hybrids with absolute configuration assigned by single crystal x-ray diffraction analysis of representative derivative and reaction mechanism studies. All derivatives were then subjected to cytotoxicity screening against A549 NSCLC cells and in vitro CDK2 inhibition evaluation guided by docking and molecular dynamic simulation studies to aid identifying the possible binding modes, stability and structural determinants of activity.
Design rationale
Building upon the benchmark spiro CDK2 inhibitors with anti-breast cancer potential, we adopted pharmacophoric hybridisation and biomimetic scaffold hopping strategies to develop efficient CDK2 inhibitors for NSCLC management. Accordingly, we started from spirooxindole I () being a privileged framework. The oxindole fragment was replaced by quinoxaline; the pharmacophoric core of erdafitinib that was recently found to inhibit tumorigenesis of NSCLC A549 cells via CDK2 inhibitionCitation28. With that, a pioneer study probing the effect of indeno ring substitution pattern and fusion to a heterocyclic motif on CDK2 inhibition potentialCitation29 directed our design rationale to assemble indeno[1,2-b]quinoxaline-based spiro compounds. Literature review for pharmacophoric fragments to be installed into the target scaffold highlighted benzimidazole as the most common motif in anticancer agents targeting NSCLC with KRAS mutation following screening a drug library of 1271 small moleculesCitation30. It comes as no surprise also that benzimidazole has been widely represented in CDK2 inhibitorsCitation31,Citation32. A biomimetic scaffold hopping approach was employed where hexahydro-1H-pyrrolizine was selected as the spiro ring inspired by the natural mitomycin; a first-generation anticancer agent that has been directed to advanced NSCLCCitation33. Moreover, the design strategy adopted broadening the chemical space of the designed compounds and influencing their electronic and steric environment, to perform SAR study, via diversifying the terminal aromatic substitution and the installed spiro ring as hexahydro-1H-pyrrolizine and (9aR)-decahydro-1H-pyrrolo[1,2-a]indole, while conserving the heterocyclic scaffold. Therefore, a library of 16 new derivatives was successfully synthesised, then subjected to computational studies and biological evaluation.
Results and discussion
Chemistry
Despite the tremendous importance of indenoquinoxaline heterocyclic derivatives due to their biological activities, there are only a few studies available on the assembly of spiropyrrolidine derivatives in a single structural framework containing both bioactive classes. In Scheme 1, the targeted new structurally complex and diverse benzimidazole-tethered indenoquinoxaline-based spiro-heterocycles 4a-h and 6a-h were synthesised. Considering the growing interest in multicomponent reactions gaining access to bioactive spiropyrrolidine derivativesCitation34,Citation35, we have synthesised the the title spiro compounds 4a-h and 6a-h via 32CA reaction of 11H-indeno[1,2-b]quinoxalin-11-one 2 with the benzimidazolyl chalcones 1a-h and L-proline or octahydroindole-2-carboxylic acid. The products of cycloaddition reaction were separated using silica gel (petroleum ether/ethyl acetate (4:1)) column chromatography. The structures of all compounds 4a-h and 6a-h were established using IR, 1H- and 13C-NMR spectroscopy, and elemental analysis as detailed in the experimental section. Moreover, the absolute configuration of a representative derivative was assigned by X-ray diffraction analysis of single crystal.
It is worth mentioning that the reaction of chalcones 1a-h with stabilised azomethine ylides generated from indenoquinoxalinone 2, cyclic amino acids 3 and 5 are accompanied by binding of a more electrophilic β-C atom of the ethylene derivative with the less substituted C-3 atom of the three-atom-component (TAC) of the cycloaddition process.
Crystal structure of compound 4e
To establish the absolute configuration of the synthesised compounds 4a-h and 6a-h, we performed X-ray diffraction analysis of single crystals of 4e, which were isolated via crystallisation from ethanol/CH2Cl2. The general form of molecule 4e is shown in , and the main geometrical parameters of compound 4e were listed in tables in the supplementary material (Table S1–S3, supplementary material) along with technical procedure for data collection and refinmentsCitation36–39.
MEDT study of the 32CA reaction between AY 7 and ethylene 1h
In this section, the 32CA reaction of AY 7 with the electrophilic ethylene 1h yielding the spiro-indeno[1,2-b]quinoxaline 4h was theoretically studied with Molecular Electron Density TheoryCitation40 (MEDT).
Analysis of the DFT-based reactvity indices at the GS of the reagents
The analysis of the DFT-based reactvity indicesCitation41,Citation42 at the ground state (GS) of the reagents have shown to be powerful tool to understand the reactivity in polar reactionsCitation43. The global reactivity indices, namely, the electronic chemical potential μ, chemical hardness η, electrophilicity ω and nucleophilicity N indices, for AY 7 and ethylene 1h are gathered in .
Table 1. B3LYP/6-31G(d) electronic chemical potential μ, chemical hardness η, electrophilicity ω and nucleophilicity N indices, in eV, of AY 7 and ethylene 1h.
The electronic chemical potentials μCitation44 of AY 7, μ = −3.01 eV, is above than that of ethylene 1h, μ = −4.26 eV, indicating that along a polar 32CA reaction the global electron density transfer Citation45 (GEDT) will take place from AY 7 to the ethylene 1h, the 32CA reaction being classified as the forward electron density fluxCitation46 (FEDF).
AY 7 presents an electrophilicity ω indexCitation47 of 1.49 eV, being classified as moderate electrophile within the electrophilicity scaleCitation42, and a nucleophilicity N indexCitation48 of 4.60 eV, being classified as a strong nucleophile within the electrophilicity scaleCitation42. The very strong nucleophilic character of AY 7, higher than 4.0 eV, allows its classification as supernucleophile participating in high polar reactionsCitation43.
Ethylene 1h presents an electrophilicity ω index of 2.35 eV, being classified as a strong electrophile within the electrophilicity scale. On the other hand, 1h presents a nucleophilicity N index of 2.92 eV, being classified as moderate nucleophiles within the nucleophilicity scale.
The supernucleophilic character of AY 7 together with the strong electrophilic character of ethylene 1h suggests that the corresponding 32CA reaction of FEDF will have a high polar character.
Study of the 32CA reaction of AY 7 with electrophilic ethylene 1h
Due to the non-symmetry of both reagents, two pairs of endo and exo stereoisomeric and two pairs of ortho and meta regioisomeric reaction paths are feasible. The four competitive reaction paths were studied (see Scheme 2). Analysis of the stationary points found in the four reaction paths indicates that this 32CA reaction takes place though a one-step mechanism. The ωB97X-D/6-311G(d,p) relative enthalpies and Gibbs free energies are given in . Electronic energies and the thermodynamic data are given in Table S4 in Supplementary Material.
Table 2. ωB97X-D/6-311G(d,p) relative enthalpies (ΔH in kcal·mol−1), entropies (ΔS in cal·mol−1K−1), and Gibbs free energies (ΔG in kcal·mol−1), with respect to the separated reagents, computed at 337.75 K and 1 atm in methanol, for the stationary points involved in the 32CA reaction of AY 7 with ethylene 1h.
A series of molecular complexes (MCs) in which the two reagents are already joined by weak intermolecular interactions were also found. Only the most stable of them, MC-on, was selected as the energy reference. The distance between the two frameworks at this MC is ca. 3.2 Å; MC-on is found 26.0 kcal·mol−1 below the separated reagents (see ). Some appealing conclusion can be obtained from the gas relative enthalpies given in : (i) the most favourable TS-on is found 18.1 kcal·mol−1 below the separated reagents, but if the formation of MC-on is considered, the activation enthalpy becomes positive by 7.9 kcal·mol−1; (ii) this 32CA reaction is completely endo stereoselective as TS-ox is found 3.1 kcal·mol−1 above TS-on; (iii) this 32CA reaction is completely ortho regioselective as TS-mx is found 3.0 kcal·mol−1 above TS-on. Both endo stereoselectivity and ortho regioselectivity are in complete agreement with the experimental outcomes; and iv) this 32CA reaction is strongly exothermic as spiro compound 4h is found 48.8 kcal·mol−1 below the separated reagents. Consequently, formation of spiro compound 4h is attained by a kinetic control.
A representation of the enthalpy and Gibbs free energy profiles associated with the four competitive reaction paths is given in . Inclusion of the thermal corrections and entropies to enthalpies increases the relative Gibbs free energies by between 16.7 and 19.5 kcal·mol−1 as a consequence of the unfavourable activation entropies associated with this bimolecular process, which are found in the range −49.3 and −57.7 cal·mol−1·K−1. Formation of MC-on is exergonic by 9.3 kcal·mol−1. The activation Gibbs free energy associated with the 32CA reaction of AY 7 with ethylene 1h via TS-on rises to 10.7 kcal·mol−1, while the formation of spiro compound 4h is exergonic by 30.3 kcal·mol−1. Considering the activation Gibbs free energies, this 32CA reaction is completely endo stereoselective and ortho regioselective as TS-ox and TS-mn are located 3.0 and 2.0 kcal·mol−1, respectively, above TS-on (see ).
Figure 3. ωB97X-D/6-311G(d,p) enthalpy, in blue, ΔH in kcal mol−1, and Gibbs free energy, in red, ΔG in kcal mol−1, profiles, in methanol at 65 °C, for the 32CA reaction of AY 7 with the ethylene 1h.
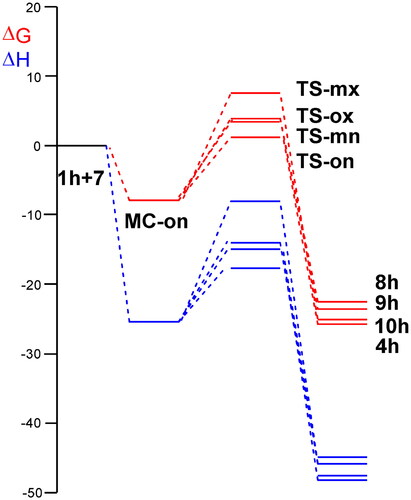
Considering that this 32CA reaction takes place under favourable kinetic control, the Eyring-Polanyi relationCitation49, which allows to correlate the relative reaction rate constants krel with the relative activation Gibbs free energies ΔΔG≠, was used to estimate the composition of the reaction mixture. Considering the Gibbs free energies associated to TS-on, TS-ox, TS-mn and TS-mx, given in , and the reaction temperature, 65 °C, the following relationship 94.1 (4h): 1.1 (8h): 4.8 (9h): 0.0 (10h) between the four isomeric spiro compounds can be estimated, in reasonable agreement with the experimental outcome, in which only spiro compound 4h is obtained.
The geometries of the four TSs optimised in methanol are given in . The C − C distances between the four interacting carbons at the four TSs indicate that, less the most unfavourable TS-mx, the other three TSs correspond with high asynchronous C − C single bond formation processes, in which the shorter C − C distance corresponds to that involving the participation of the most electrophilic β-conjugated C4 carbon of ethylene 1h. Note that at the most favourable TS-on, the C − C distances between the two pair of interacting carbons are 2.110 (C3 − C4) and 2.794 (C1 − C5) Å. Analysis of the intrinsic reaction coordinatesCitation50 associated to the high asynchronous TS-on indicates that this 32CA reaction takes place through a non-concerted two-stage one-step mechanismCitation51 in which formation of the second C − C single bond begins when the first C − C single bond is completely formed.
Figure 4. ωB97X-D/6-311G(d,p) geometry obtained in methanol of the TSs involved in the 32CA reaction of AY 7 with ethylene 1h. The distances are given in Angstrom.
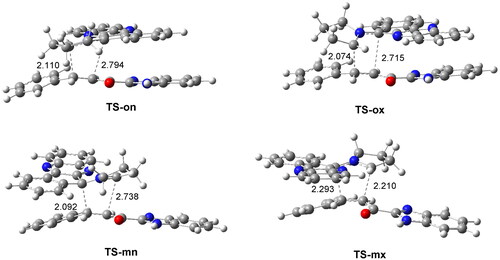
Finally, analysis of GEDT at the most favourable TS-on permits assessment of the polar character of this 32CA reaction. GEDT values lower than 0.05 e correspond with non-polar processes, while values higher than 0.20 e correspond with high polar processes. The computed GEDT value at TS-on is 0.34 e. This high value, which is a consequence of the supernucleophilic character of AY 7 and the strong nucleophilic character of ethylene 1h (see ), indicates that this 32CA reaction has high polar character. The flux of the electron density, which goes form AY 7 to ethylene 1h, classifies this 32CA reaction as FEDF, in clear agreement with the analysis of the reactivity indices.
Cytotoxicity screening of 4(a-h) and 6(a-h)
All the synthesised compounds were preliminarily screened via MTT assay for their cytotoxicity on normal human lung fibroblasts (Wi-38) to evaluate their safety and selectivity profiles in terms of EC100 and IC50. Then, the compounds were evaluated for their potential in vitro anticancer activities against human lung cancer A549 compared to 5-fluorouracil (5-FU) utilising MTT assay ().
Table 3. Cytotoxicity screening of 4(a-h) and 6(a-h) against human lung fibroblasts (Wi-38) and lung cancer cells (A549) and their selectivity index (SI) values.
All the screened compounds were more active than the reference chemotherapy 5-FU against A549 cells with 6b (IC50 = 0.054 µM) at the top of the list followed by the comparable derivatives 4a, 6 g, 6d, 6a and 6f recording IC50 values ranging from 0.082 to 0.107 µM. Nearly equipotent cytotoxic activities (IC50 = 0.135–0.146 µM) were observed for 4d, 6e, 6c, and 4f. The moderately active derivatives 4h, 4 g, 6h, 4c and 4b exhibited slighly lower potency (IC50 = 0.177–0.225 µM), whereas 4e (IC50 = 0.321 µM) was the least active derivative among the evalauted series. While potency is an important consideration, assessing the compound’s selectivity to A549 cancer cells over Wi-38 normal lung fobroblasts expressed as compound’s selectivity index (SI) is key to lead identification. Hence, the selectivty indices of the tested compounds were calculated. Herein, the most potent derivatives 6b and 4a displayed the highest selectivity (SI = 6.648 and 7.402, respectively) followed by 6f (SI = 5.130). 4b, 4e and 4f were moderately selective (SI = 3.942, 3.429 and 3.082, respectively). Other derivatives were beyond the acceptable selectivity limit (SI˂3), hampering their further evaluation.
A546 cells were examined morphologically after treatment with 6b in comparison with 5-FU-treated cells and the untreated ones (). As seen, the cancerous cells treated with 6b lost their characteristic shape and suffered severe shrinkage due to the potent apoptotic potential of the evaluated compound relative to the control.
Docking and molecular dynamics simulations into CDK2 active site
Among the evaluated series, only potent derivatives with acceptable selectivity profile (SI > 3) were subjected to virtual screening protocol involving docking and molecualr dynamic simulations to identify the hit compound for further in vitro CDK2 inhibition studies. Using the MOE docking suit, the title derivatives namely 4a, 4b, 4e, 4f, 6b and 6f were docked into the active site of CDK2 and ranked based on their docking scores. Results (Supplemetary data; Table 5S) indicated that 6b was the top ranked derivative (ΔG = −11.44 Kcal/mol). Inspection of the most stable docking poses within CDK2, revealed that most compounds could bind favourably with the catalytic domain (Figure 1S, supplementary material). The binding mode of the hit spiro derivative 6b into the active site of CDK2 was then investigated in comparison to the reference CDK2 inhibitor roscovitine ( and ). As illustrated in , roscovitine could bind with the ATP-binding pocket, where its purine ring occupied a region that closely aligned with the purine ring of ATP. Both ring systems generally overlapped within the same plane, but there is a noticeable difference in the orientation of roscovitine’s purine ring compared to ATP within the protein structure. In this particular orientation, the N7 of roscovitine was proximate to the position occupied by the N1 in ATP’s purine ring. Additionally, the benzyl ring extended outward from the ATP-binding pocket. The interaction between roscovitine and CDK2 is primarily characterised by hydrophobic and van der Waals interactions, engaging the same hydrophobic residues within the enzyme that typically form the pocket for ATP’s adenine base in the ATP complex. Besides hydrophobic and van der Waals interactions, hydrogen bonds were also observed between the ligand and protein, involving two hydrogen bonds linking the purine ring nitrogens with Phe83 at a distance of 2.8 and 3.4 Å. While a third bond was observed between the oxygen of the ligand and Glu12 at a distance of 3.5 Å.
Figure 6. Binding pose of the reference drug roscovitine within the active site of CDK2 protein. The dashed black line depicts hydrogen bond interaction.
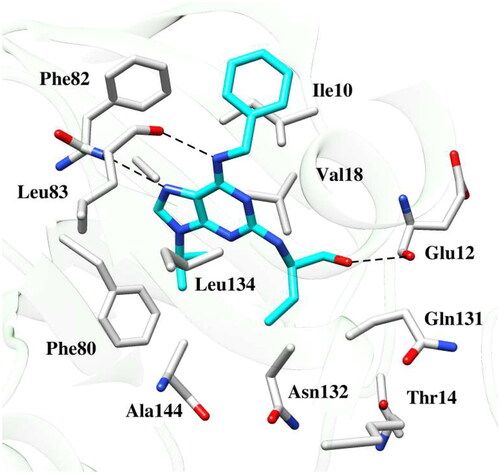
Figure 7. Binding poses of the active compound 6b with CDK2 protein (a) Docking pose (b) MD simulation pose. The protein residues of the target CDK2 protein are presented as light grey. The dashed black line depicts hydrogen bond interaction.
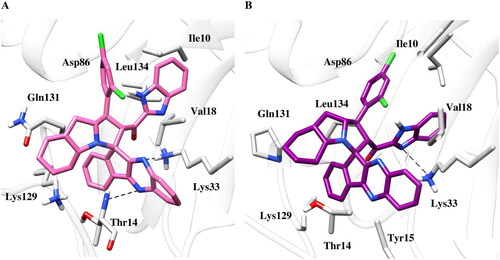
depicted the binding mode of 6b within CDK2. The terminal phenyl ring exhibited pi-pi stacking interaction face-to-face with the aromatic ring of Tyr15. Both nitrogen of quinoxaline ring demonstrated hydrogen bond interactions with the backbone and side chain of Thr14 and Lys33 at a distance of 2.9 and 1.8 Å, respectively. The compound was further stabilised by hydrophobic contacts with Ile10, Tyr15, Val18, Lys33, Phe82, Lys129 and Gln131.
In order to comprehend the dynamic behaviour of the hit compound 6b, a short simulation of 100 ns was performed using AMBER22. Simulation trajectories were analysed (). The overall stability of system and quality of the simulation were assessed by analysing the root mean square deviation (RMSD), root mean square fluctuation (RMSF) and radius of gyration (RoG) (). The RMSD of the heavy atoms of main chain of protein was calculated using the “rms” command in CPPTRAJ. depicts the RMSD deviation of heavy atoms of protein backbone. As evident, the system showed stability with an average RMSD value of 2.7 Å. This observation was further supported by investigating RoG, which suggests that the systems was well-compacted (). Further, to understand the dynamics of side chains of residues, RMSF of the protein was calculated as a function of time (). The results highlighted the amino acid residues in the protein–ligand complex were stable after binding with the active compound of the series.
The visual analysis of simulation trajectories suggested that compound 6b () stabilises in the cavity of CDK2 by strongly mediating hydrophobic and pi-pi interaction with Tyr15, Val18, Ile35, Phe80, Leu134 and Val163. Further the nitrogen of quinoxaline ring exhibits pi-cation interaction with Lys33. A part of hydrophobic interaction, the nitrogen of the imidazole ring is involved in hydrogen bond interaction involving the side chain of Lys33 with the occupancy of 85%. Collectively, these pieces of evidence proposed the crucial role of hydrophobic interaction in lowering the solvation energy. During simulation, most of the protein-ligand interaction was found consistent with the docking pose.
In light of the aforementioned in silico results, we selected 6b for further in vitro enzymatic studies to evaluate its potential as a CDK2 inhibitor.
In vitro CDK2 inhibition
As shown in and , compound 6b exhibited promising CDK2 inhibition with an IC50 value of 177.4 nM and 70.7% CDK2 inhbition at 10 μM compared to roscovitine (IC50 = 141 nM and 89.6% CDK2 inhibition at 10 μM). These results highlighted the potency of 6b as CDK2 inhibitor and suggested that CDK2 may be its main molecular target in A549 cells.
Figure 11. Dose-response curve for the CDK2 inhibition versus serial dilutions of compound 6b and roscovitine [0.01–10 μM].
![Figure 11. Dose-response curve for the CDK2 inhibition versus serial dilutions of compound 6b and roscovitine [0.01–10 μM].](/cms/asset/a83ca270-1791-4009-a26c-a1ebb34d3ba8/ienz_a_2281260_f0011_b.jpg)
Table 4. Summarised IC50 values with the percentage of CDK2 inhibition of compound 6b and roscovitine.
Structure-activity relationship
The general cytotoxicity pattern of the screened series 4a-h and 6a-h highlighted the promising potency of the designed scaffold against A549 cells. However, the relative activity and, to a greater extent, the selectivity were found to be a function of the spiro ring’s size as well as the terminal aromatic substituent’s nature (). Within the hexahydrospiro-indeno[1,2-b]quinoxaline-11,3′-pyrrolizine derivatives 4a-h, the furan-2-yl substituent in 4a conferred the optimum cytotoxic profile to the scaffold regarding potency and selectivity. Replacing the furan-2-yl group by a thiophen-2-yl in 4g led to 2 folds drop in the scaffold’s cytotoxic activity and critical decrease in selectivity towards A549 cells over Wi-38 cells. Isosteric replacement of thiophene by unsubstituted phenyl group in 4h didn’t notably affect the cytotoxic character of the scaffold. Bromination of the terminal phenyl group in para position led to supplemental increase in potency and selectivity of the respective derivative 4d compared to the unsubstituted derivative 4h. On the other hand, the p-fluorinated derivative 4c was slightly less active and selective than the unsubstituted analog 4h. Shifting the fluoro substituent to the meta-position in 4e decreased the anticancer potency but enhanced the selectivity index of the scaffold. Improved selectivity was accomplished by chlorination of the aromatic ring in ortho and para positions (compound 4b), whereas p-methoxy substitution led to both higher potency and selectivity in 4f. Seemingly, the decahydrospiro indeno[1,2-b]quinoxaline-11,3′-pyrrolizine derivatives 6a-h showed relatively better anticancer activities than their hexahydrospiro analogs. Herein, the chlorinated derivative 6b exhibited the highest cytotoxic activity among all the screened spiro compounds with promising selectivity. Replacing the dichlorophenyl group by thiophen-2-yl, p-bromophenyl or furan-2-yl in 6 g, 6d and 6a, respectively, slightly decreased the observed potency, but dramatically abolished the compounds selectivity. Further diversification of the halogen type and position didn’t improve the scaffold activity as observed in case of the p- and m- fluoro derivatives 6c and 6e. The p-methoxyphenyl derivative 6f was more active and selective than the latter derivatives, whereas the unsubstituted phenyl derivative 6h was the least active and selective derivative among the series.
Conclusion
CDK2 inhibition continues to appeal as a strategy to exploit in developing efficient anticancer agents. In the current study, we outline hybridisation and scaffold hopping strategies for the rational design of new spiroindeno[1,2-b]quinoxaline-based CDK2 inhibitors for halting lung cancer. A cost‐effective one-pot multicomponent [3 + 2] cycloaddition reaction was employed to afford the designed library. The reaction mechanism was studied by MEDT and the scaffold absolute configuration was assigned by single crystal x-ray diffraction analysis. Cytotoxicity screening of the series against A549 cells and normal lung fibroblasts Wi-38 revealed that 6b was the most promising derivative (IC50 = 54 nM and SI = 6.64). Docking simulations and molecular dynamic studies suggested that 6b resided well and was stabilised into the cavity of CDK2 by strong hydrophobic interactions and pi-pi stacking with several key amino acid residues. Enzymatic evaluation revealed that 6b (IC50 = 177 nM) was nearly equipotent to roscovitine (IC50 = 141 nM) against CDK2. Collectively, we attempt to provide a quick outlook on introducing hybrid CDK2 inhibitors as new avenues for lung cancer therapy.
Materials and methods
Chemistry
General information
“All chemicals were purchased from Aldrich, Sigma-Aldrich and Fluka, which were used without further purification unless otherwise stated. All melting points were measured using a Gallenkamp melting point apparatus in open glass capillaries and were uncorrected. Crude products were purified by column chromatography on silica gel of 100–200 mesh. IR spectra were measured as KBr pellets using a Nicolet 6700 FT-IR spectrophotometer. The NMR spectra were recorded using a Varian Mercury Jeol-400 NMR spectrometer. 1H NMR (400, 500 or 700 MHz) and 13C NMR (101, 126 or 176 MHz) spectroscopy were performed in deuterated dimethylsulfoxide (DMSO-d6). Chemical shifts (δ) are reported in terms of ppm and coupling constants J are given in Hz. Elemental analysis was carried out using an Elmer 2400 Elemental Analyzer in CHN mode”.
General procedure for the synthesis of spiro compounds (4a-h, 6a-h)
A mixture of the enone derivative 1a-h (0.5 mmol), indeno[1,2-b] quinoxalin-11-one 2 (0.5 mmol, 116 mg) and L-proline (0.5 mmol, 57.5 mg) or octahydroindole-2-carboxylic acid (0.5 mmol, 84.5 mg) in methanol (20 ml) was stirred at 60–65 °C using an oil bath for 3 h to give 4a-h and 6a-h, respectively. After completion of the reaction evident by TLC, the solvent was then removed under vacuum. The crude compound was purified by column chromatography on silica gel (petroleum ether/ethyl acetate (4:1)) to afford the spiro compound as solid in pure form.
(1H-Benzo[d]imidazol-2-yl)((1'S,2'S,7a’R,11R)-1'-(furan-2-yl)-1',2',5',6',7',7a’-hexahydrospiro[indeno[1,2-b]quinoxaline-11,3'-pyrrolizin]-2'-yl)methanone 4a
Yield (%): 78; Yellow solid material; m.p.: 178–180 °C; Molecular Formula: C33H25N5O2; [M+] m/z: 523; (KBr, Cm−1): 3361 (NH), 1678(C = O), 1619(C = N);= +3.82 (c = 0.05, MeOH); 1H-NMR (500 MHz, DMSO-d6) δ 12.76 (s, 1H, NH), 8.31 (dd, J = 8.1, 1.7 Hz, 1H, ArH), 7.95 (dd, J = 8.1, 1.7 Hz, 1H, ArH), 7.83 (t, J = 10.0 Hz, 2H, ArH), 7.62 (dd, J = 7.3, 1.4 Hz, 2H, ArH), 7.53 (d, J = 2.7 Hz, 1H, ArH), 7.49 (td, J = 7.5, 1.3 Hz, 1H, ArH), 7.37 − 7.31 (m, 1H, ArH), 7.17 (d, J = 8.1 Hz, 1H, ArH), 7.09 (td, J = 8.2, 1.3 Hz, 1H, ArH), 6.87 (td, J = 8.2, 1.3 Hz, 1H, ArH), 6.34 (dd, J = 3.3, 1.7 Hz, 1H, ArH), 6.27 (d, J = 3.2 Hz, 1H, ArH), 6.16 (d, J = 8.2 Hz, 1H, ArH), 5.53 (d, J = 11.6 Hz, 1H, COCH), 4.30 − 4.17 (m, 2H), 2.58 (dt, J = 9.1, 7.1 Hz, 1H), 2.30 − 2.23 (m, 1H), 2.13 − 2.06 (m, 1H), 2.02 − 1.89 (m, 2H), 1.88 − 1.78 (m, 1H); 13C-NMR (126 MHz, DMSO-d6) δ 189.32, 165.84, 154.02, 152.92, 147.26, 143.57, 142.70, 142.64, 142.61, 142.14, 137.38, 134.51, 131.45, 130.23, 129.98, 129.89, 129.53, 129.25, 128.35, 126.18, 123.23, 122.18, 120.71, 113.03, 111.04, 106.60, 74.57, 69.70, 63.75, 47.27, 44.95, 30.89, 28.16; Anal. for C33H25N5O2; calcd: C, 75.70; H, 4.81; N, 13.38 Found: C, 74.89; H, 5.03; N, 13.04.
(1H-Benzo[d]imidazol-2-yl)((1'R,2'S,7a’R,11R)-1'-(2,4-dichlorophenyl)-1',2',5',6',7',7a’-hexahydrospiro[indeno[1,2-b]quinoxaline-11,3'-pyrrolizin]-2'-yl)methanone 4b
Yield (%): 85; Pale yellow solid material; m.p.: 205–207 °C; Molecular Formula: C35H25Cl2N5O; [M+] m/z: 601; (KBr, Cm−1): 3364 (NH), 1677(C = O), 1619 (C = N); = +3.82 (c = 0.05, MeOH);1H-NMR (500 MHz, DMSO-d6) δ 12.74 (s, 1H, NH), 8.36 (dd, J = 8.0, 1.8 Hz, 1H, ArH), 8.01 (dd, J = 8.1, 1.7 Hz, 1H, ArH), 7.91 − 7.79 (m, 3H, ArH), 7.66 (d, J = 7.5 Hz, 1H, ArH), 7.63 − 7.55 (m, 2H, ArH), 7.52 (t, J = 6.8 Hz, 1H, ArH), 7.44 (dd, J = 8.6, 2.3 Hz, 1H, ArH), 7.34 (t, J = 7.5 Hz, 1H, ArH), 7.16 (d, J = 8.1 Hz, 1H, ArH), 7.11 − 7.05 (m, 1H, ArH), 6.88 (t, J = 7.1 Hz, 1H, ArH), 6.25 (d, J = 8.4 Hz, 1H, ArH), 5.62 (d, J = 11.6 Hz, 1H, COCH), 4.75 − 4.63 (m, 1H), 4.19 (dt, J = 9.8, 6.3 Hz, 1H), 2.74 − 2.60 (m, 1H), 2.35 − 2.25 (m, 1H), 2.01 − 1.94 (m, 2H), 1.91 − 1.75 (m, 2H); 13C- NMR (126 MHz, DMSO-d6) δ 189.55, 165.94, 153.01, 147.32, 143.56, 142.75, 142.51, 142.13, 137.66, 137.06, 135.28, 134.47, 132.49, 131.62, 130.41, 130.31, 130.08, 130.02, 129.63, 129.51, 129.33, 128.65, 127.77, 126.19, 123.26, 122.34, 120.78, 113.03, 74.51, 72.90, 66.15, 47.40, 46.80, 30.15, 27.82; Anal. for C35H25Cl2N5O; calcd: C, 69.77; H, 4.18; N, 11.62 Found: C, 68.89; H, 5.01; N, 12.04.
(1H-Benzo[d]imidazol-2-yl)((1'R,2'S,7a’R,11R)-1'-(4-fluorophenyl)-1',2',5',6',7',7a’-hexahydrospiro[indeno[1,2-b]quinoxaline-11,3'-pyrrolizin]-2'-yl)methanone 4c
Yield (%): 89; Yellow solid material; m.p.:188–190 °C; Molecular Formula: C35H26FN5O; [M+] m/z: 551; (KBr, Cm−1): 3369 (NH), 1682(C = O), 1621(C = N);= +7.47 (c = 0.05, MeOH);1H-NMR (400 MHz, DMSO-d6) δ 12.76 (s, 1H, NH), 8.40 (d, J = 8.1 Hz, 1H, ArH), 8.02 (d, J = 8.1 Hz, 1H, ArH), 7.89 (dq, J = 15.4, 7.0 Hz, 2H, ArH), 7.71 (dd, J = 12.5, 7.3 Hz, 2H, ArH), 7.63 − 7.51 (m, 3H, ArH), 7.38 (t, J = 7.3 Hz, 1H, ArH), 7.15 (m, 4H, ArH), 6.91 (t, J = 7.7 Hz, 1H, ArH), 6.26 (d, J = 8.1 Hz, 1H, ArH), 5.57 (d, J = 11.0 Hz, 1H, COCH), 4.32 − 4.24 (m, 1H), 4.20 (d, J = 11.7 Hz, 1H), 2.68 (q, J = 7.3, 6.6 Hz, 1H), 2.36 − 2.28 (m, 1H), 2.01 (dd, J = 11.7, 5.9 Hz, 2H), 1.95 − 1.80 (m, 2H); 13C-NMR (101 MHz, DMSO-d6) δ 189.84, 166.13, 153.01, 147.47, 143.85, 142.76, 142.62, 142.18, 137.51, 136.80, 134.52, 131.48, 130.23, 130.16, 130.09, 129.95, 129.60, 129.32, 128.32, 126.20, 123.26, 122.25, 120.79, 116.13, 115.92, 113.05, 74.65, 72.61, 66.29, 50.80, 47.43, 30.40, 28.11; Anal. for C35H26FN5O; calcd: C, 76.21; H, 4.75; N, 12.70 Found: C, 76.16; H, 4.62; N, 12.79.
(1H-Benzo[d]imidazol-2-yl)((1'R,2'S,7a’R,11R)-1'-(4-bromophenyl)-1',2',5',6',7',7a’-hexahydrospiro[indeno[1,2-b]quinoxaline-11,3'-pyrrolizin]-2'-yl)methanone 4d
Yield (%): 85; Yellow solid material; m.p.: 163–165 °C; Molecular Formula: C35H26BrN5O; [M+] m/z: 611; (KBr, Cm−1): 3364 (NH), 1679(C = O), 1619(C = N); = +2.97 (c = 0.05, MeOH);1H-NMR (700 MHz, DMSO-d6) δ 12.76 (s, 1H, NH), 8.40 (d, J = 8.1 Hz, 1H, ArH), 8.03 (d, J = 8.2 Hz, 1H, ArH), 7.94 − 7.85 (m, 2H, ArH), 7.71 (dd, J = 25.9, 7.6 Hz, 2H, ArH), 7.59 − 7.51 (m, 5H, ArH), 7.39 (t, J = 7.4 Hz, 1H, ArH), 7.20 (d, J = 8.1 Hz, 1H, ArH), 7.13 (t, J = 7.6 Hz, 1H, ArH), 6.92 (t, J = 7.7 Hz, 1H, ArH), 6.26 (d, J = 8.3 Hz, 1H, ArH), 5.58 (d, J = 11.8 Hz, 1H, COCH), 4.26 (dt, J = 9.9, 6.5 Hz, 1H), 4.18 (t, J = 10.8 Hz, 1H), 2.90 (s, 1H), 2.74 (s, 1H), 2.69 (q, J = 7.5, 7.1 Hz, 1H), 2.06 − 1.99 (m, 2H), 1.93 (dd, J = 13.5, 6.9 Hz, 1H), 1.88 − 1.82 (m, 1H); 13C- NMR (176 MHz, DMSO-d6) δ 189.62, 165.98, 162.78, 152.91, 147.30, 143.66, 142.65, 142.52, 142.06, 139.98, 137.40, 134.42, 132.06, 131.38, 130.53, 130.15, 129.99, 129.85, 129.50, 129.22, 128.20, 126.10, 123.16, 122.14, 120.67, 120.37, 112.96, 74.56, 72.42, 65.92, 55.40, 50.87, 47.34, 36.25, 30.26, 28.01; Anal. for C35H26BrN5O; calcd: C, 68.63; H, 4.28; N, 11.43 Found: C, 67.75; H, 4.35; N, 12.04.
(1H-Benzo[d]imidazol-2-yl)((1'R,2'S,7a’R,11R)-1'-(3-fluorophenyl)-1',2',5',6',7',7a’-hexahydrospiro[indeno[1,2-b]quinoxaline-11,3'-pyrrolizin]-2'-yl)methanone 4e
Yield (%): 88; Yellow solid material; m.p.: 200–202 °C; Molecular Formula: C35H26FN5O; [M+] m/z: 551; (KBr, Cm−1): 3362(NH), 1681(C = O), 1621(C = N);= +9.35 (c = 0.05, MeOH);1H-NMR (400 MHz, DMSO-d6) δ 12.77 (s, 1H, NH), 8.39 (d, J = 8.1 Hz, 1H, ArH), 8.03 (d, J = 8.1 Hz, 1H, ArH), 7.89 (dq, J = 15.4, 7.0 Hz, 2H, ArH), 7.71 (dd, J = 11.0, 7.3 Hz, 2H, ArH), 7.55 (t, J = 7.7 Hz, 1H, ArH), 7.45 − 7.32 (m, 4H, ArH), 7.20 (d, J = 8.1 Hz, 1H, ArH), 7.15 − 7.02 (m, 2H, ArH), 6.91 (t, J = 7.7 Hz, 1H, ArH), 6.28 (d, J = 8.8 Hz, 1H, ArH), 5.58 (d, J = 11.7 Hz, 1H, COCH), 4.38 − 4.27 (m, 1H), 4.26 − 4.15 (m, 1H), 2.69 (q, J = 8.4 Hz, 1H), 2.33 (h, J = 3.7 Hz, 1H), 2.08 − 1.78 (m, 4H); 13C-NMR (101 MHz, DMSO-d6) δ 189.79, 166.10, 164.08, 161.66, 153.04, 147.44, 143.77, 142.76, 142.60, 142.18, 137.54, 134.53, 131.73, 130.23, 123.84, 117.52, 74.66, 72.98, 67.06, 54.59, 50.77, 46.26, 30.29, 25.61; Anal. for C35H26FN5O; calcd: C, 76.21; H, 4.75; N, 12.70 Found: C, 75.92; H, 4.13; N, 12.01.
(1H-Benzo[d]imidazol-2-yl)((1'R2'S7a’R11R)-1'-(4-methoxyphenyl)-1',2',5',6',7',7a’-hexahydrospiro[indeno[1,2-b]quinoxaline-11,3'-pyrrolizin]-2'-yl)methanone 4f
Yield (%): 74; Yellow solid material; m.p.: 158–160 °C; Molecular Formula: C36H29N5O2; [M+] m/z: 563; (KBr, Cm−1): 3360 (NH), 1678(C = O), 1618(C = N);= +2.26 (c = 0.05, MeOH); 1H-NMR (500 MHz, DMSO-d6) δ 12.69 (s, 1H, NH), 8.35 (dd, J = 8.2, 1.5 Hz, 1H, ArH), 7.97 (dd, J = 8.2, 1.6 Hz, 1H, ArH), 7.87 (ddd, J = 8.3, 6.9, 1.6 Hz, 1H, ArH), 7.81 (ddd, J = 8.3, 6.9, 1.5 Hz, 1H, ArH), 7.69 − 7.63 (m, 2H, ArH), 7.50 (td, J = 7.5, 1.3 Hz, 1H, ArH), 7.42 (d, J = 8.8 Hz, 2H, ArH), 7.34 (td, J = 7.4, 1.0 Hz, 1H, ArH), 7.15 (dt, J = 8.2, 1.1 Hz, 1H, ArH), 7.07 (ddd, J = 8.1, 6.9, 1.2 Hz, 1H, ArH), 6.87 (dd, J = 8.7, 2.5 Hz, 3H, ArH), 6.23 (d, J = 8.3 Hz, 1H, ArH), 5.52 (d, J = 11.9 Hz, 1H, COCH), 4.26 − 4.18 (m, 1H), 4.06 (dd, J = 12.0, 9.7 Hz, 1H), 3.65 (s, 3H), 2.69 − 2.59 (m, 1H), 2.32 − 2.25 (m, 1H), 1.97 (dd, J = 14.8, 11.2 Hz, 2H), 1.88 − 1.74 (m, 2H); 13C-NMR (126 MHz, DMSO-d6) δ 189.95, 166.15, 158.68, 152.94, 147.52, 143.93, 142.70, 142.59, 142.16, 137.41, 134.47, 132.40, 131.42, 130.12, 130.06, 129.87, 129.53, 129.29, 129.26, 128.32, 126.11, 123.19, 122.18, 120.74, 114.65, 113.00, 74.66, 72.56, 66.27, 55.52, 50.88, 47.39, 30.51, 28.11; Anal. for C36H29N5O2; calcd: C, 76.71; H, 5.19; N, 12.43 Found: C, 76.12; H, 5.01; N, 12.78.
(1H-Benzo[d]imidazol-2-yl)((1'S,2'S,7a’R,11R)-1'-(thiophen-2-yl)-1',2',5',6',7',7a’-hexahydrospiro[indeno[1,2-b]quinoxaline-11,3'-pyrrolizin]-2'-yl)methanone 4g
Yield (%): 87; White solid material; m.p.: 176–178 °C; Molecular Formula: C33H25N5OS; [M+] m/z: 539; (KBr, Cm−1): 3359 (NH), 1678(C = O), 1617(C = N);= +4.85 (c = 0.05, MeOH); 1H-NMR (400 MHz, DMSO-d6) δ 12.86 (s, 1H, NH), 8.37 (d, J = 8.1 Hz, 1H, ArH), 8.01 (d, J = 8.1 Hz, 1H, ArH), 7.87 (dq, J = 15.4, 7.0 Hz, 2H, ArH), 7.69 (d, J = 8.8 Hz, 2H, ArH), 7.54 (t, J = 7.7 Hz, 1H, ArH), 7.38 (q, J = 5.5, 3.7 Hz, 2H, ArH), 7.22 (d, J = 8.8 Hz, 1H, ArH), 7.12 (dd, J = 13.9, 5.9 Hz, 2H, ArH), 7.01 − 6.95 (m, 1H, ArH), 6.91 (t, J = 7.7 Hz, 1H, ArH), 6.26 (d, J = 8.1 Hz, 1H, ArH), 5.52 (d, J = 11.0 Hz, 1H, COCH), 4.44 (d, J = 11.7 Hz, 1H), 4.40 − 4.32 (m, 1H), 2.68 (q, J = 8.1 Hz, 1H), 2.37 − 2.27 (m, 1H), 2.10 (dd, J = 11.0, 6.6 Hz, 1H), 2.04 − 1.82 (m, 3H); 13C-NMR (101 MHz, DMSO-d6) δ 189.61, 165.88, 152.96, 147.43, 143.66, 143.59, 142.77, 142.63, 142.23, 137.45, 134.59, 131.49, 130.28, 130.05, 129.61, 129.32, 128.42, 127.70, 126.28, 125.43, 124.84, 123.32, 122.26, 120.82, 113.10, 74.80, 72.51, 67.21, 47.46, 46.78, 30.58, 28.09; Anal. for C33H25N5OS; calcd: C, 73.45; H, 4.67; N, 12.98 Found: C, 73.36; H, 4.61; N, 12.91.
(1H-Benzo[d]imidazol-2-yl)((1'R,2'S,7a’R,11R)-1'-phenyl-1',2',5',6',7',7a’-hexahydrospiro[indeno[1,2-b]quinoxaline-11,3'-pyrrolizin]-2'-yl)methanone 4h
Yield (%): 92; Yellow solid material; m.p.: 192–194 °C; Molecular Formula: C35H27N5O; [M+] m/z: 533; (KBr, Cm−1): 3360 (NH), 1675(C = O), 1619(C = N);= +8.53 (c = 0.05, MeOH); 1H-NMR (400 MHz, DMSO-d6) δ 12.75 (s, 1H, NH), 8.40 (d, J = 8.1 Hz, 1H, ArH), 8.02 (d, J = 8.1 Hz, 1H, ArH), 7.89 (dt, J = 22.7, 7.0 Hz, 2H, ArH), 7.71 (dd, J = 12.5, 8.1 Hz, 2H, ArH), 7.59 − 7.51 (m, 3H, ArH), 7.37 (dt, J = 11.0, 7.3 Hz, 3H, ArH), 7.21 (dd, J = 15.4, 8.1 Hz, 2H, ArH), 7.12 (t, J = 7.7 Hz, 1H, ArH), 6.91 (t, J = 7.7 Hz, 1H, ArH), 6.26 (d, J = 8.1 Hz, 1H, ArH), 5.63 (d, J = 12.5 Hz, 1H, COCH), 4.35 − 4.25 (m, 1H), 4.22 − 4.12 (m, 1H), 2.69 (q, J = 8.1 Hz, 1H), 2.33 (m, 1H), 2.07 − 1.97 (m, 2H), 1.88 (m, 2H); 13C-NMR (101 MHz, DMSO-d6) δ 189.91, 166.19, 153.02, 147.51, 143.92, 142.76, 142.64, 142.20, 140.70, 137.50, 134.53, 131.49, 130.20, 130.11, 129.94, 129.59, 129.32, 129.27, 128.37, 127.40, 126.18, 123.25, 122.24, 120.79, 113.05, 74.71, 72.68, 66.18, 51.60, 47.42, 30.58, 28.16; Anal. for C35H27N5O; calcd: C, 78.78; H, 5.10; N, 13.12 Found: C, 78.72; H, 5.15; N, 13.08.
(1H-Benzo[d]imidazol-2-yl)((1'S,2'S,11R)-1'-(furan-2-yl)-1',2',4a’,5',6',7',8',8a’,9',9a’-decahydrospiro[indeno[1,2-b]quinoxaline-11,3'-pyrrolo[1,2-a]indol]-2'-yl)methanone 6a
Yield (%): 74; Orange solid material; m.p.: 175–177 °C; Molecular Formula: C37H25N5O2; [M+] m/z: 571; (KBr, Cm−1): 3432 (NH), 1677(C = O), 1620(C = N);= +56.44 (c = 0.05, MeOH); 1H-NMR (400 MHz, DMSO-d6) δ 12.74 (s, 1H, NH), 8.42 (d, J = 8.1 Hz, 1H, ArH), 7.96 (d, J = 8.1 Hz, 1H, ArH), 7.85 (d, J = 8.1 Hz, 2H, ArH), 7.76 (d, J = 8.1 Hz, 1H, ArH), 7.65 (d, J = 7.3 Hz, 1H, ArH), 7.60 − 7.53 (m, 2H, ArH), 7.40 (t, J = 7.7 Hz, 1H, ArH), 7.19 (d, J = 8.1 Hz, 1H, ArH), 7.12 (t, J = 7.7 Hz, 1H, ArH), 6.89 (t, J = 8.1 Hz, 1H, ArH), 6.42 − 6.33 (m, 1H, ArH), 6.27 (d, J = 3.7 Hz, 1H, ArH), 6.06 (d, J = 8.8 Hz, 1H, ArH), 5.59 (d, J = 11.7 Hz, 1H, COCH), 4.49 (q, J = 8.8, 6.6 Hz, 1H), 4.36 − 4.24 (m, 1H), 2.14 (dd, J = 29.7, 14.3 Hz, 2H), 1.90 − 1.81 (m, 1H), 1.53 (d, J = 6.6 Hz, 1H), 1.44 (dd, J = 13.2, 4.4 Hz, 2H), 1.39 (s, 1H), 1.35 (d, J = 7.3 Hz, 1H), 1.23 (d, J = 7.3 Hz, 1H), 1.01 (d, J = 6.6 Hz, 2H), 0.78 (d, J = 4.4 Hz, 1H), 0.68 (d, J = 12.5 Hz, 1H); 13C-NMR (101 MHz, DMSO-d6) δ 188.82, 153.88, 152.72, 147.19, 142.58, 142.15, 137.26, 134.52, 130.10, 129.44, 129.26, 126.20, 123.20, 122.08, 120.71, 111.04, 106.57, 73.65, 71.49, 70.18, 69.34, 64.56, 51.17, 46.75, 33.90, 31.20, 29.86, 29.65, 27.01, 25.53, 18.26, 17.72, 15.29; Anal. for C37H31N5O2; Calcd: C, 76.93; H, 5.41; N, 12.12 Found: C, 76.99; H, 5.36; N, 12.20.
(1H-Benzo[d]imidazol-2-yl)((1'R,2'S,11R)-1'-(2,4-dichlorophenyl)-1',2',4a’,5',6',7',8',8a’,9',9a’-decahydrospiro[indeno[1,2-b]quinoxaline-11,3'-pyrrolo[1,2-a]indol]-2'-yl)methanone 6b
Yield (%): 84; Yellow solid material; m.p.: 161–163 °C; Molecular Formula: C39H25Cl2N5O; [M+] m/z: 649; (KBr, Cm−1): 3430 (NH), 1672(C = O), 1619(C = N); = +62.15 (c = 0.05, MeOH);1H-NMR (500 MHz, DMSO-d6) δ 12.60 (s, 1H, NH), 8.41 (d, J = 8.4 Hz, 1H, ArH), 7.96 (d, J = 9.2 Hz, 1H, ArH), 7.89 − 7.80 (m, 2H, ArH), 7.68 − 7.55 (m, 5H, ArH), 7.41 − 7.33 (m, 2H, ArH), 7.15 − 7.04 (m, 2H, ArH), 6.85 (t, J = 7.6 Hz, 1H, ArH), 6.03 (d, J = 8.4 Hz, 1H, ArH), 5.70 (d, J = 12.2 Hz, 1H, COCH), 4.70 (dd, J = 12.2, 9.9 Hz, 1H), 4.29 (td, J = 9.6, 6.1 Hz, 1H), 3.26 (d, J = 7.6 Hz, 1H), 2.18 − 2.09 (m, 1H), 2.08 − 1.99 (m, 1H), 1.73 − 1.63 (m, 1H), 1.45 − 1.32 (m, 3H), 1.15 − 1.02 (m, 1H), 0.80 (d, J = 19.9 Hz, 1H), 0.70 − 0.57 (m, 2H), 0.22 (d, J = 10.7 Hz, 1H); 13C-NMR (126 MHz, DMSO-d6) δ 188.77, 166.57, 152.79, 147.03, 143.00, 142.74, 142.04, 137.48, 136.53, 135.40, 134.38, 132.39, 131.30, 130.23, 130.11, 129.82, 129.73, 129.51, 129.43, 129.27, 128.46, 128.36, 126.19, 123.18, 122.22, 120.67, 112.98, 73.54, 71.76, 66.57, 57.15, 47.69, 41.95, 37.39, 28.35, 27.61, 24.67, 19.08; Anal. for C39H31Cl2N5O; Calcd: C, 71.34; H, 4.76; N, 10.67 Found: C, 71.29; H, 4.82; N, 10.61.
(1H-Benzo[d]imidazol-2-yl)((1'R,2'S,11R)-1'-(4-fluorophenyl)-1',2',4a’,5',6',7',8',8a’,9',9a’-decahydrospiro[indeno[1,2-b]quinoxaline-11,3'-pyrrolo[1,2-a]indol]-2'-yl)methanone 6c
Yield (%): 93; Yellow solid material; m.p.: 187–189 °C; Molecular Formula: C39H32FN5O; [M+] m/z: 605; (KBr, Cm−1): 3434 (NH), 1678(C = O), 1620(C = N); = +90.21 (c = 0.05, MeOH);1H-NMR (400 MHz, DMSO-d6) δ 12.68 (s, 1H, NH), 8.46 (d, J = 8.1 Hz, 1H, ArH), 7.98 (d, J = 8.1 Hz, 1H, ArH), 7.87 (m, 3H, ArH), 7.68 (d, J = 7.3 Hz, 1H, ArH), 7.56 (m, 3H, ArH), 7.41 (t, J = 7.3 Hz, 1H, ArH), 7.15 (m, 4H, ArH), 6.93 − 6.85 (m, 1H, ArH), 6.10 (d, J = 8.8 Hz, 1H, ArH), 5.66 (d, J = 12.5 Hz, 1H, COCH), 4.39 (q, J = 8.8 Hz, 1H), 4.29 − 4.19 (m, 1H), 2.20 − 2.03 (m, 2H), 1.68 (dd, J = 11.7, 5.9 Hz, 1H), 1.48 − 1.37 (m, 3H), 1.25 − 1.15 (m, 1H), 1.15 − 1.07 (m, 1H), 0.92 − 0.81 (m, 1H), 0.65 (t, J = 11.7 Hz, 2H), 0.25 (d, J = 11.0 Hz, 1H); 13C-NMR (101 MHz, DMSO-d6) δ 189.22, 166.92, 152.77, 147.29, 143.08, 142.75, 142.32, 142.16, 137.33, 136.31, 134.49, 131.10, 130.12, 130.01, 129.93, 129.69, 129.42, 129.28, 129.08, 126.18, 123.19, 122.10, 120.75, 116.02, 115.81, 113.02, 73.76, 71.55, 66.34, 57.20, 51.67, 42.06, 37.46, 30.98, 28.45, 27.86, 24.83, 19.15; Anal. for C39H32FN5O; calcd: C, 77.33; H, 5.33; N, 11.56 Found: C, 77.27; H, 5.39; N, 11.51.
(1H-Benzo[d]imidazol-2-yl)((1'R,2'S,11R)-1'-(4-bromophenyl)-1',2',4a’,5',6',7',8',8a’,9',9a’-decahydrospiro[indeno[1,2-b]quinoxaline-11,3'-pyrrolo[1,2-a]indol]-2'-yl)methanone 6d
Yield (%): 83; Yellow solid material; m.p.: 160–162 °C; Molecular Formula: C39H26BrN5O; [M+] m/z: 659; (KBr, Cm−1): 3437 (NH), 1675(C = O), 1621(C = N); = +75.91 (c = 0.05, MeOH);1H-NMR (700 MHz, DMSO-d6) δ 12.66 (s, 1H, NH), 8.45 (dd, J = 8.2, 1.5 Hz, 1H, ArH), 7.98 (dd, J = 8.1, 1.5 Hz, 1H, ArH), 7.91 (ddd, J = 8.3, 6.9, 1.6 Hz, 2H, ArH), 7.86 (t, J = 6.8 Hz, 2H, ArH), 7.67 (d, J = 7.5 Hz, 1H, ArH), 7.59 (td, J = 7.5, 1.2 Hz, 1H, ArH), 7.54 (d, J = 8.6 Hz, 2H, ArH), 7.47 (d, J = 8.6 Hz, 2H, ArH), 7.42 (td, J = 7.4, 1.0 Hz, 1H, ArH), 7.20 − 7.17 (m, 1H, ArH), 7.12 (ddd, J = 8.0, 6.9, 1.1 Hz, 1H, ArH), 6.90 (ddd, J = 8.2, 7.0, 1.1 Hz, 1H, ArH), 5.64 (d, J = 12.4 Hz, 1H, COCH), 4.37 (td, J = 9.3, 5.9 Hz, 1H), 4.31 − 4.18 (m, 1H), 2.22 − 2.09 (m, 2H), 1.71 (dd, J = 11.8, 5.9 Hz, 1H), 1.53 − 1.39 (m, 3H), 1.18 (t, J = 7.1 Hz, 1H), 1.09 (tt, J = 13.0, 2.8 Hz, 1H), 0.91 (dt, J = 13.3, 3.2 Hz, 1H), 0.73 − 0.63 (m, 2H), 0.29 − 0.20 (m, 1H); 13C- NMR (176 MHz, DMSO-d6) δ 189.00, 166.74, 152.64, 147.10, 142.94, 142.61, 142.13, 142.01, 139.52, 137.20, 134.36, 131.97, 131.01, 130.32, 130.06, 130.03, 129.60, 129.33, 129.16, 128.97, 126.09, 124.72, 123.09, 122.79, 121.98, 120.59, 120.29, 112.91, 73.62, 71.39, 65.96, 60.23, 57.10, 51.64, 41.93, 37.25, 28.32, 27.77, 24.71, 21.24, 19.01, 14.56; Anal. for C39H32BrN5O; Calcd: C, 70.27; H, 4.84; N, 10.51 Found: C, 70.21; H, 4.78; N, 10.45.
(1H-Benzo[d]imidazol-2-yl)((1'R,2'S,11R)-1'-(3-fluorophenyl)-1',2',4a’,5',6',7',8',8a’,9',9a’-decahydrospiro[indeno[1,2-b]quinoxaline-11,3'-pyrrolo[1,2-a]indol]-2'-yl)methanone 6e
Yield (%): 65; Orange solid material; m.p.: 160–162 °C; Molecular Formula: C39H26FN5O; [M+] m/z: 599; (KBr, Cm−1): 3434 (NH), 1681(C = O), 1617(C = N); = +79.12 (c = 0.05, MeOH);1H-NMR (400 MHz, DMSO-d6) δ 12.69 (s, 1H, NH), 8.45 (d, J = 8.1 Hz, 1H, ArH), 7.97 (d, J = 8.1 Hz, 1H, ArH), 7.94 − 7.77 (m, 4H, ArH), 7.67 (d, J = 7.3 Hz, 1H, ArH), 7.59 (t, J = 7.7 Hz, 2H, ArH), 7.45 − 7.26 (m, 5H, ArH), 7.05 (t, J = 9.2 Hz, 2H, ArH), 5.64 (d, J = 12.5 Hz, 1H, COCH), 4.41 (q, J = 7.7, 6.6 Hz, 1H), 4.34 − 4.20 (m, 1H), 2.15 (d, J = 8.8 Hz, 2H), 1.73 − 1.65 (m, 1H), 1.54 − 1.38 (m, 4H), 1.10 − 1.03 (m, 1H), 0.89 (d, J = 11.7 Hz, 1H), 0.68 (d, J = 9.5 Hz, 2H), 0.25 (d, J = 11.7 Hz, 1H); 13C-NMR (101 MHz, DMSO-d6) δ 189.11, 166.84, 164.04, 161.61, 152.77, 147.23, 143.25, 143.18, 143.04, 142.72, 142.22, 137.32, 131.13, 130.13, 129.33, 124.55, 122.66, 114.20, 73.75, 71.37, 66.17, 57.21, 52.03, 42.03, 36.82, 28.42, 27.85, 24.81, 19.13; Anal. for C39H32FN5O; calcd: C, 77.33; H, 5.33; N, 11.56 Found: C, 77.38; H, 5.25; N, 11.48.
(1H-Benzo[d]imidazol-2-yl)((1'R,2'S,11R)-1'-(4-methoxyphenyl)-1',2',4a’,5',6',7',8',8a’,9',9a’-decahydrospiro[indeno[1,2-b]quinoxaline-11,3'-pyrrolo[1,2-a]indol]-2'-yl)methanone 6f
Yield (%): 73; Orange solid material; m.p.: 161–163 °C; Molecular Formula: C40H29N5O2; [M+] m/z: 611; (KBr, Cm−1): 3432 (NH), 1674(C = O), 1620(C = N); = +85.67 (c = 0.05, MeOH);1H-NMR (400 MHz, DMSO-d6) δ 12.64 (s, 1H, NH), 8.46 (d, J = 8.1 Hz, 1H, ArH), 8.00 − 7.78 (m, 4H, ArH), 7.67 (d, J = 7.3 Hz, 1H, ArH), 7.59 (t, J = 7.7 Hz, 1H, ArH), 7.41 (t, J = 8.1 Hz, 3H, ArH), 7.18 (d, J = 8.1 Hz, 1H, ArH), 7.11 (t, J = 7.7 Hz, 1H, ArH), 6.90 (d, J = 8.8 Hz, 3H, ArH), 6.09 (d, J = 8.1 Hz, 1H, ArH), 5.63 (d, J = 12.5 Hz, 1H, COCH), 4.44 − 4.30 (m, 1H), 4.23 − 4.09 (m, 1H), 3.69 (s, 3H), 2.23 − 2.03 (m, 2H), 1.70 (dd, J = 11.7, 5.9 Hz, 1H), 1.53 − 1.35 (m, 3H), 1.23 − 1.04 (m, 2H), 0.89 (q, J = 16.9, 14.3 Hz, 1H), 0.68 (d, J = 8.8 Hz, 2H), 0.25 (d, J = 10.3 Hz, 1H); 13C-NMR (101 MHz, DMSO-d6) δ 189.34, 166.96, 158.67, 152.71, 147.38, 143.07, 142.71, 142.44, 142.16, 137.25, 134.47, 131.98, 130.11, 129.12, 126.15, 123.15, 122.47, 114.60, 113.00, 73.81, 71.56, 66.31, 57.18, 55.53, 51.75, 42.09, 27.47, 24.83, 19.16; Anal. for C40H35N5O2; calcd: C, 77.77; H, 5.71; N, 11.34 Found: C, 77.84; H, 5.63; N, 11.41.
(1H-Benzo[d]imidazol-2-yl)((1'S,2'S,11R)-1'-(thiophen-2-yl)-1',2',4a’,5',6',7',8',8a’,9',9a’-decahydrospiro[indeno[1,2-b]quinoxaline-11,3'-pyrrolo[1,2-a]indol]-2'-yl)methanone 6g
Yield (%): 95; Yellow solid material; m.p.: 182–184 °C; Molecular Formula: C37H31N5OS; [M+] m/z: 593; (KBr, Cm−1): 3433 (NH), 1674(C = O), 1621(C = N);= +59.74 (c = 0.05, MeOH); 1H-NMR (400 MHz, DMSO-d6) δ 12.81 (s, 1H, NH), 8.45 (d, J = 8.8 Hz, 1H, ArH), 7.99 (d, J = 8.1 Hz, 1H, ArH), 7.88 (dd, J = 15.8, 7.7 Hz, 2H, ArH), 7.80 (m, 1H, ArH), 7.70 (d, J = 7.3 Hz, 1H, ArH), 7.58 (t, J = 7.3 Hz, 1H, ArH), 7.41 (t, J = 7.3 Hz, 1H, ArH), 7.35 (m, J = 5.1 Hz, 1H, ArH), 7.23 (d, J = 8.1 Hz, 1H, ArH), 7.12 (t, J = 7.7 Hz, 1H, ArH), 7.07 (d, J = 4.4 Hz, 1H, ArH), 6.95 (t, J = 4.4 Hz, 1H, ArH), 6.89 (t, J = 7.7 Hz, 1H, ArH), 6.13 (d, J = 8.1 Hz, 1H, ArH), 5.58 (d, J = 11.0 Hz, 1H, COCH), 4.53 (t, J = 8.4 Hz, 2H), 2.17 − 2.05 (m, 2H), 1.83 (dd, J = 11.4, 5.5 Hz, 1H), 1.43 (t, J = 12.8 Hz, 3H), 1.18 − 1.03 (m, 1H), 0.90 − 0.76 (m, 1H), 0.66 (d, J = 12.5 Hz, 2H), 0.26 (d, J = 11.7 Hz, 1H); 13C-NMR (101 MHz, DMSO-d6) δ 189.05, 166.64, 152.71, 147.30, 143.25, 143.07, 142.77, 142.24, 142.10, 137.30, 134.58, 131.11, 130.14, 129.73, 129.45, 129.30, 127.64, 126.25, 125.31, 124.66, 123.24, 122.13, 120.80, 113.08, 73.98, 71.49, 67.63, 57.22, 55.48, 47.67, 42.04, 37.61, 28.41, 27.92, 24.85, 19.16; Anal. for C37H31N5OS; Calcd: C, 74.85; H, 5.26; N, 11.80 Found: C, 74.79; H, 5.32; N, 11.73.
(1H-Benzo[d]imidazol-2-yl)((1'R,2'S,11R)-1'-phenyl-1',2',4a’,5',6',7',8',8a’,9',9a’-decahydrospiro[indeno[1,2-b]quinoxaline-11,3'-pyrrolo[1,2-a]indol]-2'-yl)methanone 6h
Yield (%): 84; Yellow solid material; m.p.: 176–178 °C; Molecular Formula: C39H33N5O; [M+] m/z: 587; (KBr, Cm−1): 3428(NH), 1679(C = O), 1617(C = N); = +33.57 (c = 0.05, MeOH);1H-NMR (400 MHz, DMSO-d6) δ 12.68 (s, 1H, NH), 8.47 (d, J = 8.1 Hz, 1H, ArH), 7.99 (d, J = 8.1 Hz, 1H, ArH), 7.95 − 7.80 (m, 3H, ArH), 7.69 (d, J = 7.3 Hz, 1H, ArH), 7.60 (t, J = 7.3 Hz, 1H, ArH), 7.46 (dd, J = 24.9, 7.3 Hz, 3H, ArH), 7.32 (t, J = 7.3 Hz, 2H, ArH), 7.23 − 7.12 (m, 3H, ArH), 7.10 (d, J = 8.1 Hz, 1H, ArH), 6.89 (t, J = 7.7 Hz, 1H, ArH), 6.12 (d, J = 8.1 Hz, 1H, ArH), 5.73 (d, J = 12.5 Hz, 1H, COCH), 4.48 − 4.37 (m, 1H), 4.28 − 4.18 (m, 1H), 3.34 (s, 1H), 2.17 − 2.03 (m, 2H), 1.69 (dd, J = 11.0, 5.9 Hz, 1H), 1.43 (t, J = 11.0 Hz, 3H), 1.13 (dt, J = 16.1, 7.7 Hz, 2H), 0.66 (d, J = 9.5 Hz, 2H), 0.27 (d, J = 11.0 Hz, 1H); 13C-NMR (101 MHz, DMSO-d6) δ 189.31, 167.00, 152.78, 147.35, 143.11, 142.76, 142.40, 142.19, 140.22, 137.33, 134.51, 131.10, 130.15, 130.10, 129.68, 129.41, 129.29, 129.18, 129.11, 128.14, 127.32, 126.16, 123.18, 122.11, 120.76, 113.03, 73.83, 71.64, 66.17, 57.21, 52.50, 42.09, 37.68, 28.48, 27.86, 24.85, 19.16; Anal. for C39H33N5O; calcd: C, 79.70; H, 5.66; N, 11.92 Found: C, 79.65; H, 5.71; N, 12.85.
Biology
MTT assay
A549 cancer cells and normal human lung fibroblasts (Wi-38) were purchased from the National Cancer Institute in Cairo, Egypt, and were cultured in “RPMI-1640 medium L-Glutamine (Lonza Verviers SPRL, Belgium, cat#12-604F), 10% foetal bovine serum (FBS, Sigma-Aldrich, MO, USA) and 1% penicillin-streptomycin (Lonza, Belgium)”. All cells were incubated at 37 °C in 5% carbon dioxide atmosphere (NuAire). In a 96-well plate, cells were plated in triplicate at a density of 5 × 10^4 cells and were treated with the compounds at concentrations of (0.01, 0.1, 1, 10, and 100 µM) on the second day. Cell viability was assessed using MTT solution (Promega, USA)Citation52. The viability was calculated in comparison to the control, and the IC50 values were computedCitation53.
CDK2 inhbition assay
CDK2 luminescence kinase Assay kit (Catalog #79599, Kinase-Glo Plus, Promega, USA) was used to evaluate the inhibitory potency of compound 6b using serial dilutions of 0.01–10 μM against the CDK2 inhbition. The autophosphorylation percentage inhibition by compound 6b was calculated using the following equation: 100-[Control/Treated-Control)] using the curves of percentage inhibition of eight concentrations of each compound, IC50 was calculated using the GraphPad prism7 softwareCitation54,Citation55.
Computational studies
MEDT
The ωB97X-DCitation56 functional, together with the standard 6–311 G(d,p)Citation57 basis set, were used throughout this MEDT study. Solvent effects of methanol were taken into account by full optimisation of the gas phase structures at the same computational level using the polarisable continuum model (PCM)Citation58,Citation59 in the framework of the self-consistent reaction field (SCRF)Citation60–62. The GEDT values were computed by using the equation GEDT(f) = Σqf, were q are the natural chargesCitation63,Citation64 of the atoms belonging to one of the two frameworks (f) at the TS geometries. Global and local CDFT indices were calculated by using the equations given in reference.Citation42 The Gaussian 16 suite of programs was used to perform the calculations.Citation65
Docking and molecular dynamics
To comprehend the binding modes and potential activities of the newly developed spiro-indeno[1,2-b]quinoxaline derivatives, molecular docking was performed against the established target: CDK2 (PDB ID 2A4L)Citation66. The crystal structure of the target protein was retrieved from the Protein Data Bank (www.rcsb.org/pdb). CDK2 protein was protonated, charged and minimised by using MOE 2019.01 suiteCitation67. The chemical structure of the screened compounds and the reference drug roscovitine were constructed using the Builder tool implemented into MOE. The structures were further prepared including atom-type corrections, protonation, minimisation, and charge assignment and saved in its respective 3D conformations. Prior to the molecular docking experiments, a re-docking was performed to assess the efficiency of the software and docking protocol. The re-docking outcomes confirmed the suitability of MOE as an appropriate software for further docking studies of the quinoxaline derivatives. Further, to investigate the dynamic stability of compound 6b in the active site of CDK2, a short production run of 100 ns was performed using AMBER22Citation68. System preparation including minimisation and equilibrium process were done as previously describedCitation69. The CPPTRAJ module integrated in AMBER suite was used for trajectory analysisCitation70.
Supplemental Material
Download PDF (5.3 MB)Disclosure statement
The authors declare that they have no known competing financial interests or personal relationships that could have appeared to influence the work reported in this paper.
Additional information
Funding
References
- Horiuchi D, Huskey NE, Kusdra L, Wohlbold L, Merrick KA, Zhang C, Creasman KJ, Shokat KM, Fisher RP, Goga A. Chemical-genetic analysis of cyclin dependent kinase 2 function reveals an important role in cellular transformation by multiple oncogenic pathways. Proc Natl Acad Sci USA. 2012;109(17):E1019–E1027.
- Tetsu O, McCormick F. Proliferation of cancer cells despite CDK2 inhibition. Cancer Cell. 2003;3(3):233–245.
- Yang L, Fang D, Chen H, Lu Y, Dong Z, Ding HF, Jing Q, Su SB, Huang S. Cyclin-dependent kinase 2 is an ideal target for ovary tumors with elevated cyclin E1 expression. Oncotarget. 2015;6(25):20801–20812.
- Sun H, Yin M, Hao D, Shen Y. Anti-cancer activity of Catechin against A549 Lung Carcinoma cells by induction of cyclin kinase inhibitor p21 and suppression of cyclin E1 and P–AKT. Appl Sci. 2020;10(6):2065.
- Ullah MA, Farzana M, Islam MS, Moni R, Zohora US, Rahman MS. Identification of the prognostic and therapeutic values of cyclin E1 (CCNE1) gene expression in Lung Adenocarcinoma and Lung Squamous Cell Carcinoma: a database mining approach. Heliyon. 2022; 8(9):e10367.
- Barriere C, Santamaria D, Cerqueira A, Galan J, Martin A, Ortega S, Malumbres M, Dubus P, Barbacid M. Mice thrive without Cdk4 and Cdk2. Mol Oncol. 2007;1(1):72–83.
- Beale G, Haagensen EJ, Thomas HD, Wang LZ, Revill CH, Payne SL, Golding BT, Hardcastle IR, Newell DR, Griffin RJ, et al. Combined PI3K and CDK2 inhibition induces cell death and enhances in vivo antitumour activity in colorectal cancer. Br J Cancer. 2016;115(6):682–690.
- Bolin S, Borgenvik A, Persson CU, Sundstrom A, Qi J, Bradner JE, Weiss WA, Cho YJ, Weishaupt H, Swartling FJ. Combined BET Bromodomain and CDK2 inhibition in MYC-driven medulloblastoma. Oncogene. 2018;37(21):2850–2862.
- Stefanikova A, Klacanova K, Pilchova I, Hatok J, Racay P. Cyclin-dependent Kinase 2 inhibitor SU9516 increases sensitivity of colorectal carcinoma cells Caco-2 but not HT29 to BH3 Mimetic ABT-737. Gen Physiol Biophys. 2017;36(5):539–547.
- Whittaker SR, Barlow C, Martin MP, Mancusi C, Wagner S, Self A, Barrie E, Te Poele R, Sharp S, Brown N, et al. Molecular profiling and combinatorial activity of CCT068127: a potent CDK2 and CDK9 inhibitor. Mol Oncol. 2018;12(3):287–304.
- Azimi A, Caramuta S, Seashore-Ludlow B, Bostrom J, Robinson JL, Edfors F, Tuominen R, Kemper K, Krijgsman O, Peeper DS, et al. Targeting CDK2 overcomes melanoma resistance against BRAF and Hsp90 inhibitors. Mol Syst Biol. 2018;14(3):e7858.
- Herrera-Abreu MT, Palafox M, Asghar U, Rivas MA, Cutts RJ, Garcia-Murillas I, Pearson A, Guzman M, Rodriguez O, Grueso J, et al. Early adaptation and acquired resistance to CDK4/6 inhibition in estrogen receptor-positive breast cancer. Cancer Res. 2016;76(8):2301–2313.
- Pernas S, Tolaney SM, Winer EP, Goel S. CDK4/6 inhibition in breast cancer: current practice and future directions. Ther Adv Med Oncol. 2018; 10(10):1758835918786451.
- Schang LM. Advances on Cyclin-dependent Kinases (CDKs) as Novel targets for antiviral drugs. Curr Drug Targets Infect Disord. 2005;5(1):29–37.
- Lee KH, Lee SJ, Lee HJ, Choi GE, Jung YH, Kim DI, Gabr AA, Ryu JM, Han HJ. Amyloid beta1-42 (Abeta1-42) induces the CDK2-mediated phosphorylation of Tau through the activation of the mTORC1 signaling pathway while promoting neuronal cell death. Front Mol Neurosci. 2017;10:229.
- Asghar U, Witkiewicz AK, Turner NC, Knudsen ES. The history and future of targeting Cyclin-dependent Kinases in cancer therapy. Nat Rev Drug Discov. 2015;14(2):130–146.
- Razga F, Nemethova V. Selective therapeutic intervention: a challenge against off-target effects. Trends Mol Med. 2017;23(8):671–674.
- Whittaker SR, Mallinger A, Workman P, Clarke PA. Inhibitors of cyclin-dependent kinases as cancer therapeutics. Pharmacol Ther. 2017; 173:83–105.
- Shapiro GI. Preclinical and clinical development of the cyclin-dependent kinase inhibitor flavopiridol. Clin Cancer Res. 2004;10(12 Pt 2):4270s–4275s.
- Benson C, White J, De Bono J, O'Donnell A, Raynaud F, Cruickshank C, McGrath H, Walton M, Workman P, Kaye S, et al. A phase I trial of the selective oral cyclin-dependent kinase inhibitor seliciclib (CYC202; R-Roscovitine), administered twice daily for 7 days every 21 days. Br J Cancer. 2007;96(1):29–37.
- Jackson RC, Barnett AL, McClue SJ, Green SR. Seliciclib, a cell cycle modulator that acts through the inhibition of cyclin-dependent kinases. Expert Opin Drug Discov. 2008;3(1):131–143.
- Tadesse S, Caldon EC, Tilley W, Wang S. Cyclin-dependent kinase 2 inhibitors in cancer therapy: an update. J Med Chem. 2019;62(9):4233–4251.
- Sung H, Ferlay J, Siegel RL, Laversanne M, Soerjomataram I, Jemal A, Bray F. Global cancer statistics 2020: GLOBOCAN estimates of incidence and mortality worldwide for 36 cancers in 185 countries. CA Cancer J Clin. 2021;71(3):209–249.
- Kawakami M, Mustachio LM, Rodriguez-Canales J, et al. Next-generation CDK2/9 inhibitors and anaphase catastrophe in lung cancer. J Natl Cancer Inst. 2017;109(6):109.
- Kodym E, Kodym R, Reis AE, Habib AA, Story MD, Saha D. The small-molecule CDK inhibitor, SNS-032, enhances cellular radiosensitivity in quiescent and hypoxic non-small cell lung cancer cells. Lung Cancer. 2009;66 (1):37–47.
- Raghavan P, Tumati V, Yu L, Chan N, Tomimatsu N, Burma S, Bristow RG, Saha D. AZD5438, an inhibitor of Cdk1, 2, and 9, enhances the radiosensitivity of non-small cell lung carcinoma cells. Int J Radiat Oncol Biol Phys. 2012;84(4):e507–e514.
- Al‐Jassas RM, Islam MS, Al-Majid AM, Nafie MS, Haukka M, Rahman AM, Alayyaf AMA, Barakat A. Synthesis and SARs study of novel spiro‐oxindoles as potent antiproliferative agents with CDK‐2 inhibitory activities. Arch Pharm. 2023;356(8):e2300185.
- Meng X, Zhu X, Ji J, Zhong H, Li X, Zhao H, Xie G, Wang K, Shu H, Wang X. Erdafitinib inhibits tumorigenesis of human lung adenocarcinoma A549 by inducing S-phase cell-cycle arrest as a CDK2 inhibitor. Molecules. 2022;27(19):6733.
- Nugiel DA, Vidwan A, Etzkorn AM, Rossi KA, Benfield PA, Burton CR, Cox S, Doleniak D, Seitz SP. Synthesis and evaluation of indenopyrazoles as cyclin-dependent kinase inhibitors. 2. Probing the indeno ring substituent pattern. J Med Chem. 2002;45(24):5224–5232.
- Shimomura I, Yokoi A, Kohama I, Kumazaki M, Tada Y, Tatsumi K, Ochiya T, Yamamoto Y. Drug library screen reveals benzimidazole derivatives as selective cytotoxic agents for KRAS-mutant lung cancer. Cancer Lett. 2019;451:11–22.
- El-Hameed RH, Fatahala SS, Sayed AI. Synthesis of some Novel Benzimidazole derivatives as anticancer agent and evaluation for CDK2 inhibition activity. Med Chem. 2022;18(2):238–248.
- Zhong W, Lalovic B, Zhan J. AG-024322, a novel cyclindependent kinase (CDK) inhibitor. Health. 2009;1(4):249–262.
- Sculier J, Ghisdal L, Berghmans T, Branle F, Lafitte JJ, Vallot F, Meer AP, Lemaitre F, Steels E, Burniat A, et al. The role of mitomycin in the treatment of non-small cell lung cancer: a systematic review with meta-analysis of the literature. Br J Cancer. 2001;84(9):1150–1155.
- Barakat A, Alshahrani S, Al-Majid AM, Alamary AS, Haukka M, Abu-Serie MM, Dömling A, Mazyed EA, Badria FA, El-Senduny FF. Novel spirooxindole based benzimidazole scaffold: in vitro, nanoformulation and in vivo studies on anticancer and antimetastatic activity of breast adenocarcinoma. Bioorg Chem. 2022;129:106124.
- Alshahrani S, Al-Majid AM, Ali M, Alamary AS, Abu-Serie MM, Dömling A, Shafiq M, Ul-Haq Z, Barakat A. Rational design, synthesis, separation, and characterization of new spiroxindoles combined with benzimidazole scaffold as an MDM2 inhibitor. Separations. 2023;10(4):225.
- CrysAlisPro. Rikagu Oxford Diffraction. Yarnton, Oxfordshire, England: Rikagu Oxford Diffraction Inc.; 2020.
- Sheldrick GM. SHELXT - Integrated space-group and crystal-structure determination. Acta Crystallogr A Found Adv 2015;71:3–8.
- Sheldrick GM. Crystal structure refinement with SHELXL. Acta Crystallogr C Struct Chem. 2015;71(Pt 1):3–8.
- Hübschle CB, Sheldrick GM, Dittrich B. ShelXle: a Qt graphical user interface for SHELXL. J Appl Crystallogr. 2011;44(Pt 6):1281–1284.
- Domingo LR. Molecular electron density theory: a modern view of reactivity in organic chemistry. Molecules. 2016;21(10):1319.
- Parr RG, Yang W. Density functional theory of atoms and molecules. New York: Oxford University Press; 1989.
- Domingo LR, Ríos-Gutiérrez M, Pérez P. Applications of the conceptual density functional indices to organic chemistry reactivity. Molecules. 2016;21(6):748.
- Domingo LR, Ríos-Gutiérrez M. Application of reactivity indices in the study of polar Diels–alder reactions. In: Liu S, editors, Conceptual density functional theory: towards a new chemical reactivity theory. Weinheim, German: WILEY-VCH GmbH; 2022, Volume 2, p. 481–502.
- Parr RG, Pearson RG. Absolute hardness: companion parameter to absolute electronegativity. J Am Chem Soc. 1983;105(26):7512–7516.
- Domingo LR. A new C–C bond formation model based on the quantum chemical topology of electron density. RSC Adv. 2014;4(61):32415–32428.
- Doming LR, Ríos-Gutiérrez M. A useful classification of organic reactions based on the flux of the electron density. Sci Rad. 2023;2(1):1–24.
- Parr RG, Szentpaly LV, Liu S. Electrophilicity index. J Am Chem Soc. 1999;121(9):1922–1924.
- Domingo LR, Chamorro E, Pérez P. Understanding the reactivity of captodative ethylenes in polar cycloaddition reactions. A theoretical study. J Org Chem. 2008;73(12):4615–4624.
- Evans MG, Polanyi M. Some applications of the transition state method to the calculation of reaction velocities, especially in solution. Trans Faraday Soc. 1935;31:875–894.
- Fukui K. Formulation of the reaction coordinate. J Phys Chem. 1970;74(23):4161–4163.
- Domingo LR, Sáez JA, Zaragozá RJ, Arnó M. Understanding the participation of Quadricyclane as nucleophile in polar cycloadditions toward electrophilic molecules. J Org Chem. 2008;73(22):8791–8799.
- Mosmann T. Rapid colorimetric assay for cellular growth and survival: application to proliferation and cytotoxicity assays. J Immunol Methods. 1983;65(1–2):55–63.
- Nafie MS, Kishk SM, Mahgoub S, Amer AM. Quinoline‐based thiazolidinone derivatives as potent cytotoxic and apoptosis‐inducing agents through EGFR inhibition. Chem Biol Drug Des. 2022;99(4):547–560.
- Shawish I, Nafie MS, Barakat A, Aldalbahi A, Al-Rasheed HH, Ali M, Alshaer W, Al Zoubi M, Al Ayoubi S, De la Torre BG, et al. Pyrazolyl-s-triazine with indole motif as a novel of epidermal growth factor receptor/cyclin-dependent kinase 2 dual inhibitors. Front Chem. 2022; 10:1078163.
- Dawood KM, Raslan MA, Abbas AA, Mohamed BE, Abdellattif MH, Nafie MS, Hassan MK. Novel Bis-Thiazole derivatives: synthesis and potential cytotoxic activity through apoptosis with molecular docking approaches. Front Chem. 2021;9:694870.
- Chai J-D, Head-Gordon M. Long-range corrected hybrid density functionals with damped atom–atom dispersion corrections. Phys Chem Chem Phys. 2008;10(44):6615–6620.
- Hehre MJ, Radom L, Schleyer PR, Pople J. Ab initio molecular orbital theory. New York: Wiley; 1986.
- Tomasi J, Persico M. Molecular interactions in solution: and overview of methods based on continuous distributions of the solvent. Chem Rev. 1994;94(7):2027–2094.
- Simkin. BYa, Sheikhet II. Quantum chemical and statistical theory of solutions– computational approach. London: Ellis Horwood; 1995.
- Cossi M, Barone V, Cammi R, Tomasi J. Ab initio study of solvated molecules: a new implementation of the polarizable continuum model. Chem Phys Lett. 1996;255(4–6):327–335.
- Cances E, Mennucci B, Tomasi J. A new integral equation formalism for the polarizable continuum model: theoretical background and applications to isotropic and anisotropic dielectrics. J. Chem. Phys. 1997;107(8):3032–3041.
- Barone V, Cossi M, Tomasi J. Geometry optimization of molecular structures in solution by the polarizable continuum model. J Comput Chem. 1998;19(4):404–417.
- Reed AE, Weinstock RB, Weinhold F. Natural population analysis. J Chem Phys. 1985;83(2):735–746.
- Reed AE, Curtiss LA, Weinhold F. Intermolecular interactions from a natural bond orbital, donor-acceptor viewpoint. Chem Rev. 1988;88(6):899–926.
- Frisch MJ. Gaussian 16, Revision A.03. Wallingford CT: Gaussian, Inc.; 2016.
- De Azevedo WF, Leclerc S, Meijer L, Havlicek L, Strnad M, Kim SH. Inhibition of cyclin‐dependent kinases by purine analogues: crystal structure of human cdk2 complexed with roscovitine. Eur J Biochem. 1997;243(1–2):518–526.
- “Molecular Operating Environment (MOE), 2013.08; Chemical Computing Group ULC, 1010 Sherbooke St. West, Suite #910, Montreal, QC, Canada, H3A 2R7, 2019.” 2019.
- Case DA, Aktulga HM, Belfon K, Ben-Shalom IY, Berryman JT, et al. Amber 2023. San Francisco: University of California. 2023.
- Khalil R, Ashraf S, Khalid A, Ul-Haq Z. Exploring Novel N-Myristoyltransferase inhibitors: a molecular dynamics simulation approach. ACS Omega. 2019;4(9):13658–13670.
- Roe DR, Cheatham TE. III PTRAJ and CPPTRAJ: software for processing and analysis of molecular dynamics trajectory data. J Chem Theory Comput. 2013;9(7):3084–3095.