Abstract
New thymol–3,4-disubstitutedthiazole hybrids were synthesised as dual COX-2/5-LOX inhibitors. Compounds 6b, 6d, 6e, and 6f displayed in vitro inhibitory activity against COX-2 (IC50= 0.037, 0.042, 0.046, and 0.039 µM) nearly equal to celecoxib (IC50= 0.045 µM). 6b, 6d, and 6f showed SI (379, 341, and 374, respectively) higher than that of celecoxib (327). 6a–l elicited in vitro 5-LOX inhibitory activity higher than quercetin. 6a–f, 6i–l, 7a, and 7c possessed in vivo inhibition of formalin induced paw edoema higher than celecoxib. 6a, 6b, 6f, 6h–l, and 7b showed gastrointestinal safety profile as celecoxib and diclofenac sodium in the population of fasted rats. Induced fit docking and molecular dynamics simulation predicted good fitting of 6b and 6f without changing the packing and globularity of the apo protein. In conclusion, 6b and 6f achieved the target goal as multitarget inhibitors of inflammation.
1. Introduction
Inflammation leads to the release of mediators which result in various cardiovascular and renal problemsCitation1. In the inflammatory process, cyclooxygenase (COX) and 5-lipoxygenase (5-LOX) enzymes produce various prostaglandins and leukotrienes (LTs) from arachidonic acid, respectivelyCitation1. The COX isoenzymes are endoplasmic reticulum-bound enzymes. The COX isoforms crystal structures are homologous. Each monomer consists of an N-terminal epidermal growth factor-like domain, a membrane-binding domain that anchors to the lipid bilayer, and a C-terminal catalytic domain that includes COX active siteCitation2,Citation3. Moreover, COX-1 is constitutive and is involved in homoeostatic processes in platelets, endothelium, and kidneys. Besides, COX-2 is constitutive in some tissues, like the brain and kidneys while it is inducible in inflammatory processesCitation4–9. On the other hand, LTs play a major role in inflammatory processes like allergic rhinitis, asthma, osteoarthritis, atopic dermatitis, gastric damage, ulceration ischaemia, atherosclerosis, septic shock, and tumorigenesisCitation10–23. In addition, LTC4 and LTD4 are potent contractors of blood vessels and bronchi smooth muscles and inducers of mucus secretion. Besides, LTB4 is a potent chemotactic and chemokinetic agentCitation19. Non-steroidal anti-inflammatory drugs (NSAIDs) have been applied for the treatment of pain, inflammation, arthritis, and fever. NSAIDs inhibit COX enzymes, so they suppress the production of prostaglandins. Dual inhibition of COX-1 and COX-2 by classical NSAIDs leads to various side effectsCitation24–28. Consequently, various selective COX-2 inhibitors like celecoxib, rofecoxib, and valdecoxib showed low gastrointestinal side effects, so they were clinically approved. However, these compounds were withdrawn from the market due to their cardiovascular side effectsCitation29–32. Dual inhibition of COX-1/COX-2 upregulates 5-LOX pathway, so it increases LTs synthesisCitation33. Consequently, simultaneous inhibition of COX-2 and 5-LOX enzymes could decrease the side effects of anti-inflammatory candidatesCitation34,Citation35. On the other hand, 1,5-diarylpyrazole was a major pharmacophore in various selective COX-2 inhibitors like celecoxib I. Besides, thymol II, a natural phenol derivative, was reported as a COX-2 inhibitor with anti-inflammatory activitiesCitation36–38. In addition, Thymus vulgaris essential oil which contains a significant percentage of thymol was proved to suppress 5-LOX activity in human monocytic cell line (THP-1)Citation39. It is worth mentioning that, the hybridisation of 1,5-diarylpyrazole to thymol produced compounds (IIIa–c) possessing in vitro dual inhibitory activities against COX-2 and 5-LOX higher than references celecoxib and quercetin IV (COX-2 IC50 for IIIa–c and celecoxib: 0.043, 0.045, 0.068, and 0.045 µM, respectively) (5-LOX IC50 for IIIa–c and quercetin: 3.05, 1.58, 1.60, and 3.34 µM, respectively)Citation40. In addition, compounds IIIa–c possessed in vivo potent anti-inflammatory activity higher than celecoxib and gastrointestinal safety profile as celecoxibCitation40. Moreover, hybridisation between thymol and thiazolidinone produced compounds Va,b which elicited in vitro dual COX-2/5-LOX inhibitory activities (COX-2 IC50: 0.058, 0.054 µM; 5-LOX IC50: 3.61, 3.26 µM respectively)Citation41. In addition, hybridisation between di-tert-butylphenol and thiazolidinone in darbufelone VI resulted in dual COX-2/5-LOX inhibitionCitation42. Furthermore, thiazole was considered bioisostere to pyrazole moiety, so various substituted thiazole derivatives were reported as COX-2 inhibitors, 5-LOX inhibitors and effective anti-inflammatory agents. Besides, 4-arylthiazole derivative VII showed 5-LOX inhibitory activity (IC50 = 10 μM) with anti-inflammatory activitiesCitation43. Moreover, combination of 4-arylthiazole with thiazolidinone in compound VIII showed 34% COX-2 inhibition, 5-LOX IC50, 54 µM, and 67.3% protection on in-vivo anti-inflammatory modelsCitation44. On the other hand, 3,4-diarylthiazole derivatives, IX and X revealed dual COX-2/5-LOX inhibition (COX-2 IC50 = 0.08, 0.77 µM; 5-LOX IC50 = 0.36, 0.58 µM, respectively)Citation45,Citation46 ().
In the present investigation, 3,4-disubstitutedthiazole pharmacophore, a bioisostere to the COX-2 inhibitor pharmacophore 1,5-diarylpyrazole, was hybridised with thymol, a natural phenolic 5-LOX inhibitor, aiming to act as dual COX-2/5-LOX inhibitors. These compounds could possess in vivo anti-inflammatory activities with better safety profile and fewer side effects than traditional NSAIDs ().
2. Experimental
2.1. Chemistry
Griffin melting point apparatus or electrothermal capillary tube melting point apparatus in open glass capillaries were used to measure melting points and were all uncorrected. Perkin-Elmer 1430 Infra-red spectrophotometer and Schimadzu FT-IR Affinity-1 Spectrometer were used to record infra-red spectra (IR) using KBr discs, in the Central Laboratory, Faculty of Pharmacy, Alexandria University, Egypt and in Faculty of Pharmacy, Cairo University, Egypt, respectively. Bruker High Performance Digital FT-NMR Spectrometer Avance III 400 MHz was used to determine nuclear magnetic resonance (1H-NMR and 13C-NMR) in Faculty of Pharmacy, Cairo University, Egypt using deuterated dimethylsulphoxide as a solvent. The data were reported as chemical shifts, or δ values (ppm) relative to tetramethylsilane (TMS) as internal standard. Signals are indicated by the following abbreviations: s = singlet, d = doublet, t = triplet, q = quartette, and m = multiplet. Gas chromatograph/mass spectrophotometer at Al-Azhar University (The Regional Centre of Microbiology and Biotechnology) was used to run electron impact mass spectra (EIMS). The most characteristic fragments were recorded as relative intensity %. Elemental microanalyses were performed at the microanalytical unit, Al-Azhar University (The Regional Centre of Microbiology and Biotechnology), Egypt. Thin-layer chromatography (TLC) on silica gel sheets (60 GF254, Merck) was used to monitor reaction progress. The spots were visualised by exposure to iodine vapour, or UV-lamp at λ 254 nm for few seconds.
Compounds 2Citation47–50, 3a–dCitation51,Citation52, 4a–dCitation41, and 5a–cCitation53–55 were prepared according to reported procedures.
2.1.1. General procedure for synthesis of 2-((2-Hydroxy-3-isopropyl-6-methylbenzylidene)hydrazono)-3,4-disubstituted-2,3-dihydrothiazoles (6a-l)
A mixture of the corresponding hydrazine carbothioamide 4a–d (0.1 mol), appropriate phenacyl bromide 5a–c (0.1 mol) and fused sodium acetate (0.15 mol) in glacial acetic acid (10 ml) was refluxed for 5–8 h. Then the reaction mixture was cooled to RT, poured onto crushed ice, the formed precipitate was filtered, washed with water, air-dried, and then recrystallized from ethanol.
2.1.1.1. 2-((2-Hydroxy-3-isopropyl-6-methylbenzylidene)hydrazono)-3,4-diphenyl-2,3-dihydrothiazole; 6a
Yellow crystals yield 82%, m.p0.175–177 °C. IR (KBr, v cm−1): 3421.72(OH); 1589.34, 1573.91(C = N). 1H-NMR (400 MHz, DMSO-d6δ ppm): 1.19(d, 6H, J = 6.88 Hz, 2CH3 of isopropyl); 2.32(s, 3H, CH3 of 6-methylthymol); 3.25-3.28(m, 1H, CH of isopropyl); 6.67(d, 1H, J = 7.68 Hz, C5 of thymol); 6.72(s, 1H, CH of thiazole); 7.10(d, 1H, J = 7.68 Hz, C4 of thymol); 7.18-7.20(m, 3H, C2, C4, C6 of N-phenyl);7.24-7.26(m, 3H, C3, C4, C5 of phenyl); 7.31-7.35 (m, 2H, C3, C5 of N-phenyl); 7.38-7.41(m, 2H, C2, C6 of phenyl); 8.52(s, 1H, CH of CH=N); 12.19(s, 1H, OH, D2O exchangeable).13C-NMR (100 MHz, DMSO-d6δ ppm): 19.29(CH3 of 6-methylthymol); 22.90(2CH3 of isopropyl); 26.32(CH of isopropyl); 101.17(CH of thiazole); 116.15(C1 of thymol); 121.40(C5 of thymol);127.92(C4 of phenyl); 128.72(3 C, C2, C4, C6 of N-phenyl); 128.92(2 C, C2, C6 of phenyl); 129.11 (2 C, C3, C5 of phenyl); 129.32(2 C, C3, C5 of N-phenyl); 129.54(C4 of thymol); 130.88(C1 of phenyl); 133.41(C3 of thymol); 136.02(C1 of N-phenyl); 137.65(C6 of thymol); 140.80(C4 of thiazole); 153.04(CH of CH = N); 156.46(C2 of thymol); 169.07(C = N of thiazole). EIMS, m/z (relative abundance %): 428 (M+.+1) (29.56); 427 (M+.) (100.00); 426 (3.5); 413 (3.98); 412 (13.74); 411 (6.69); 410 (12.94); 384 (2.43). Analysis Calcd for C26H25N3OS (427.57): C, 73.04; H, 5.89; N, 9.83; S, 7.50. Found: C, 72.88; H, 6.11; N, 9.97; S, 7.62.
2.1.1.2. 2-((2-Hydroxy-3-isopropyl-6-methylbenzylidene)hydrazono)-3-(2-chlorophenyl)-4-phenyl -2,3-dihydrothiazole; 6b
Pale yellow crystals yield 87%, m.p0.225–227 °C. IR (KBr, v cm−1): 3488.44(OH); 1593.20, 1573.91(C = N). 1H-NMR (400 MHz, DMSO-d6δ ppm): 1.19(d, 6H, J = 6.88 Hz, 2CH3 of isopropyl); 2.31(s, 3H, CH3 of 6-methylthymol); 3.27-3.28(m, 1H, CH of isopropyl); 6.67(d, 1H, J = 7.72 Hz, C5 of thymol); 6.74(s, 1H, CH of thiazole); 7.11(d, 1H, J = 7.72 Hz, C4 of thymol); 7.24-7.29(m, 5H, C3, C4, C5 of phenyl, C5, C6 of chlorophenyl);7.42-7.44(m, 2H, C2, C6 of phenyl); 7.54-7.56 (m, 1H, C4 of chlorophenyl); 7.67-7.69(m, 1H, C3 of chlorophenyl); 8.50(s, 1H, CH of CH=N); 12.18(s, 1H, OH, D2O exchangeable). 13C-NMR (100 MHz, DMSO-d6δ ppm): 19.29(CH3 of 6-methylthymol); 22.83 (2CH3 of isopropyl); 26.34(CH of isopropyl); 100.99(CH of thiazole); 116.06(C1 of thymol); 121.43(C5 of thymol);128.06(C4 of chlorophenyl); 128.75, 128.81(5 C, C2, C3, C5, C6 of phenyl, C6 of chlorophenyl); 129.48 (C4 of thymol); 130.32(C4 of phenyl); 130.68(C2 of chlorophenyl); 131.39(C1 of phenyl); 132.70(2 C, C1, C3 of chlorophenyl); 133.42(C3 of thymol); 135.17(C5 of chlorophenyl); 136.18(C6 of thymol); 140.40(C4 of thiazole); 153.49(CH of CH = N); 156.51(C2 of thymol); 168.36(C = N of thiazole). EIMS, m/z (relative abundance %): 463 (M+.+2) (39.97); 462 (M+.+1) (32.90); 461 (M+.) (100.00); 448 (6.04); 447 (5.51); 446 (16.76); 445 (4.07); 444 (7.79); 428 (2.25); 427 (4.30); 426 (13.11); 409 (4.24). Analysis Calcd for C26H24ClN3OS (461.13): C, 67.59; H, 5.24; N, 9.10; S, 6.94. Found: C, 67.73; H, 5.47; N, 9.26; S, 6.89.
2.1.1.3. 2-((2-Hydroxy-3-isopropyl-6-methylbenzylidene)hydrazono)-3-(2-pyridyl)-4-phenyl-2,3-dihydrothiazole; 6c
Yellow crystals yield 85%, m.p0.179–181 °C. IR (KBr, v cm−1): 3421.72(OH); 1566.20, 1597.06(C = N). 1H-NMR (400 MHz, DMSO-d6δ ppm): 1.19(d, 6H, J = 6.92 Hz, 2CH3 of isopropyl); 2.32(s, 3H, CH3 of 6-methylthymol); 3.22-3.279(m, 1H, CH of isopropyl); 6.68(d, 1H, J = 7.72 Hz, C5 of thymol); 6.74(s, 1H, CH of thiazole); 7.11(d, 1H, J = 7.72 Hz, C4 of thymol); 7.15-7.17(m, 2H, C2, C6 of phenyl);7.22-7.25(m, 3H, C3, C4, C5 of phenyl); 7.38-7.41 (m, 1H, C4 of 2-pyridyl); 7.74 (d, 1H, J = 7.96 Hz, C3 of 2-pyridyl); 7.97-8.01(m, 1H, C5 of 2-pyridyl); 8.40(d, 1H, J = 3.8 Hz, C6 of 2-pyridyl); 8.54(s, 1H, CH of CH=N); 12.15(s, 1H, OH, D2O exchangeable). EIMS, m/z (relative abundance %): 429 (M+.+1) (42.82); 428 (M+.) (100.00); 413 (4.95); 411 (4.09). Analysis Calcd for C25H24N4OS (428.55): C, 70.07; H, 5.65; N, 13.07; S, 7.48. Found: C, 70.34; H, 5.82; N, 12.89; S, 7.60.
2.1.1.4. 2-((2-Hydroxy-3-isopropyl-6-methylbenzylidene)hydrazono)-3-(4-methoxyphenyl)-4-phenyl-2,3-dihydrothiazole; 6d
Khaki crystals yield 85%, m.p0.195–198 °C. IR (KBr, v cm−1): 3425.58(OH); 1589.34, 1573.91(C = N). 1H-NMR (400 MHz, DMSO-d6δ ppm): 1.19(d, 6H, J = 6.92 Hz, 2CH3 of isopropyl); 2.32(s, 3H, CH3 of 6-methylthymol); 3.18-3.28(m, 1H, CH of isopropyl); 3.75(s, 3H, CH3 of OCH3); 6.67(d, 1H, J = 7.68 Hz, C5 of thymol); 6.68(s, 1H, CH of thiazole); 6.92(d, 2H, J = 8.92 Hz, C2, C6 of p-methoxyphenyl); 7.10(d, 1H, J = 7.68 Hz, C4 of thymol); 7.20-7.28(m, 7H, C2, C3, C4, C5, C6 of phenyl, C3, C5 of p-methoxy phenyl); 8.52(s, 1H, CH of CH=N); 12.21(s, 1H, OH, D2O exchangeable). EIMS, m/z (relative abundance %): 458 (M+.+1) (34.60); 457 (M+.) (100.00); 443 (3.19); 442 (10.82); 441 (5.30); 440 (9.56); 281 (2.5). Analysis Calcd for C27H27N3O2S (457.59): C, 70.87; H, 5.95; N, 9.18; S, 7.01. Found: C, 71.06; H, 6.12; N, 9.41; S, 7.08.
2.1.1.5. 2-((2-Hydroxy-3-isopropyl-6-methylbenzylidene)hydrazono)-3-phenyl-4-(4-bromophenyl) -2,3-dihydrothiazole; 6e
Linen crystals yield 88%, m.p0.211–213 °C. IR (KBr, v cm−1): 3417.86(OH); 1589.34 (C = N). 1H-NMR (400 MHz, DMSO-d6δ ppm): 1.19(d, 6H, J = 6.92 Hz, 2CH3 of isopropyl); 2.32(s, 3H, CH3 of 6-methylthymol); 3.28-3.31(m, 1H, CH of isopropyl); 6.67(d, 1H, J = 7.72 Hz, C5 of thymol); 6.78(s, 1H, CH of thiazole); 7.10(d, 1H, J = 7.72 Hz, C4 of thymol); 7.14(d, 2H, J = 8.52 Hz, C2, C6 of bromophenyl); 7.33-7.37(m, 3H, C3, C4, C5 of phenyl); 7.41(d, 2H, J = 7.64 Hz, C2, C6 of phenyl); 7.46(d, 2H, J = 8.52 Hz, C3, C5 of bromophenyl); 8.52(s, 1H, CH of CH=N); 12.17(s, 1H, OH, D2O exchangeable). EIMS, m/z (relative abundance %): 509 (M+.+2) (10.04); 508 (M+.+1) (32.16); 507 (M+.) (100.00); 506 (37.01); 505 (9.09); 492 (12.74); 491 (9.18); 490 (22.40); 489 (6.10); 488 (11.02); 331 (8.19); 329 (4.19). Analysis Calcd for C26H24BrN3OS (506.46): C, 61.66; H, 4.78; N, 8.30; S, 6.33. Found: C, 61.52; H, 4.89; N, 8.56; S, 6.49.
2.1.1.6. 2-((2-Hydroxy-3-isopropyl-6-methylbenzylidene)hydrazono)-3-(2-chlorophenyl)-4-(4-bromophenyl)-2,3-dihydrothiazole; 6f
Yellow crystals yield 85%, m.p0.185–188 °C. IR (KBr, v cm−1): 3479.58(OH); 1593.20 (C = N). 1H-NMR (400 MHz, DMSO-d6δ ppm): 1.19(d, 6H, J = 6.84 Hz, 2CH3 of isopropyl); 2.31(s, 3H, CH3 of 6-methylthymol); 3.27-3.31(m, 1H, CH of isopropyl); 6.67(d, 1H, J = 7.76 Hz, C5 of thymol); 6.81(s, 1H, CH of thiazole); 7.10(d, 1H, J = 7.76 Hz, C4 of thymol); 7.19(d, 2H, J = 8.48 Hz, C2, C6 of bromophenyl); 7.44-7.49(m, 4H, C3, C5 of bromophenyl, C4, C6 of chlorophenyl); 7.56-7.59(m, 1H, C5 of chlorophenyl); 7.70-7.73(m, 1H, C3 of chlorophenyl); 8.50(s, 1H, CH of CH=N); 12.16(s, 1H, OH, D2O exchangeable). 13C-NMR (100 MHz, DMSO-d6δ ppm): 19.29(CH3 of 5-methyl thymol); 22.92(2CH3 of isopropyl); 26.35(CH of isopropyl); 101.72(CH of thiazole); 116.02(C1 of thymol); 121.44(C5 of thymol); 122.98(C4 of bromophenyl); 128.11 (C6 of chlorophenyl); 128.83(C4 of chlorophenyl); 129.51 (C4 of thymol); 130.76(C2 of chlorophenyl); 130.82(2 C, C2, C6 of chlorophenyl); 131.54(C1 of bromophenyl); 131.79(2 C, C3, C5 of bromophenyl); 132.57(C3 of chlorophenyl); 132.71(C5 of chlorophenyl); 133.43(C3 of thymol); 134.92 (C1 of chlorophenyl); 136.22(C6 of thymol); 139.21(C4 of thiazole); 153.69(CH of CH = N); 156.53(C2 of thymol); 168.20(C = N of thiazole). EIMS, m/z (relative abundance %): 545 (M+.+4)(8.05); 543 (M+.+2) (22.45); 542(M+.+1) (34.58); 541 (M+.) (100.00); 540 (29.41); 539 (76.77); 526 (14.02); 525 (5.50); 524 (14.79); 506 (8.73); 504 (8.18). Analysis Calcd for C26H23BrClN3OS (540.90): C, 57.73; H, 4.29; N, 7.77; S, 5.93. Found: C, 58.01; H, 4.42; N, 7.92; S, 6.04.
2.1.1.7. 2-((2-Hydroxy-3-isopropyl-6-methylbenzylidene)hydrazono)-3-(2-pyridyl)-4-(4-bromophenyl)-2,3-dihydrothiazole; 6 g
Light yellow crystals yield 79%, m.p0.112–115 °C. IR (KBr, v cm−1): 3421.72(OH); 1589.34, 1570.06 (C = N). 1H-NMR (400 MHz, DMSO-d6δ ppm): 1.19(d, 6H, J = 6.92 Hz, 2CH3 of isopropyl); 2.33(s, 3H, CH3 of 6-methylthymol); 3.28-3.32(m, 1H, CH of isopropyl); 6.68(d, 1H, J = 7.76 Hz, C5 of thymol); 6.81(s, 1H, CH of thiazole); 7.10-7.13(m, 3H, C4 of thymol, C3, C5 of bromophenyl); 7.40-7.47(m, 3H, C2, C6 of bromophenyl, C4 of 2-pyridyl); 7.78(d, 1H, J = 7.96 Hz, C3 of 2-pyridyl); 8.00-8.04(m, 1H, C5 of 2-pyridyl); 8.40 (d, 1H, J = 4.8 Hz, C6 of 2-pyridyl); 8.55(s, 1H, CH of CH=N); 12.12(s, 1H, OH, D2O exchangeable). EIMS, m/z (relative abundance %): 510 (M+.+2) (9.15); 509 (M+.+1) (29.52); 508 (M+.) (100.00); 507 (33.70); 506 (97.12); 493 (11.29); 492 (7.76); 491 (19.78); 490 (5.28); 489 (10.16); 332 (3.11). Analysis Calcd for C25H23BrN4OS (507.45): C, 59.17; H, 4.57; N, 11.04; S, 6.32. Found: C, 59.39; H, 4.65; N, 11.32; S, 6.49.
2.1.1.8. 2-((2-Hydroxy-3-isopropyl-6-methylbenzylidene)hydrazono)-3-(4-methoxyphenyl)-4-(4-bromophenyl)-2,3-dihydrothiazole; 6h
Cream crystals yield 86%, m.p0.255–258 °C. IR (KBr, v cm−1): 3421.72(OH); 1589.34 (C = N). 1H-NMR (400 MHz, DMSO-d6δ ppm): 1.19(d, 6H, J = 6.88 Hz, 2CH3 of isopropyl); 2.32(s, 3H, CH3 of 6-methylthymol); 3.28-3.30(m, 1H, CH of isopropyl); 3.76(s, 3H, CH3 of OCH3); 6.67(d, 1H, J = 7.68 Hz, C5 of thymol); 6.74(s, 1H, CH of thiazole); 6.94(d, 2H, J = 8.80 Hz, C2, C6 of p-methoxyphenyl); 7.10(d, 1H, J = 7.68 Hz, C4 of thymol); 7.16(d, 2H, J = 8.40 Hz, C2, C6 of bromophenyl); 7.25(d, 2H, J = 8.80 Hz, C3, C5 of p-methoxy phenyl); 7.48(d, 2H, J = 8.40 Hz, C3, C5 of bromophenyl); 8.52(s, 1H, CH of CH=N); 12.18(s, 1H, OH, D2O exchangeable). EIMS, m/z (relative abundance %): 539 (M+.+2) (8.58); 538 (M+.+1) (29.34); 537 (M+.) (100.00); 536 (32.61); 535 (91.43); 522 (14.57); 521 (11.27); 520 (28.32); 518 (13.43); 363 (25.64); 362 (30.19); 361 (84.06); 360 (29.54); 359 (62.40); 348 (12.34); 347 (9.25); 346 (12.46). Analysis Calcd for C27H26BrN3O2S (536.49): C, 60.45; H, 4.89; N, 7.83; S, 5.98. Found: C, 60.71; H, 4.95; N, 7.98; S, 6.12.
2.1.1.9. 2-((2-Hydroxy-3-isopropyl-6-methylbenzylidene)hydrazono)-3-phenyl-4-(4-methoxyphenyl)-2,3-dihydrothiazole; 6i
Light yellow crystals yield 84%, m.p0.208–210 °C. IR (KBr, v cm−1): 3483.44(OH); 1589.34 (C = N). 1H-NMR (400 MHz, DMSO-d6δ ppm): 1.19(d, 6H, J = 6.92 Hz, 2CH3 of isopropyl); 2.31(s, 3H, CH3 of 6-methylthymol); 3.28-3.30(m, 1H, CH of isopropyl); 3.70(s, 3H, CH3 of OCH3); 6.60(s, 1H, CH of thiazole); 6.67(d, 1H, J = 7.72 Hz, C5 of thymol); 6.79(d, 2H, J = 8.80 Hz, C3, C5 of p-methoxyphenyl); 7.09-7.14(m, 3H, C4 of thymol, C2, C6 of p-methoxyphenyl); 7.30-7.43 (m, 5H, C2, C3, C4, C5, C6 of phenyl,); 8.51(s, 1H, CH of CH=N); 12.21(s, 1H, OH, D2O exchangeable). 13C-NMR (100 MHz, DMSO-d6δ ppm): 19.29(CH3 of 6-methylthymol); 22.90(2CH3 of isopropyl); 26.32(CH of isopropyl); 55.59(CH3 of OCH3); 99.91(CH of thiazole); 114.13(2 C, C3, C5 of p-methoxyphenyl); 116.17(C1 of thymol); 121.38(C5 of thymol); 123.15(C4 of phenyl); 127.86(C1 of p-methoxyphenyl); 128.71(2 C, C2, C6 of phenyl); 129.39(2 C, C3, C5 of phenyl); 129.54(C4 of thymol); 130.38(2 C, C2, C6 of p-methoxyphenyl); 133.41(C3 of thymol); 135.97(C1 of phenyl); 137.72(C6 of thymol); 140.65(C4 of thiazole); 152.83(CH of CH = N); 156.44(C2 of thymol); 159.79(C4 of p-methoxyphenyl); 169.14(C = N of thiazole). EIMS, m/z (relative abundance %): 458 (M+.+1) (33.22); 457 (M+.) (100.00); 442 (10.94); 441 (6.02); 440 (10.72); 382 (2.45); 381 (4.81). Analysis Calcd for C27H27N3O2S (457.59): C, 70.87; H, 5.95; N, 9.18; S, 7.01. Found: C, 71.23; H, 6.12; N, 9.40; S, 7.06.
2.1.1.10. 2-((2-Hydroxy-3-isopropyl-6-methylbenzylidene)hydrazono)-3-(2-chlorophenyl)-4-(4-methoxyphenyl)-2,3-dihydrothiazole; 6j
Light yellow crystals yield 85%, m.p0.183–184 °C. IR (KBr, v cm−1): 3421.72(OH); 1597.06(C = N). 1H-NMR (400 MHz, DMSO-d6δ ppm): 1.19(d, 6H, J = 6.84 Hz, 2CH3 of isopropyl); 2.31(s, 3H, CH3 of 6-methylthymol); 3.28-3.32(m, 1H, CH of isopropyl); 3.70(s, 3H, CH3 of OCH3); 6.63(s, 1H, CH of thiazole); 6.67(d, 1H, J = 7.72 Hz, C5 of thymol); 6.81(d, 2H, J = 8.76 Hz, C3, C5 of p-methoxyphenyl); 7.10(d, 1H, J = 7.72 Hz, C4 of thymol); 7.16(d, 2H, J = 8.76 Hz, C2, C6 of p-methoxyphenyl); 7.41-7.46 (m, 2H, C4, C6 of chlorophenyl); 7.55-7.57(m, 1H, C5 of chlorophenyl); 7.65-7.67(m, 1H, C3 of chlorophenyl); 8.48(s, 1H, CH of CH=N); 12.20(s, 1H, OH, D2O exchangeable). EIMS, m/z (relative abundance %): 493 (M+.+2) (39.29); 492 (M+.+1) (30.70); 491 (M+.) (100.00); 478 (6.28); 477 (6.12); 476 (18.69); 475 (4.65); 474 (8.89); 458 (3.55); 457 (6.61); 456 (17.99); 441 (2.94); 440 (3.48); 439 (9.87). Analysis Calcd for C27H26ClN3O2S (492.03): C, 65.91; H, 5.33; N, 8.54; S, 6.52. Found: C, 66.12; H, 5.42; N, 8.82; S, 6.63.
2.1.1.11. 2-((2-Hydroxy-3-isopropyl-6-methylbenzylidene)hydrazono)-3-(2-pyridyl)-4-(4-methoxyphenyl)-2,3-dihydrothiazole; 6k
Lemon chiffon crystals yield 85%, m.p0.150–153 °C. IR (KBr, v cm−1): 3483.44(OH); 1597.06, 1570.08(C = N). 1H-NMR (400 MHz, DMSO-d6δ ppm): 1.19(d, 6H, J = 6.88 Hz, 2CH3 of isopropyl); 2.32(s, 3H, CH3 of 6-methylthymol); 3.28-3.32(m, 1H, CH of isopropyl); 3.70(s, 3H, CH3, OCH3); 6.62(s, 1H, CH of thiazole); 6.68(d, 1H, J = 7.76 Hz, C5 of thymol); 6.79(d, 2H, J = 8.84 Hz, C3, C5 of p-methoxyphenyl); 7.07-7.12(m, 3H, C4 of thymol, C2, C6 of p-methoxyphenyl); 7.40-7.43(m, 1H, C4 of 2-pyridyl); 7.69(d, 1H, J = 7.92 Hz, C3 of 2-pyridyl); 7.96-8.01(m, 1H, C5 of 2-pyridyl); 8.43-8.45(d, 1H, J = 8 Hz, C6 of 2-pyridyl); 8.52(s, 1H, CH of CH=N); 12.16(s, 1H, OH, D2O exchangeable). EIMS, m/z (relative abundance %): 459 (M+.+1) (26.96); 458 (M+.) (100.00); 443 (14.74); 442 (7.95); 441 (18.12); 352 (6.21). Analysis Calcd for C26H26N4O2S (458.58): C, 68.10; H, 5.72; N, 12.22; S, 6.99. Found: C, 68.34; H, 5.83; N, 12.47; S, 7.14.
2.1.1.12. 2-((2-Hydroxy-3-isopropyl-6-methylbenzylidene)hydrazono)-3,4-di(4-methoxyphenyl)-2,3-dihydrothiazole; 6 l
Ivory crystals yield 80%, m.p0.235–238 °C. IR (KBr, v cm−1): 3479.58(OH); 1589.34, 1573.91 (C = N). 1H-NMR (400 MHz, DMSO-d6δ ppm): 1.19(d, 6H, J = 6.84 Hz, 2CH3 of isopropyl); 2.32(s, 3H, CH3 of 6-methylthymol); 3.28-3.30(m, 1H, CH of isopropyl); 3.71, 3.76(2s, 6H, 2OCH3); 6.57(s, 1H, CH of thiazole); 6.67(d, 1H, J = 7.68 Hz, C5 of thymol); 6.82(d, 2H, J = 8.64 Hz, C2, C6 of N-p-methoxyphenyl); 6.93(d, 2H, J = 8.80 Hz, C3, C5 of p-methoxyphenyl); 7.10(d, 1H, J = 7.68 Hz, C4 of thymol); 7.13(d, 2H, J = 8.64 Hz, C3, C5 of N-p-methoxyphenyl); 7.23(d, 2H, J = 8.80 Hz, C2, C6 of p-methoxyphenyl); 8.50(s, 1H, CH of CH=N); 12.22(s, 1H, OH, D2O exchangeable). EIMS, m/z (relative abundance %): 488 (M+.+1) (42.70); 487 (M+.) (100.00); 472(10.10); 471 (4.84); 470 (10.74). Analysis Calcd for C28H29N3O3S (487.62): C, 68.97; H, 5.99; N, 8.62; S, 6.57. Found: C, 69.21; H, 6.15; N, 8.90; S, 6.72.
2.1.2. General procedure for synthesis of 2-((2-Hydroxy-3-isopropyl-6-methylbenzylidene)hydrazono)-4-methyl-3-substituted-2,3-dihyrothiazoles (7a-c)
A mixture of the corresponding hydrazine carbothioamide 4a–c (1 mmol) and chloroacetone (1 mmol) was refluxed with fused sodium acetate (1.5 mmol) in glacial acetic acid for 6–8 h, cooling to RT, pouring on to crushed ice, then filtering the precipitate, air-dried, recrystallization from ethanol.
2.1.2.1. 2-((2-Hydroxy-3-isopropyl-6-methylbenzylidene)hydrazono)-4-methyl-3-phenyl-2,3-dihyrothiazole; 7a
Dark goldenrod crystals yield 60%, m.p0.100–101 °C. IR (KBr, v cm−1): 3406.29(OH); 1589.34, 1570.06(C = N). 1H-NMR (400 MHz, DMSO-d6δ ppm): 1.18(d, 6H, J = 6.92 Hz, 2CH3 of isopropyl); 1.85(s, 3H, CH3 of thiazole); 2.28(s, 3H, CH3 of 6-methylthymol); 3.26-3.29(m, 1H, CH of isopropyl); 6.34(s, 1H, CH of C5 of thiazole); 6.64(d, 1H, J = 7.72 Hz, C5 of thymol); 7.06(d, 1H, J = 7.72 Hz, C4 of thymol); 7.44(d, 2H, J = 7.24 Hz, C2, C6 of phenyl); 7.48-7.52(m, 1H, C4 of phenyl);7.55-7.58(m, 2H, C3, C5 of phenyl); 8.45(s, 1H, CH of CH=N); 12.24(s, 1H, OH, D2O exchangeable). 13C-NMR (100 MHz, DMSO-d6δ ppm): 14.99(CH3 of thiazole); 19.26(CH3 of 6-methylthymol); 22.86(2CH3 of isopropyl); 26.34(CH of isopropyl); 97.41(CH of C5 of thiazole); 116.22(C1 of thymol); 121.30(C5 of thymol); 127.64(C4 of phenyl); 129.19(2 C, C2, C6 of phenyl); 129.37(C4 of thymol); 130.09(2 C, C3, C5 of phenyl); 133.35(C3 of thymol); 135.78(C1 of phenyl); 136.59(C6 of thymol); 137.04(C4 of thiazole); 152.17(CH of CH = N); 156.39(C2 of thymol); 169.46(C = N of thiazole). Analysis Calcd for C21H23N3OS (365.50): C, 69.01; H, 6.34; N, 11.50; S, 8.77. Found: C, 69.28; H, 6.43; N, 11.15; S, 8.59.
2.1.2.2. 2-((2-Hydroxy-3-isopropyl-6-methylbenzylidene)hydrazono)-4-methyl-3-(2-chlorophenyl)-2,3-dihyrothiazole; 7b
Chocolate crystals yield 58%, m.p0.169–171 °C. IR (KBr, v cm−1): 3421.72(OH); 1587.70 (C = N). 1H-NMR (400 MHz, DMSO-d6δ ppm): 1.18(d, 6H, J = 6.88 Hz, 2CH3 of isopropyl); 1.81(s, 3H, CH3 of thiazole); 2.29(s, 3H, CH3 of 6-methylthymol); 3.28-3.31(m, 1H, CH of isopropyl); 6.38(s, 1H, CH of C5 of thiazole); 6.64(d, 1H, J = 7.68 Hz, C5 of thymol); 7.07(d, 1H, J = 7.68 Hz, C4 of thymol); 7.56-7.59(m, 2H, C4, C6 of chlorophenyl); 7.64-7.65(m, 1H, C5 of chlorophenyl); 7.73-7.75(m, 1H, C3 of chlorophenyl); 8.45(s, 1H, CH of CH=N); 12.22(s, 1H, OH, D2O exchangeable). Analysis Calcd for C21H22ClN3OS (399.94): C, 63.07; H, 5.54; N, 10.51; S, 8.02. Found: C, 62.93; H, 5.36; N, 10.24; S, 7.93.
2.1.2.3. 2-((2-Hydroxy-3-isopropyl-6-methylbenzylidene)hydrazono)-4-methyl-3-(4-methoxyphenyl)-2,3-dihyrothiazole; 7c
Saddle brown crystals yield 52%, m.p0.158–160 °C. IR (KBr, v cm−1): 3421.72(OH); 1589.34, 1573.91(C = N). 1H-NMR (400 MHz, DMSO-d6δ ppm): 1.12(d, 6H, J = 6.84 Hz, 2CH3 of isopropyl); 1.92(s, 3H, CH3 of thiazole); 2.04(s, 3H, CH3 of 6-methylthymol); 3.22-3.27(m, 1H, CH of isopropyl); 3.32(s, 3H, CH3 of OCH3); 6.34(s, 1H, CH of C5 of thiazole); 6.59(d, 1H, J = 7.64 Hz, C5 of thymol); 6.66(d, 2H, J = 8.76 Hz, C2, C6 of p-methoxyphenyl); 6.88(d, 1H, J = 7.64 Hz, C4 of thymol); 7.09(d, 2H, J = 8.76 Hz, C3, C5 of p-methoxyphenyl); 8.65(s, 1H, CH of CH=N); 12.20(s, 1H, OH, D2O exchangeable). 13C-NMR (100 MHz, DMSO-d6δ ppm): 14.90(CH3 of thiazole); 19.27(CH3 of 6-methylthymol); 22.88(2CH3 of isopropyl); 26.35(CH of isopropyl); 57.10(CH3 of OCH3); 97.91(CH of C5 of thiazole); 115.71(2 C, C3, C5 of p-methoxyphenyl); 118.82(C1 of thymol); 121.35(C5 of thymol); 127.86(C1 of p-methoxyphenyl); 128.58(C4 of thymol); 133.40(2 C, C2, C6 of p-methoxyphenyl); 133.58(C3 of thymol); 136.42(C6 of thymol); 139.89(C4 of thiazole); 150.41(CH of CH = N); 156.22(C2 of thymol); 156.46(C4 of p-methoxyphenyl); 168.83(C = N of thiazole). Analysis Calcd for C22H25N3O2S (395.52): C, 66.81; H, 6.37; N, 10.62; S, 8.11. Found: C, 67.12; H, 6.21; N, 10.30; S, 8.37.
2.2. Biological screening
2.2.1. In vitro COX-1 and COX-2 inhibitory assay
Compounds 6a–l and 7a–c were tested for their in vitro COX-1 and COX-2 inhibitory activities using Cayman colorimetric COX (ovine) inhibitor screening assay kit (Catalog No. 560131) supplied by Cayman chemicals, Ann Arbour, MI, USA according to reported methodCitation41,Citation56. (Page: S34, Supp. file)
2.2.2. In vitro 5-LOX inhibitory assay
Compounds 6a–l and 7a–c were tested for their in vitro 5-LOX inhibitory activity using Abnova 5-LOX inhibitor screening assay kit (Catalog No. 760700) according to reported method Citation41,Citation57. (Page: S34–35, Supp. file)
2.2.3. In vivo anti-inflammatory activity
2.2.3.1. Formalin-induced paw edoema
Compounds 6a–l and 7a–c were evaluated for their in vivo anti-inflammatory activity using formalin-induced paw edoema screening protocol as an acute inflammation model using celecoxib and diclofenac sodium as reference drugs according to reported proceduresCitation41,Citation58,Citation59 (Approved by AlexU-IACUC)Citation60. (Page: S37-39, Supp. file)
2.2.3.2. Gastric ulcerogenic activity
Acute gastric ulcerogenic effect was evaluated in adult female Wistar rats according to reported proceduresCitation41,Citation59,Citation61 (Approved by AlexU-IACUC)Citation60. (Page: S40, Supp. file)
2.3. Molecular modelling
2.3.1. Induced fit docking to COX-2 and 5-LOX
Molecular Operating Environment (MOE 2016.08) software (Chemical Computing Group, Montreal, Canada)Citation62 was used to perform the molecular modelling studies. The proteins crystal structures were downloaded from the Protein Data Bank (PDB) website. (Page: S41, Supp. file)
2.3.2. Molecular dynamics simulation
Molecular dynamics simulation of protein–ligand complexes was performed by using WebGROCitation63,Citation64. (Page: S41-44, Supp. file)
3. Results and discussion
3.1. Chemistry
Scheme 1 outlined the synthesis of the target compounds. It started with the synthesis of 2-formylthymol (2) by reaction of thymol (1) with magnesium methoxide and paraformaldehydeCitation47–50. The 2-formylthymol (2) was then reacted with various substituted thiosemicarbazides (3a–d)Citation51,Citation52 in the presence of a few drops of glacial acetic acid in ethanol, to give thiosemicarbazones (4a–d)Citation41. Compounds 4a-d were further cyclized by refluxing with α-haloketone (namely, substituted phenacyl bromide, or α-chloroacetone) in glacial acetic acid containing fused sodium acetate to afford the corresponding 3,4-disubstitutedthiazole derivatives 6a–l and 7a–c, respectively. Spectroscopic data have been utilised to elucidate the chemical structures of the target thiazole derivatives. The presence of C5-H of thiazole ring in compounds 6a–l was demonstrated by 1H-NMR and 13C-NMR spectroscopy which exhibited singlet signal at (6.60 − 6.74) ppm for one proton, and (99.91–101.72) ppm, respectively. The structures of the prepared compounds 7a–c were confirmed by studying the IR, 1H-NMR as well as 13C-NMR spectra for these compounds. IR spectra were characterised by the absence of absorption bands assigned to (NH) and (C = S) groups, and the presence of absorption bands assigned to (C = N), in addition to (OH) group at expected absorption regions. 1H-NMR spectra of compounds 7a–c were characterised by the absence of signals assigned to NH protons, and the presence of singlet signals assigned to protons of CH3 at C4 of thiazole and proton at C5 of thiazole at 1.81–1.85 ppm and 6.30–6.38 ppm, respectively. 13C-NMR spectra of compounds 7a and 7c showed the presence of signals of CH3 at C4 of thiazole, C5 of thiazole and C = N at 137–138.36 ppm, 96.94–97.41 ppm and 169.46–169.73 ppm, respectively.
3.2. Biological screening
3.2.1. In vitro cox-1 and cox-2 inhibitory assay
Compounds 6a–6l and 7a–7c were screened for their ability to inhibit COX-1 and COX-2 enzymes in vitro. The results () revealed that all compounds showed lower inhibitory activity against COX-1 with IC50 8.01 − 14.58 µM compared to diclofenac sodium (IC50 = 3.9 µM). Among the tested compounds, compound 7a was the most active against COX-1. In addition, compounds 6b, 6d, 6e, and 6f showed COX-2 inhibitory activities nearly equal to that of celecoxib. Moreover, compounds 6b, 6d, and 6f showed SI (379, 341, and 374, respectively) higher than that of celecoxib (327) while 6e SI (301) was comparable to celecoxib. Furthermore, compounds 6a, 6c, 6 g, and 6h were slightly less active than celecoxib while compounds 6i–6l showed moderate inhibitory activity against COX-2. On the other hand, compound 7c showed nearly half the activity of celecoxib, while 7a and 7b elicited lower inhibitory activity than celecoxib (). It is worth mentioning that 4-arylthiazole derivatives (6a–6l) showed higher COX-2 selectivity and inhibitory activity than 4-methylthiazole derivatives (7a–7c). In addition, substitution at the para position of the phenyl moiety at position 4 of thiazole with lipophilic bromo halogen (6e–6h) resulted in increased COX-2 inhibitory activity and selectivity followed by the unsubstituted derivatives (6a–6d). While the substitution with polar methoxy group resulted in decreased activity. Furthermore, substitution at position 3 of thiazole with lipophilic 2-chlorophenyl moiety increased COX-2 selectivity and inhibitory activity (6b and 6f).
Table 1. In vitro COX-1, COX-2, and 5-LOX enzyme inhibitory activities, aIC50 values and bSI of the tested compounds.
.
3.2.2. In vitro 5-lox inhibitory assay
Compounds 6a–6l and 7a–7c were screened for their ability to inhibit 5-lipoxygenase enzyme. The results listed in revealed that compounds 6a–6l showed 5-LOX inhibitory activities higher than quercetin while compounds 7a–7c elicited slightly less activity than quercetin (). Moreover, 4-arylthiazole derivatives (6a–6l) showed higher 5-LOX inhibitory activity than 4-methylthiazole derivatives (7a–7c). Furthermore, substitution at the para position of the phenyl moiety at position 4 of thiazole with lipophilic bromo halogen (6e–6h) resulted in increased 5-LOX inhibitory activity over unsubstituted derivatives (6a–6d) and p-methoxy derivatives (6i–6l). In addition, substitution at position 3 of thiazole with lipophilic 2-chlorophenyl moiety (6b, 6f, and 6j) increased 5-LOX inhibitory activity within the same series.
3.2.3. In vivo anti-inflammatory activity
3.2.3.1. Formalin-induced paw edoema test (acute inflammation model)
Compounds 6a–l and 7a–c were subjected to in vivo anti-inflammatory activity ( and in Supp. File page S38-S39). Compounds (6a–f, 6i–l, 7a, 7c) showed higher % inhibition than celecoxib. Compounds 6a–c, 6e–f, 6i, j showed higher % inhibition than that of diclofenac sodium. Compounds 6b and 6j exhibited double % inhibition exhibited by celecoxib.
3.2.3.2. Gastric ulcerogenic activity
Compounds 6a–l and 7a–c were evaluated for their ulcerogenic potential in rats. Gross examination revealed that compounds 6a, 6b, 6f, 6h–l, and 7b showed a superior gastrointestinal safety profile (no ulceration) as the reference celecoxib in the population of fasted rats. On the other hand, compounds 6c–e, 6 g, 7a, and 7c showed variable degrees of hyperaemia (Supp. file page S40). In addition, histopathological examination was performed to confirm gross observations for the most active compounds (6b and 6f) as well as for references and control. Microscopic examination revealed that compounds 6b and 6f showed a superior gastrointestinal safety profile (no ulceration with normal gastric mucosa) as well as the reference celecoxib and DMSO. As regards diclofenac sodium, normal epithelium, and some congested blood vessels were observed ().
3.3. Molecular modeling
3.3.1. Molecular docking
The molecular modelling studies were performed using the Molecular Operating Environment (MOE 2016.08) softwareCitation65 for compounds 6b and 6f using the crystal structure of COX-2 enzyme co-crystallized with celecoxib (PDB: 3LN1) and 5-LOX in complex with 4-[(2R,3S)-3-[(3,4-dihydroxyphenyl)methyl]-2-methylbutyl]benzene-1,2-diol (NDGA) (PDB: 6N2W). It is well-known that protein flexibility plays an important role in the protein–ligand recognition process. Induced fit docking considers both the ligand and active site residues flexibility in its calculations which increases the accuracy of predicted binding interactions and binding affinityCitation66,Citation67. In the present investigation, MOE induced fit docking was used as a docking method. This method continuously sample ligand-receptor conformations as the docking proceeds, allowing for a best fit of the ligand within a potential binding pocket. In addition, five different compound-receptor fit complexes were obtained for each compound, and the best score was chosen.
3.3.1.1 Induced fit docking inside COX-2 active site
Compounds 6b and 6f which showed dual in vitro COX-2/5-LOX inhibiting activity and the reference celecoxib were subjected to induced fit docking into COX-2 active site (PDB: 3LN1)Citation68 using Molecular Operating Environment (MOE) version 2016.0802 softwareCitation62. The docking solutions of the compounds 6b, 6f, and celecoxib ( and –Citation8) confirmed the potential activities against COX-2 as the modes of interaction of the best poses were comparable to the reference celecoxib. All the target compounds revealed hydrophobic interaction with the key amino acid Leu338, and polar interaction with the key amino acid Ser339Citation69 in the polar side pocket of COX-2 active site. Interaction with this small pocket’s amino acids is essential for the selective inhibition of COX-2 enzymeCitation69. In addition, 6b interacted by 2 hydrogen bonds between Cl and Val335, and between imino N and Arg106 (). Also, 6f interacted by hydrogen bond between imino N and Arg106 (). Moreover, the overlay of compounds 6b, 6f, and celecoxib inside the active site of COX-2 () revealed that the 3,4-diarylthiazole moiety of 6b and 6f was superposed on 1,5-diarylpyrazole moiety of celecoxib with p-substitutedphenyl at position 4 of thiazole interacted at the same position of tolyl moiety of celecoxib. Besides, the substitutedphenyl at position 3 of thiazole interacted at the same position of benzenesulfonamide moiety of celecoxib.
Figure 5. Left: co-crystallized celecoxib, ligand-enzyme interaction (2D). Right: Overlay of co-crystallized celecoxib (yellow) and docked celecoxib (cyan) with RMSD = 0.422 (3D) inside COX-2 active site.
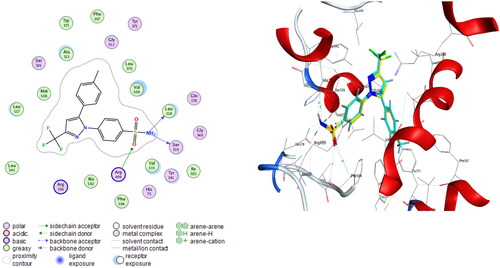
Table 2. Induced fit docking results of the active compounds inside COX-2 active site.
3.3.1.2. Induced fit docking to 5-LOX active site
Compounds 6b and 6f which showed dual in vitro COX-2/5-LOX inhibiting activity and co-crystallized ligand 4-[(2R,3S)-3-[(3,4-dihydroxyphenyl)methyl]-2-methylbutyl]benzene-1,2-diol (NDGA) were subjected to induced fit docking into 5-LOX active site (PDB: 6N2W)Citation70 using Molecular Operating Environment (MOE) version 2016.0802 softwareCitation62. The docking solutions of compounds 6b, 6f, and NDGA ( and –Citation13) supported the potential activities against 5-LOX. It is worth mentioning that, compounds 6b and 6f interacted with all residues interacted with NDGA (–Citation12). Furthermore, 5-LOX active site was reported to have several anchors. Polar positively-charged anchor consists of His550, His367, and His372 which interact with Fe2+. Polar anchor includes Asp176, Asn180, and Gln363 which interact by both electrostatic and hydrogen-bonding. Besides, Phe177, Leu607, Ile673, Leu414, Phe421, Gly174, Val175, Leu368, and Ile406 were considered hydrophobic anchor Citation71. Compounds 6b and 6f interacted with various anchors inside 5-LOX active site. All of them showed polar interaction with the His367 and His372 as positively charged anchors in addition to interaction with the key ferrous ion FE701. Besides, all compounds elicited polar interaction with Gln363 as part of the second polar anchor. Furthermore, all compounds elicited hydrophobic interaction with Leu368, Ile406, Leu414, Leu607, and Ile673 as part of the hydrophobic anchor. Moreover, the overlay of compounds 6b, 6f, and NDGA inside 5-LOX active site () predicted that, thymol moieties of both 6b and 6f were superposed on the first 3,4-dihydroxyphenyl moiety of NDGA. Furthermore, the substituted phenyl moieties at position 4 of thiazole of both 6b and 6f were superposed on the second 3,4-dihydroxyphenyl moiety of NDGA. This result confirmed the importance of the substituted phenyl moiety at position 4 of thiazole in the interaction with 5-LOX active site. In conclusion, the target compounds revealed binding score and fitting to 5-LOX active site better than NDGA. In addition, induced fit docking of 6b and 6f predicted additional polar interaction with the key ferrous ion FE701 which was not found with NDGA. So, the targets 6b and 6f could have a favourable binding affinity towards 5-LOX more than NDGA.
Figure 10. Overlay of NDGA (cyan) and docked NDGA (yellow) inside 5-LOX active site with RMSD = 1.2.
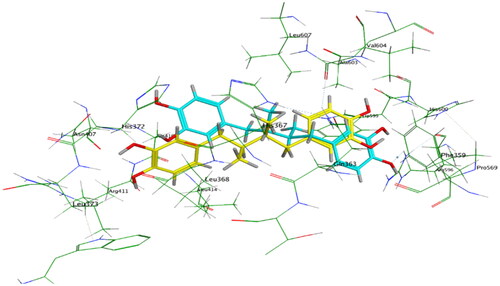
Table 3. Induced fit docking results of the active compounds in 5-LOX active site.
3.3.2. Molecular dynamics simulation
3.3.2.1. Molecular dynamics simulation of COX-2–ligand complexes
3.3.2.1.1. Root mean square deviation (RMSD)
RMSD plot for the backbone of apo protein and complex structures with the best docked 6b and 6f conformers were calculated (). It was observed that, after 10 ns, 6b and 6f complexes together with apo structure attained a stable state with non-significant change in RMSD. This indicated that, the complexes between compounds 6b and 6f with COX-2 retained dynamic conformational stability of the apo protein backbone. Furthermore, RMSD of 6b and 6f ligands were calculated (Supp. file page S41). 6b revealed neglected deviation from the induced docking conformer throughout the time of the test while 6f showed non-significant change in RMSD after 10 ns. These results confirmed the good fitting of the inhibitors 6b and 6f inside COX-2 active site.
3.3.2.1.2. Root mean square fluctuation (RMSF)
The interaction residues in COX-2 active site included three parts: part I for residues 335–345, part II residues 367–373, and part III residues 504–516. RMSF predicted that, non-significant variations of apo, 6b and 6f at the interaction residues (part I, II, and III) were noticed (, Supp. file page S42). This means that, the interaction between 6b and 6f ligands with the COX-2 active site residues formed stable and low energy complexes due to good fitting inside the active site.
3.3.2.1.3. Radius of gyration analysis (Rg)
Apo protein, 6b and 6f complexes Rg values predicted non-significant variations, and after 70000 ps all showed nearly the same Rg. This means that the binding of 6b and 6f with COX-2 did not change the packing and globularity of the apo protein. In addition, all complexes were compact and stable along with the apo throughout the simulation ().
3.3.2.1.4. Hydrogen bond analysis
There was not much considerable change in the hydrogen bond interaction within the protein of all the complexes. The average values of hydrogen bonds were nearly constant after 15 ns for all complexes (Supp. file page S42). Furthermore, the number of hydrogen bonds was calculated within 0.35 nm between protein and ligand complex structures. 6b complex predicted a maximum number of 7 hydrogen bond pairs after 45 ns, while the 6f complex was 6 pairs after 50 ns (). This indicated that, 6b and 6f were able to form hydrogen bonds with a high frequency of occurring a hydrogen bond.
3.3.2.1.5. Solvent-accessible surface area (SASA) calculations
The interaction area between the solvent and protein/protein–ligand complexes was calculated. The mean values for apo, 6b and 6f complexes were 254.23 nm2, 253.84 nm2, and 259.55 nm2. Higher value of SASA implies more hydrophilicity. The SASA value for 6b complex was nearly equal to apo while the 6f complex was more accessible to the solvent than apo and 6b complex (Supp. file page S42). The SASA did not show a significant change between complex structures in comparison with apo protein.
3.3.2.2. Molecular dynamics simulations of 5-LOX–ligand complexes
3.3.2.2.1. Root mean square deviation (RMSD)
RMSD plot for the backbone of apo protein and complex structures with the best docked 6b and 6f conformers were calculated (). It was observed that, after 30 ns, 6b and 6f complexes together with apo structure attained a stable state with non-significant change in RMSD. This indicated that the complexes between compounds 6b and 6f with 5-LOX retained dynamic conformational stability of the apo protein backbone. Furthermore, RMSD of 6b and 6f ligands were calculated (Supp. file page S43). 6b revealed stability with neglected deviation from the induced docking conformer after 30 ns of simulation while 6f showed a non-significant change in RMSD after 5 ns till the end of the simulation. These results confirmed the good fitting of the inhibitors 6b and 6f inside 5-LOX active site.
3.3.2.2.2. Root mean square fluctuation (RMSF)
The interaction residues in 5-LOX active site included three parts: part I for residues 359–376, part II residues 406–414, and part III residues 596–607. RMSF predicted that, non-significant variations of apo, 6b and 6f at the interaction residues (part I, II, and III) were noticed (, Supp. file page S43). This means that, the interaction between 6b and 6f ligands with the 5-LOX active site residues formed stable and low energy complexes due to good fitting inside the active site.
3.3.2.2.3. Radius of gyration analysis (Rg)
Apo protein, 6b and 6f complexes Rg values predicted non-significant variations after 20 ns. 6b complex was slightly more compact than apo and 6f complex. This means that the binding of 6b and 6f with 5-LOX did not significantly change the packing and globularity of the apo protein. In addition, all complexes were compact and stable along with the apo throughout the simulation ().
3.3.2.2.4. Hydrogen bond analysis
There was not much considerable change in the hydrogen bond interaction within the protein of all the complexes. The average values of hydrogen bonds were nearly constant after 10 ns for all complexes (Supp. file page S43). Furthermore, the number of hydrogen bonds was calculated within 0.35 nm between protein and ligand complex structures. 6b complex predicted a maximum number of 6 hydrogen bond pairs, while 6f complex was 5 pairs (). This indicated that 6b and 6f were able to form hydrogen bonds with 5-LOX active site and a high frequency of occurrence of a hydrogen bond.
3.3.2.2.5. Solvent-accessible surface area (SASA) calculations
The interaction area between the solvent and protein/protein–ligand complexes was calculated. The mean values for apo, 6b, and 6f complexes were 283.31 nm2, 286.42 nm2, and 290.55 nm2. A higher value of SASA implies more hydrophilicity. The SASA value for 6b and 6f complexes were higher than apo which means that 6b and 6f complexes were more accessible to the solvent than apo (Supp. file page S44). The SASA did not show a significant change between complex structures in comparison with apo protein.
3.4. Structure–activity relationship
The in vitro COX-1, COX-2, and 5-LOX inhibitory activities, and in vivo anti-inflammatory activity of the target compounds showed that substitution at position 4 of thiazole with aryl moiety is essential for activity as all 6a–l compounds were more active than the methyl derivatives (7a-c). Furthermore, the type of para substitution on the phenyl moiety at position 4 of thiazole greatly affected COX-2 and 5-LOX inhibitory activities. The para substitution with lipophilic electron withdrawing bromo derivatives (6e–6h) showed the highest dual COX-2/5-LOX inhibitory activities followed by the unsubstituted derivatives (6a–6d) while substitution with polar electron-donating methoxy group (6i–6 l) decreased COX-2 inhibitory activity, and retained 5-LOX inhibitory activity as unsubstituted derivatives. On the other hand, the type of substitution on the aryl moiety at position 3 of thiazole slightly affected the activity. The most active were the derivatives which contained the lipophilic electron-withdrawing 2-chloro substitution (6b and 6f) followed by the unsubstituted phenyl derivatives (6a and 6e) and the 2-pyridyl derivatives (6c and 6 g). In conclusion, the presence of aromatic moiety substituted with electron withdrawing lipophilic halogen at both positions three and four of thiazole had a great effect on COX-2 and 5-LOX inhibitory activities. This could be due to the hydrophobic interaction with the lipophilic anchors in both COX-2 and 5-LOX active sites.
4. Conclusion
There is an urgent need for new safe anti-inflammatory drugs lake the side effects of selective inhibition of COX-2 alone. Consequently, simultaneous inhibition of COX-2 and 5-LOX enzymes could reduce the side effects of anti-inflammatory candidates. In the present investigation, 3,4-disubstitutedthiazole pharmacophore, a bioisostere to the COX-2 inhibitor pharmacophore 1,5-diarylpyrazole, was hybridised with thymol, a natural 5-LOX inhibitor, aiming to act as dual COX-2/5-LOX inhibitors to produce in vivo active anti-inflammatory compounds with better safety profile and fewer side effects. Compounds 6b, 6d, 6e, and 6f displayed in vitro inhibitory activity against COX-2 (IC50= 0.037, 0.042, 0.046, and 0.039 µM) nearly equal to celecoxib (IC50= 0.045 µM). Besides, 6b, 6d, and 6f showed SI (379, 341, and 374, respectively) higher than that of celecoxib (327). Moreover, 6a–l elicited in vitro 5-LOX inhibitory activity higher than reference quercetin. On the other hand, 6a–f, 6i–l, 7a, and 7c possessed in vivo inhibition of formalin induced paw edoema higher than celecoxib. In addition, 6a, 6b, 6f, 6h–l, and 7b showed a superior gastrointestinal safety profile (no ulceration) as celecoxib and diclofenac sodium in the population of fasted rats. Furthermore, induced fit docking together with molecular dynamics simulation of COX-2 and 5-LOX – ligand complexes and apo protein were calculated. RMSD results confirmed the good fitting of the inhibitors 6b and 6f inside both COX-2 and 5-LOX active sites. Moreover, RMSF results predicted that the interaction between 6b and 6f ligands and both COX-2 and 5-LOX active sites’ residues formed stable and low energy complexes due to good fitting. Besides, Rg anticipated that, binding of 6b and 6f with both COX-2 and 5-LOX active sites did not change the packing and globularity of the apo protein, and all complexes were compact and stable. Furthermore, H-bond analysis concluded that 6b and 6f were able to form hydrogen bonds with a high frequency of occurrence. On the other hand, SASA did not show the significant change between complex structures in comparison with apo protein. In conclusion, 6b and 6f elicited multitarget inhibition of inflammation by in vitro simultaneous inhibition of COX-2 and 5-LOX higher than celecoxib and quercetin, in vivo potent anti-inflammatory activity higher than celecoxib, and in vivo gastrointestinal safety profile as celecoxib.
Author contributions
The manuscript was written through the contributions of all authors. All authors have given approval to the final version of the manuscript. All authors contributed equally.
Acknowledgement
Ahmed K. Al-Kubeisi would like to thank Alnukhba University College for their free support.
References
- Martel-Pelletier J, Lajeunesse D, Reboul P, Pelletier JP. Therapeutic role of dual inhibitors of 5-lox and cox, selective and non-selective non-steroidal anti-inflammatory drugs. Ann Rheum Dis. 2003;62(6):501–509.
- Garavito RM, Malkowski MG, DeWitt DL. The structures of prostaglandin endoperoxide h synthases-1 and-2. Prostagland Other Lipid Mediat. 2002;68-69:129–152.
- Zarghi A, Arfaei S. Selective cox-2 inhibitors: a review of their structure-activity relationships. Iran J Pharm Res. 2011;10(4):655–683.
- Konturek P, Kania J, Burnat G, Hahn E, Konturek S. Prostaglandins as mediators of cox-2 derived carcinogenesis in gastrointestinal tract. J Physiol Pharmacol. 2005;56(Suppl 5):57–73.
- Williams CS, DuBOIS RN. Prostaglandin endoperoxide synthase: why two isoforms? Am J Physiol. 1996;270(3 Pt 1):G393–G400.
- Jouzeau J-Y, Terlain B, Abid A, Nédélec E, Netter P. Cyclo-oxygenase isoenzymes: how recent findings affect thinking about nonsteroidal anti-inflammatory drugs. Drugs. 1997;53(4):563–582.
- Fu J-Y, Masferrer J, Seibert K, Raz A, Needleman P. The induction and suppression of prostaglandin h2 synthase (cyclooxygenase) in human monocytes. J Biol Chem. 1990;265(28):16737–16740.
- Maślińska D, Kaliszek A, Opertowska J, Toborowicz J, Deregowski K, Szukiewicz D. Constitutive expression of cyclooxygenase-2 (cox-2) in developing brain. A. Choroid plexus in human fetuses. Folia Neuropathologica. 1999;37(4):287–291.
- Kömhoff M, Wang J-L, Cheng H-F, Langenbach R, Mckanna JA, Harris RC, Breyer MD. Cyclooxygenase-2–selective inhibitors impair glomerulogenesis and renal cortical development. Kidney Int. 2000;57(2):414–422.
- Charlier C, Michaux C. Dual inhibition of cyclooxygenase-2 (cox-2) and 5-lipoxygenase (5-lox) as a new strategy to provide safer non-steroidal anti-inflammatory drugs. Eur J Med Chem. 2003;38(7-8):645–659.
- Young RN. Inhibitors of 5-lipoxygenase: a therapeutic potential yet to be fully realized? Eur J Med Chem. 1999;34(9):671–685.
- Lamie PF, Ali WAM, Bazgier V, Rárová L. Novel n-substituted indole schiff bases as dual inhibitors of cyclooxygenase-2 and 5-lipoxygenase enzymes: synthesis, biological activities in vitro and docking study. Eur J Med Chem. 2016;123803–813.
- Rainsford K. The effects of 5-lipoxygenase inhibitors and leukotriene antagonists on the development of gastric lesions induced by nonsteroidal antiinflammatory drugs in mice. Agents Actions. 1987;21(3-4):316–319.
- Rainsford K. Leukotrienes in the pathogenesis of nsaid-induced gastric and intestinal mucosal damage. Agents Actions. 1993;39 Spec No (S1):C24–C26.
- Laufer S. Discovery and development of ml3000. Inflammopharmacology. 2001;9(1-2):101–112.
- Hudson N, Balsitis M, Everitt S, Hawkey C. Enhanced gastric mucosal leukotriene b4 synthesis in patients taking non-steroidal anti-inflammatory drugs. Gut. 1993;34(6):742–747.
- Burnett BP, Levy RM. 5-lipoxygenase metabolic contributions to nsaid-induced organ toxicity. Adv Ther. 2012;29(2):79–98.
- Sala A, Zarini S, Bolla M. Leukotrienes: lipid bioeffectors of inflammatory reactions. Biochemistry-New York-English Translation of Biokhimiya. 1998;63(1):84–92.
- Sala A, Zarini S, Bolla M. Leukotrienes: Lipid bioeffectors of inflammatory reactions. Biochemistry (Mosc)). 1998;63(1):84–92.
- Hallstrand TS, Henderson WR. An update on the role of leukotrienes in asthma. Curr Opin Allergy Clin Immunol. 2010;10(1):60–66.
- Rossi A, Cuzzocrea S, Sautebin L. Involvement of leukotriene pathway in the pathogenesis of ischemia-reperfusion injury and septic and non-septic shock. Curr Vasc Pharmacol. 2009;7(2):185–197.
- Wang D, DuBois RN. Eicosanoids and cancer. Nat Rev Cancer. 2010;10(3):181–193.
- Bäck M. Leukotriene signaling in atherosclerosis and ischemia. Cardiovasc Drugs Ther. 2009;23(1):41–48.
- Yoshimura H, Sekine S, Adachi H, Uematsu Y, Mitani A, Futaki N, Shimizu N. High levels of human recombinant cyclooxygenase-1 expression in mammalian cells using a novel gene amplification method. Protein Expr Purif. 2011;80(1):41–46.
- Yao B, Xu J, Harris RC, Zhang M-Z. Renal localization and regulation of 15-hydroxyprostaglandin dehydrogenase. Am J Physiol Renal Physiol. 2008;294(2):433–439.
- Haruna H, Shimizu T, Ohtsuka Y, Yarita Y, Fujii T, Kudo T, Yamashiro Y. Expression of cox-1, cox-2, and ppar-γ in the gastric mucosa of children with helicobacter pylori infection. Pediatr Int. 2008;50(1):1–6.
- Yoshida S, Ujiki M, Ding X-Z, Pelham C, Talamonti MS, Bell RH, Denham W, Adrian TE. Pancreatic stellate cells (pscs) express cyclooxygenase-2 (cox-2) and pancreatic cancer stimulates cox-2 in pscs. Mol Cancer. 2005;4(1):27.
- Abdelall EKA, Kamel GM. Synthesis of new thiazolo-celecoxib analogues as dual cyclooxygenase-2/15-lipoxygenase inhibitors: determination of regio-specific different pyrazole cyclization by 2d nmr. Eur J Med Chem. 2016;118(:250–258.
- Garcia-Rodriguez LA, Hernández-Diaz S. Nonsteroidal antiinflammatory drugs as a trigger of clinical heart failure. Epidemiology. 2003;14:240–246.
- Ruan CH, So SP, Ruan KH. Inducible cox-2 dominates over cox-1 in prostacyclin biosynthesis: Mechanisms of cox-2 inhibitor risk to heart disease. Life Sci. 2011;88(1-2):24–30.
- Khan M, Fraser A. Cox-2 inhibitors and the risk of cardiovascular thrombotic events. Ir Med J. 2012;105(4):119–121.
- Singh P, Prasher P, Dhillon P, Bhatti R. Indole based peptidomimetics as anti-inflammatory and anti-hyperalgesic agents: Dual inhibition of 5-lox and cox-2 enzymes. Eur J Med Chem. 2015;97:104–123.
- Tries S, Neupert W, Laufer S. The mechanism of action of the new antiinflammatory compound ml3000: Inhibition of 5-lox and cox-1/2. Inflamm Res. 2002;51(3):135–143.
- Alvaro-Gracia JM. Licofelone—clinical update on a novel lox/cox inhibitor for the treatment of osteoarthritis. Rheumatology (Oxford)). 2004;43 Suppl 1 (90001):i21–i25.
- Philoppes JN, Abdelgawad MA, Abourehab MAS, Sebak M,A, Darwish M, Lamie PF. Novel n-methylsulfonyl-indole derivatives: biological activity and cox-2/5-lox inhibitory effect with improved gastro protective profile and reduced cardio vascular risks. J Enzyme Inhib Med Chem. 2023;38(1):246–266.
- Botelho MA, Barros G, Queiroz DB, Carvalho CF, Gouvea J, Patrus L, Bannet M, Patrus D, Rego A, Silva I, et al. Nanotechnology in phytotherapy: antiinflammatory effect of a nanostructured thymol gel from lippia sidoides in acute periodontitis in rats. Phytother Res. 2016;30(1):152–159.
- Marsik P, Kokoska L, Landa P, Nepovim A, Soudek P, Vanek T. In vitro inhibitory effects of thymol and quinones of nigella sativa seeds on cyclooxygenase-1- and -2-catalyzed prostaglandin e2 biosyntheses. Planta Med. 2005;71(8):739–742.
- Meeran N, Fizur M, Javed H, Al Taee H, Azimullah S, Ojha SK. Pharmacological properties and molecular mechanisms of thymol: Prospects for its therapeutic potential and pharmaceutical development. Front Pharmacol. 2017;8(:380.
- Tsai M-L, Lin C-C, Lin W-C, Yang C-H. Antimicrobial, antioxidant, and anti-inflammatory activities of essential oils from five selected herbs. Biosci Biotechnol Biochem. 2011;75(10):1977–1983.
- El-Miligy MMM, Al-Kubeisi AK, Bekhit MG, El-Zemity SR, Nassra RA, Hazzaa AA. Towards safer anti-inflammatory therapy: Synthesis of new thymol–pyrazole hybrids as dual cox-2/5-lox inhibitors. J Enzyme Inhib Med Chem. 2023;38(1):294–308.
- El-Miligy MMM, Al-Kubeisi AK, El-Zemity SR, Nassra RA, Abu-Serie MM, Hazzaa AA. Discovery of small molecule acting as multitarget inhibitor of colorectal cancer by simultaneous blocking of the key cox-2, 5-lox and pim-1 kinase enzymes. Bioorg Chem. 2021;115:105171.
- Che X-H, Chen C-L, Ye X-L, Weng G-B, Guo X-Z, Yu W-Y, Tao J, Chen Y-C, Chen X. Dual inhibition of cox-2/5-lox blocks colon cancer proliferation, migration and invasion in vitro. Oncol Rep. 2016;35(3):1680–1688.
- Sinha S, Sravanthi T, Yuvaraj S, Manju S, Doble M. 2-amino-4-aryl thiazole: a promising scaffold identified as a potent 5-lox inhibitor. RSC Adv. 2016;6(23):19271–19279.
- Apostolidis I, Liaras K, Geronikaki A, Hadjipavlou-Litina D, Gavalas A, Soković M, Glamočlija J, Ćirić A. Synthesis and biological evaluation of some 5-arylidene-2-(1, 3-thiazol-2-ylimino)-1, 3-thiazolidin-4-ones as dual anti-inflammatory/antimicrobial agents. Bioorg Med Chem. 2013;21(2):532–539.
- Jacob P J, Manju SL. Identification and development of thiazole leads as cox-2/5-lox inhibitors through in-vitro and in-vivo biological evaluation for anti-inflammatory activity. Bioorg Chem. 2020;100(:103882.
- P JJ, S L M. Novel approach of multi-targeted thiazoles and thiazolidenes toward anti-inflammatory and anticancer therapy—dual inhibition of cox-2 and 5-lox enzymes. Med Chem Res. 2021;30(1):236–257.
- Rajput J, Bagul S, Tadavi S, Karandikar P, Bendre R. Design, synthesis and biological evaluation of novel class diindolyl methanes (dims) derived from naturally occurring phenolic monoterpenoids. Med Chem. 2016;6(2):123–128.
- Duff JC. 96. A new general method for the preparation of o-hydroxyaldehydes from phenols and hexamethylenetetramine. J Chem Soc. 1941:547–550.
- Casiraghi G, Casnati G, Cornia M, Pochini A, Puglia G, Sartori G, Ungaro R. Selective reactions using metal phenoxides. Part 1. Reactions with formaldehyde. J Chem Soc, Perkin Trans 1. 1978;4(4):318–321.):
- Casnati G, Casiraghi G, Puglia G, Sartori G, Terenghi G. Process for preparing 2-hydroxybenzoic aldehydes. In. Process for preparing 2-hydroxybenzoic aldehydes: Google Patents; 1979.
- Yousef TA, Badria FA, Ghazy SE, El-Gammal OA, El-Reash GMA. In vitro and in vivo antitumor activity of some synthesized 4-(2-pyridyl)-3-thiosemicarbazides derivatives. Int J Med Med Sci. 2011;3(2):37–46.
- Serra S, Moineaux L, Vancraeynest C, Masereel B, Wouters J, Pochet L, Frédérick R. Thiosemicarbazide, a fragment with promising indolamine-2, 3-dioxygenase (ido) inhibition properties. Eur J Med Chem. 2014;82(:96–105.
- Betz R, McCleland C, Marchand H. 2-bromo-1-phenylethanone. Acta Crystallogr Sect E Struct Rep Online. 2011;67(Pt 5):o1207–o1207.
- Tada N, Ban K, Hirashima S-i, Miura T, Itoh A. Direct synthesis of α-bromoketones from alkylarenes by aerobic visible light photooxidation. Org Biomol Chem. 2010;8(20):4701–4704.
- Zhang J, Zhuang L-h, Wang G-w. 2-bromo-1-(4-methoxyphenyl) ethanone. Acta Crystallogr Sect E Struct Rep Online. 2009;65(Pt 9):o2245.
- Cox inhibitor screening assay kit. No. 560131.
- Cayman C. Lipoxygenase inhibitor screening assay kit 760700..
- Razmi A, Zarghi A, Arfaee S, Naderi N, Faizi M. Evaluation of anti-nociceptive and anti-inflammatory activities of novel chalcone derivatives. Iran J Pharm Res. 2013;12(Suppl):153–159.
- Lakshmi V, Mishra V, Palit G. A new gastroprotective effect of limonoid compounds xyloccensins x and y from xylocarpus molluccensis in rats. Nat Prod Bioprospect. 2014;4(5):277–283.
- Hazzaa AA-E. Synthesis and biological evaluation of novel terpene derivatives. AlexU-IACUC (Member of ICLAS) 2019.
- Srivastava S, Nath C, Gupta M, Vrat S, Sinha J, Dhawan K, Gupta G. Protection against gastric ulcer by verapamil. Pharmacol Res. 1991;23(1):81–86.
- Molecular operating environment (moe) 2016.08, chemical computing group inc. 1010 sherbrooke st. West, suite #910, montreal, qc, canada, h3a2r7. www.Chemcomp.Com.
- Abraham MJ, Murtola T, Schulz R, Páll S, Smith JC, Hess B, Lindahl E. Gromacs: High performance molecular simulations through multi-level parallelism from laptops to supercomputers. SoftwareX. 2015;1-2:19–25.
- https://simlab.Uams.Edu/.
- Bullock AN, Russo S, Amos A, Pagano N, Bregman H, Debreczeni JE, Lee WH, von Delft F, Meggers E, Knapp S. Crystal structure of the pim2 kinase in complex with an organoruthenium inhibitor. Plos One. 2009;4(10):e7112.
- Sotriffer Accounting AC. for induced-fit effects in docking: What is possible and what is not? Curr Top Med Chem. 2011;11(2):179–191.
- Barreca ML, Iraci N, De Luca L, Chimirri A. Induced‐fit docking approach provides insight into the binding mode and mechanism of action of hiv‐1 integrase inhibitors. ChemMedChem Chem Enabl Drug Discov. 2009;4(9):1446–1456.
- Wang JL, Limburg D, Graneto MJ, Springer J, Hamper JRB, Liao S, Pawlitz JL, Kurumbail RG, Maziasz T, Talley JJ, et al. The novel benzopyran class of selective cyclooxygenase-2 inhibitors. Part 2: The second clinical candidate having a shorter and favorable human half-life. Bioorg Med Chem Lett. 2010;20(23):7159–7163.
- Kurumbail RG, Stevens AM, Gierse JK, McDonald JJ, Stegeman RA, Pak JY, Gildehaus D, Miyashiro JM, Penning TD, Seibert K, et al. Structural basis for selective inhibition of cyclooxygenase-2 by anti-inflammatory agents. Nature. 1996;384(6610):644–648.
- Gilbert NC, Rui Z, Neau DB, Waight MT, Bartlett SG, Boeglin WE, Brash AR, Newcomer ME. Conversion of human 5-lipoxygenase to a 15-lipoxygenase by a point mutation to mimic phosphorylation at serine-663. Faseb J. 2012;26(8):3222–3229.
- Hsu K-C, HuangFu W-C, Lin TE, Chao M-W, Sung T-Y, Chen Y-Y, Pan S-L, Lee J-C, Tzou S-C, Sun C-M, et al. A site-moiety map and virtual screening approach for discovery of novel 5-lox inhibitors. Sci Rep. 2020;10(1):10510.