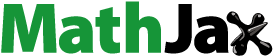
ABSTRACT
Introduction
Malaria, a devastating febrile illness caused by protozoan parasites, sickened 247,000,000 people in 2021 and killed 619,000, mostly children and pregnant women in sub-Saharan Africa. A highly effective vaccine is urgently needed, especially for Plasmodium falciparum (Pf), the deadliest human malaria parasite.
Areas covered
Sporozoites (SPZ), the parasite stage transmitted by Anopheles mosquitoes to humans, are the only vaccine immunogen achieving >90% efficacy against Pf infection. This review describes >30 clinical trials of PfSPZ vaccines in the U.S.A., Europe, Africa, and Asia, based on first-hand knowledge of the trials and PubMed searches of ‘sporozoites,’ ‘malaria,’ and ‘vaccines.’
Expert opinion
First generation (radiation-attenuated) PfSPZ vaccines are safe, well tolerated, 80–100% efficacious against homologous controlled human malaria infection (CHMI) and provide 18–19 months protection without boosting in Africa. Second generation chemo-attenuated PfSPZ are more potent, 100% efficacious against stringent heterologous (variant strain) CHMI, but require a co-administered drug, raising safety concerns. Third generation, late liver stage-arresting, replication competent (LARC), genetically-attenuated PfSPZ are expected to be both safe and highly efficacious. Overall, PfSPZ vaccines meet safety, tolerability, and efficacy requirements for protecting pregnant women and travelers exposed to Pf in Africa, with licensure for these populations possible within 5 years. Protecting children and mass vaccination programs to block transmission and eliminate malaria are long-term objectives.
1. Overview
This review is written by the developers of Plasmodium falciparum (Pf) sporozoite (SPZ) vaccines (vaccines comprised of live, metabolically active SPZ) and by researchers who have generated the published and unpublished clinical data described herein. It provides an historical account, explaining the rationale, objectives, and achievements to date, and also the challenges that need to be overcome on the pathway to licensure and deployment. The authors’ inside perspective allows the presentation of details including key development decisions that would not be available in a systematic review written from outside the field.
The main objective since the inception of development efforts has been to develop PfSPZ vaccines that are well tolerated, safe, and prevent Pf infection in >90% of recipients for at least 3 months against heterologousFootnote1 controlled human malaria infection (CHMI) and >90% for at least 2 years without boosting against genetically and antigenically diverse naturally transmitted malaria parasites in Africa. PfSPZ vaccines are thus aimed at preventing both infection and transmission, not only disease, meaning that they are designed to be deployed in mass vaccination programs (MVPs) to block transmission and eliminate malaria from defined geographic areas. PfSPZ vaccines have achieved the first target, >90% protection against heterologous CHMI. Field protection currently stands at about 50–60% vaccine efficacy against Pf infection, with the expectation that the newest PfSPZ platforms will improve this to the >90% target. Developers are concurrently working to increase manufacturing efficiency and reduce the cost of goods so the vaccines can be licensed and deployed for use in endemic areas and travelers to endemic areas.
Progress along this development pathway has required innovation in PfSPZ manufacturing, stabilization, route of administration, clinical trial assessment, and genetic manipulation of Pf parasites. Developers initially assessed PfSPZ Vaccine (Sanaria® PfSPZ Vaccine), the first generation, radiation-attenuated PfSPZ vaccine and found that it was well tolerated, safe, and induced 100% vaccine efficacy (VE) against homologousFootnote2 CHMI at 3–7 weeks after the last dose in the U.S.A., Tanzania, and Mali [Citation1–3], and sustained, but reduced VE against homologous CHMI at 14 months (VE 55%) [Citation4] and against heterologous CHMI at 8 months (VE 54%) [Citation5]. With development of an accelerated immunization regimen, similar VE (77–79%) was achieved against homologous and heterologous CHMI at 9–10 weeks [Citation6]. PfSPZ Vaccine did not achieve the target of 90% protection against heterologous CHMI, however, but did achieve one major goal for VE in the field in Africa – protection for at least 2 transmission seasons, with VE of 50–60% ([Citation7] and Diawara unpublished [NCT03989102]).
Developers progressed to PfSPZ-CVac (Sanaria® PfSPZ-CVac), the second generation, chemo-attenuated PfSPZ vaccine, and found that it induced 100% VE against heterologous CHMI at 3 months after the last dose, and did so at 22% the dose needed for PfSPZ Vaccine [Citation8]. This more potent PfSPZ platform has not yet been assessed adequately for durable VE in the field. However, depending on the partner drug used for chemo-attenuation, PfSPZ-CVac may be associated with 1–2 days of side effects 1 week after the first dose because of transient parasitemia, and has inherent, potential safety issues, because it includes administration of infectious PfSPZ requiring a co-administered drug for in vivo attenuation.
To achieve the tolerability and safety of PfSPZ Vaccine and the VE and potency of PfSPZ-CVac, developers have created through deletion of two genes a late liver stage-arresting replication competent (LARC) genetically attenuated (GA) parasite, PfSPZ-LARC2 Vaccine (Sanaria® PfSPZ-LARC2 Vaccine). This has been manufactured under current Good Manufacturing Practices (cGMPs) and will be assessed in clinical trials in 2023-2024.
The short-term goal of PfSPZ vaccine development is to achieve licensure for prevention of malaria in women of childbearing potential who become pregnant and in travelers from malaria non-endemic areas to endemic areas, especially in Africa. Extending licensure to children is a mid-term goal. The long-term goal is to use a PfSPZ vaccine in malaria elimination campaigns, and to that end Sanaria is developing a new, cGMP-compliant, in vitro method of manufacturing that does not require mosquitoes and has the potential to reduce cost of goods by 90% and increase manufacturing efficiency by at least 10-fold [Citation9].
Progress in this development effort has been possible due to the work of collaborators from all over the world who participate via the International PfSPZ Consortium, which meets regularly to exchange data, set priorities and define next steps in development.
2. Background
Attenuated PfSPZ inoculated into humans in high numbers have long been known to induce sterileFootnote3 immunity to malaria, providing a strong foundation for vaccine development. Exploratory studies using avian malaria SPZ began more than 100 years ago [Citation10–15], with scientific momentum building in the late 1960s after the publication of research at New York University by Ruth Nussenzweig, Jerome Vanderberg, and colleagues showing sterile protection in mice after intravenous immunization with radiation-attenuated SPZ of the murine malaria parasite Plasmodium berghei (Pb) [Citation16]. In the 1970s, David Clyde, Vincent McCarthy, Jerome Vanderberg and colleagues at the University of Maryland and New York University [Citation17–21] and Karl Rieckmann, Richard Beaudoin, and colleagues at the Rush-Presbyterian-St. Luke’s Medical Center in Chicago and the Naval Medical Research Institute (NMRI) [Citation22–24], with later contributions from the Naval Medical Research Center and the Walter Reed Army Institute for Research [Citation25] demonstrated that immunization of humans with radiation-attenuated PfSPZ resulted in high-level protection. Irradiated, infected mosquitoes were used to inoculate the PfSPZ, and after 1,000 or more infectious bites, 92.8% of malaria-naive humans were protected against subsequent CHMI with homologous and heterologous strain parasites. Protection was durable against a second CHMI for at least 10 months and in one research participant boosted 2 weeks beforehand, for 5 years [Citation25].
Investigators also showed that radiation-attenuated SPZ vaccines protected non-human primates against Plasmodium knowlesi, P. cynomolgi and P. vivax [Citation26–28]. These models, along with the rodent models, have permitted mechanistic investigations. These have demonstrated that T cells and a CD8 T cell clone from mice immunized with radiation-attenuated SPZ eliminated infected hepatocytes from culture [Citation29,Citation30], that adoptive transfer of CD8 T cells and T cell clones from a protected to a naive animal conferred sterile immunity to the recipient [Citation31–36], and that protection was lost with CD8 T cell depletion [Citation35,Citation37–42]. Interferon-gamma (IFN-γ) production was essential to protective immunity and likely mediated the elimination of infected hepatocytes through induction of nitric oxide [Citation39]. CD4 T cells were important to the induction and maintenance of protective immunity but not critical in most strains of mice for the effector phase of protection [Citation43–46]. Antibodies, including antibodies to the circumsporozoite protein (CSP, the outer coat of sporozoites) contributed to protection through neutralization of SPZ but were not adequate on their own in the radiation-attenuation model to mediate protection in the absence of CD8 T cells [Citation35,Citation37]. Cytotoxic T cells from humans immunized by mosquito bite with radiation-attenuated PfSPZ recognized several Pf antigens [Citation47–50], and cytotoxic CD8 T cells and IFN-γ produced by these and other cells like NK cells in the liver likely underlie protection in humans as they do in animal models [Citation51]. Many studies have pointed to the importance of liver-resident, patrolling memory CD8 T cells for protection against malaria [Citation52–56] including the maintenance of long-term memory [Citation57,Citation58]. These cells are not easily accessed in humans, however, and the ability of circulating T cell populations to serve as a proxy for liver-resident cell populations remains unclear. For these reasons, the primary effector mechanisms in humans still await clear definition.
Since the proteome of liver stage parasites includes the products of at least 4000 genes [Citation59,Citation60], CD8 T cell epitopes from thousands of antigens could be cross-presented by antigen presenting cells and prime antigen-specific CD8 T cells in the liver-draining lymph nodes at the time of first PfSPZ immunization. As the vaccine recipient progresses from their first to subsequent PfSPZ immunizations, the diversity and biomass of antigens produced by each dose of vaccine-stage sporozoites and the attenuated liver stage parasites into which they develop, are likely diminished by accruing adaptive immune responses [Citation61], which makes differentiating protective responses from immunogenic responses more difficult. When malaria is later transmitted to the vaccinated individual, a subset of the immunogenic antigens expressed on the surface of infected hepatocytes bound to MHC-I molecules could serve as the target of protective T cells leading to the killing of parasite-infected hepatocytes or the elimination of the parasite within the infected cells [Citation62]. To date, however, the protective antigen repertoire has not been identified, motivating an ongoing effort to use reagents from individuals immunized with PfSPZ for antigen discovery [Citation50,Citation63,Citation64]. The role of T cells, particularly the Vδ2 subset [Citation4,Citation65–69], and of NK cells [Citation70,Citation71] in the induction of protective immunity by PfSPZ vaccines are important areas of ongoing investigation. A reappraisal of the role of antibodies is also underway [Citation72–74], with the tools of systems serology offering an innovative approach to elucidate antibody-associated protective mechanisms following SPZ immunization [Citation72,Citation73].
The sterile protection of humans achieved with SPZ immunization is consistent with the generally high efficacy associated with using attenuated pathogens for vaccination, although unlike most acute infections for which there are successful vaccines, naturally acquired Plasmodium infection does not induce sufficient immunity to sterilely protect against subsequent infections [Citation75–78]. Efficacious live vaccines are licensed for 15 of the 31 pathogensFootnote4 for which vaccines are approved in the U.S.A. [Citation79], and vaccines based on inactivated pathogens are available for an additional six pathogensFootnote5 as well as for several pathogens for which both are licensed. Live attenuated vaccines present the immune system with multiple immunogens, which could range from tens of immunogens for viruses to hundreds or thousands with eukaryotic organisms such as Plasmodium. Typically, the pathogen carries stimulatory molecules that help in the development of immune memory. Development, licensure, and deployment of live vaccines can be done without knowing which antigens among those presented to the immune system induce protection. HLA backgrounds and other genetically variant or acquired immune characteristics add complexity, such that the protective responses may vary among individuals [Citation80]. Live attenuated vaccines are potent in part because replication increases the biomass of immunogens and may also increase their diversity, and adjuvants are generally not required. Protective immunity can last for decades (e.g. yellow fever vaccine may provide life-long protection [Citation81,Citation82]) and often only a single immunization is required. Live vaccines generally have good safety profiles, albeit some require an intact human immune system to curtail replication and are contraindicated in immunocompromised individuals.
The protection afforded by immunization with PfSPZ vaccines against Pf malaria in humans shares many characteristics with other live vaccines, including potency and durable protection, although radiation-attenuation prevents replication and the associated amplification of the immunogen, and several injections are needed. Sterile immunity has now been shown to last at least 14–28 months [Citation4,Citation83] in humans and as described above has been linked to memory CD4 and CD8 T cells in humans as in animal models [Citation84,Citation85]. Because SPZ are eukaryotic cells, the immunogen is larger than live bacterial or viral vaccine constructs and (via gliding motility) can traverse interstitial spaces and penetrate cells including endothelial cells, Kupffer cells, and hepatocytes (). The use of such large, GMP-produced, attenuated organisms as vaccine immunogens is a new undertaking in human infectious disease vaccinology.
Figure 1. Malaria sporozoite. Scanning electron micrograph of a Plasmodium cynomolgi sporozoite superimposed on a scanning electron micrograph of a rat liver sinusoid. P. falciparum sporozoites are similar in appearance and are typically 8–10 microns in length. They are large eukaryotic cells able to glide along the endothelium, traverse interstitial spaces, and penetrate Kupffer cells and hepatocytes. Pc = parenchymal cell (hepatocyte); fsc = fat-storing cells (stellate cell); SD = space of Disse. Arrows point to endothelial cell fenestrations. This composite image was made by Ute Frevert, who modified the shape of the sporozoite to better reflect the ability of the parasite to flex when gliding along natural tissue structures as shown by intravital cinematography. Reproduced with permission from Comparative Hepatology (no changes were made) [Citation86].
![Figure 1. Malaria sporozoite. Scanning electron micrograph of a Plasmodium cynomolgi sporozoite superimposed on a scanning electron micrograph of a rat liver sinusoid. P. falciparum sporozoites are similar in appearance and are typically 8–10 microns in length. They are large eukaryotic cells able to glide along the endothelium, traverse interstitial spaces, and penetrate Kupffer cells and hepatocytes. Pc = parenchymal cell (hepatocyte); fsc = fat-storing cells (stellate cell); SD = space of Disse. Arrows point to endothelial cell fenestrations. This composite image was made by Ute Frevert, who modified the shape of the sporozoite to better reflect the ability of the parasite to flex when gliding along natural tissue structures as shown by intravital cinematography. Reproduced with permission from Comparative Hepatology (no changes were made) [Citation86].](/cms/asset/5936d3ae-9ea1-4312-a455-aa5b189af927/ierv_a_2245890_f0001_b.gif)
2.1. Rationale for PfSPZ vaccines
Significant challenges confronted vaccinologists contemplating the development of this new vaccine platform based on PfSPZ [Citation87,Citation88]. These included development of methods for aseptic production (which depends upon aseptic mosquitoes as the ‘bioreactor’), purification from the salivary glands, cryostabilization in liquid nitrogen vapor phase (LNVP, a requirement for eukaryotic cells), distribution in LNVP, and optimized methods of immunization. It is useful to review the reasons that developers embarked on such an effort, as it could be argued that improving existing technologies such as recombinant malaria proteins in adjuvant, viral vectors engineered to express Pf transgenes, or DNA plasmid or mRNA platforms would be more straightforward. Of note, several authors of this review, including the first and last authors, spent decades working on these technologies, including the development and testing of the first recombinant Pf circumsporozoite protein (PfCSP)-based subunit vaccines [Citation89], the assessment of novel vaccine vectors [Citation90–92], the development of DNA plasmid [Citation93–95] and virally vectored gene-based [Citation96,Citation97] vaccines, and the first heterologous prime boost studies [Citation98,Citation99]. A DNA prime adenovirus vector boost approach achieved the best protection against CHMI ever recorded using gene-based technologies [Citation100], including the first demonstration in humans of a correlation between cellular immunity and protection against a parasite along with identification of the CD8 epitopes potentially mediating protection [Citation101,Citation102]. However, vaccine efficacy (VE) was low in this best-in-class result for gene-based approaches: only 4/15 (27%) participants were sterilely protected against homologous CHMI conducted 4 weeks after immunization. The prospect of achieving incremental improvements to reach >90% protection against heterologous CHMI at 12 weeks after immunization, the goal set by PfSPZ vaccine developers, appeared daunting.
While PfSPZ development has been underway, more traditional vaccine platforms have achieved significant successes in the fight against malaria. Thirty-seven years after the publication of the first trial of a subunit vaccine based on recombinant PfCSP in adjuvant [Citation89], the leading downstream candidates, RTS,S/AS01 and R21 have undergone Phase 3 testing. The World Health Organization (WHO) has recently endorsed the roll-out of RTS,S/AS01 (GSK’s Mosquirix®) in malaria-endemic countries, with a recommendation for heavily endemic countries to license the vaccine for use in 5- to 17-month-olds aiming to reduce the morbidity and mortality associated with P. falciparum malaria during early childhood. R21, developed by the Jenner Institute at the University of Oxford, has recently been licensed for a similar indication in Ghana [Citation103] and Nigeria [Citation104]. Both vaccines are expected to reduce the burden of disease and death caused by Pf. However, neither has achieved >90% protection against homologous CHMI, and neither has been tested against the rigor of CHMI using a heterologous challenge parasite. Protection achieved by RTS,S/AS01 has ranged from 55% to 87% against homologous CHMI at 3 weeks for the optimized, delayed-fractional dose regimen (3 doses over 7 months) [Citation105,Citation106], while that of R21 has ranged from 63% to 78% against homologous CHMI at 3 weeks (3 doses over 2 months) [Citation107]. In contrast, in the original mosquito bite studies, radiation-attenuated PfSPZ achieved 92.8% protection against homologous CHMI in humans when more than 1000 infectious bites were administered, with additional evidence for heterologous protection [Citation25], indicating a potential for high-level protection not achievable by other malaria vaccine approaches.
To the authors, achieving high efficacy after the first proof-of-principle studies provided a strong starting point, given the challenge of developing a vaccine against a pathogen proven adept at developing resistance to drugs and other interventions including evading detection by rapid diagnostic tests. PfSPZ offered diversity and breadth of immunogens, features likely needed to counteract resistance and to induce protective responses in all vaccine recipients despite the MHC-restriction characterizing cell-mediated immunity. In addition, the right PfSPZ platform (different from radiation-attenuated PfSPZ, which do not replicate) could potentially amplify and diversify important immunogens in vivo through replication and development. There was also a precedent in the veterinary field with a sporozoite vaccine widely distributed in East Africa for the prevention of East Coast Fever cause by the protozoan parasite Theileria parva [Citation108,Citation109]. Given that PfSPZ had already shown the intrinsic biological potential to induce high-level protection, even before any improvements had been made, the manufacturing and other challenges associated with a eukaryotic whole-cell vaccine appeared technical in nature, likely solvable through creative bioengineering (Box 1) and were not accepted as adequate reasons to forego development.
2.2. Principles guiding the development of PfSPZ vaccines
The guiding principles along the development pathway have been a systematic focus on achieving (1) high-level, >90% VE against CHMI with Pf strains that differ genetically and immunologically from the Pf strain in the vaccine (heterologous CHMI) [Citation110,Citation111], (2) durable protection against Pf infection with the heterogeneous Pf transmitted naturally in the field in Africa, (3) the improvements needed to repeatedly exceed prior levels of protection, and (4) safety. Two recent clinical trials have substantially advanced PfSPZ vaccines along this pathway toward a final product. A team at the Laboratory of Malaria Immunology and Vaccinology (LMIV) at the National Institute of Allergy and Infectious Diseases (NIAID) demonstrated that three doses of PfSPZ-CVac (chloroquine), the chemo-attenuated SPZ platform, gave 100% protection against CHMI conducted 12 weeks after immunization using a Pf parasite (Pf7G8) heterologous to the vaccine strain (PfNF54) and in fact more distant from the vaccine strain than any of 704 Pf isolates from all over Africa [Citation68,Citation111]. Although the sample size was small in this study group (6 research participants), this level of protection against a variant challenge strain had never been achieved before. In the second trial, the LMIV team in collaboration with a team from the Malaria Research and Training Center (MRTC) in Bamako, Mali, demonstrated that three doses of PfSPZ Vaccine (radiation-attenuated PfSPZ) administered over 4 weeks protected pregnant and non-pregnant Malian women against Pf infection and clinical malaria over two transmission seasons, without boosting [Citation112]. These demonstrations of protection that exceeded what was thought possible have changed perceptions and supported investment for ongoing development.
With the exception of efficacy and safety, developers largely eschewed target product profiles (TPPs) that mandate the selection of conventional vaccine platforms, routes of administration, dosing regimens, and storage conditions. TPPs are often applied by the vaccine industry at the start of development to channel efforts toward products with commercial viability. While aware of the risks of their decision, the developers of PfSPZ vaccines believed that it was appropriate to focus initially on proof-of-concept for high-level protection and safety no matter how impractical the approach. Issues of feasibility, such as how to scale up a manufacturing process using aseptic mosquitoes or how to distribute a vaccine stored in LNVP, were deprioritized. Simply put, the development of a highly protective vaccine against a parasite was viewed as such a formidable, societally relevant and technologically distinct undertaking compared to classical vaccine development for viral or bacterial diseases that existing models might need to be discarded to allow an entirely fresh start. The validity of this unconventional approach, with less initial attention to TPPs than the norm, involved considerable risk. Instead, feasibility issues have been pursued in parallel with vaccine testing, and 15 years into the development effort a degree of success has been achieved. For example, methods have been developed to automate PfSPZ extraction from mosquitoes [Citation149] and to produce PfSPZ in vitro in bioreactors [Citation9], advances that will be incorporated into manufacturing processes in the years ahead. In other words, now that safety and efficacy targets are being approached or met, the considerable expense and effort required to achieve downstream optimization of the PfSPZ platform can be more strongly justified to granting agencies, investors, and the developers themselves.
The body of this review describes the types of whole PfSPZ vaccines under development and highlights key insights, achievements, and next steps for each PfSPZ platform. Important challenges facing the further development of these vaccines are then described, including biological challenges such as the potential to achieve high-level VE in the field, and feasibility challenges such as scaling up manufacturing, distribution, and administration to target populations. Additional sections cover booster doses, SPZ immunization against Plasmodium vivax (Pv) (a second important human malaria parasite), plans for licensure and registration in the U.S.A., EU, and malaria-endemic countries, and applications for PfSPZ vaccination once registration is approved.
Box 1. Technology Spin-offs from Creative Bioengineering and Other Innovations
The innovative manufacturing processes for producing aseptic, purified, cryopreserved PfSPZ took Sanaria several years to develop before the first human trials. Since then, Sanaria has achieved increases in the efficiency and scale-up of manufacture of PfSPZ, greater potency of PfSPZ and reduction in cost-of-goods (COGs). Novel approaches have been extended to the manufacturing-clinical interface with the design of unique cryovials for vaccine storage and devices for vaccine thawing, and into the clinic, including the direct venous inoculation (DVI) route of administration. Breakthroughs have been achieved by International PfSPZ Consortium collaborators in the development of genetically altered parasites. A new series of innovations now at the preclinical research and development stage will enable next-generation vaccines to achieve further increases in potency and decreases in COGS as they enter the clinic in the next few years.
Innovations in Manufacturing, Vaccine Platforms, and Clinical Use
Manufacturing: Key innovations in manufacturing have supported the production of aseptic, purified, vialed, cryopreserved, live PfSPZ to meet the requirements for identity, strength, purity, and asepticity of the final vaccine product in accordance with ICH Q6A guidelines. These have included the mass production of aseptic mosquitoes and SPZ (US Pat. 8,802,919), purification and characterization of purity and asepticity of the vaccine (US Pat 8,043,625), stabilization of the product by cryopreservation, characterization of the potency and attenuation of PfSPZ [Citation1,Citation51,Citation113,Citation114], and development of methods for global distribution in a vapor phase liquid nitrogen cold chain independent of electricity [Citation115,Citation116]. All elements of Sanaria’s manufacturing process have proven amenable to scale up, independently and collectively, ensuring that the core platform technology can meet the manufacturing needs of the novel vaccine candidates as they move through the preclinical and clinical development pipeline.
PfSPZ Challenge: Aseptic, purified, vialed, cryopreserved, fully infectious PfSPZ, manufactured to GMP specifications (Sanaria® PfSPZ Challenge) can be used for CHMI. To determine the optimal route and dose of PfSPZ Challenge administration, Sanaria sponsored or supported seven studies in six countries (Netherlands, UK, Germany, Spain, Kenya, Tanzania) assessing eight different approaches to ID administration, 12 approaches to intramuscular (IM) administration, and six approaches to IV administration of PfSPZ Challenge (strain NF54) [Citation113,Citation114,Citation117–122], and two studies in two countries (U.S.A., Germany) assessing four approaches to IV administration of PfSPZ Challenge (clone 7G8) [Citation6,Citation123]. Since completing these optimization studies, PfSPZ Challenge (NF54) and PfSPZ Challenge (7G8) have been used in the U.S.A., five countries in Europe and six countries in Africa at multiple sites to conduct 124 CHMIs (44 of these in one trial). Among residents of the U.S.A. and Europe and Europeans recently moving to Africa but not yet infected with malaria, 81/81 (100%) of those injected by DVI with a 3,200 PfSPZ dose of PfSPZ Challenge (NF54) and 38/39 (97.4%) of those injected with the same dose of PfSPZ Challenge (7G8) became infected on first injection when serving as controls in clinical trials, providing a highly standardized method for CHMI. The infection rate has been lower and less predictable in African adults, varying from 100% in minimally malaria-exposed Tanzanian adults [Citation2,Citation124] to 53% in heavily malaria-exposed Malian adults [3], indicating that naturally acquired immunity decreases the infectivity of the PfSPZ. PfSPZ Challenge has been used to investigate the efficacy of anti-malaria vaccines [Citation2,Citation3,Citation6,Citation8,Citation68,Citation124–129] and drugs [Citation130,Citation131] and the complex elements of innate and acquired immunity to malaria [Citation132–135]. It has also simplified the operations of CHMI that previously required coordinating study participants’ availability, progress through a clinical trial, release of investigational product, and the production of infectious mosquitoes. PfSPZ Challenge is also the immunogen in PfSPZ-CVac.
Direct Venous Inoculation: IV administration, whether by pre-positioned catheter or by DVI has proven critical to both the efficacy of PfSPZ vaccines [Citation1,Citation8] and the infectivity of PfSPZ Challenge [Citation114]. Protection is lower by other routes of administration [Citation51,Citation122], and infection is less consistent and requires higher doses. Two concerns were originally voiced regarding DVI. The first concern related to safety: since the PfSPZ are isolated from mosquitoes, would DVI of PfSPZ lead to anaphylaxis due to mosquito-derived impurities administered with the PfSPZ? This has not been observed after 9,121 IV PfSPZ inoculations in 3,234 individuals aged 5 months to 61 years of age, a reflection of the high level of purity achieved through Sanaria’s manufacturing process. The second concern related to the practicality and acceptability of immunizing by DVI. Data from four pediatric clinical trials in Africa indicate that down to the age of 24 months, DVI is easy for operators to perform ([Citation136,Citation137], Jongo, in press, American Journal of Tropical Medicine and Hygiene [NCT02859350], Agnandji unpublished [NCT03521973]). Below the age of 2 years, with training and experience, DVI is feasible [Citation138]. In a survey in western Kenya of parents of infants and children vaccinated by the DVI route, there was good acceptability, in large part because DVI appeared less painful than IM, ID, or SC injections [Citation139]. The positive experience with DVI has supported its use for other vaccination approaches, such as prime and target vaccination [Citation140], and immunization with BCG [Citation141].
Genetically Altered Parasites:
Seattle Children’s Research Institute: Investigators at Seattle Children’s Research Institute created the first Pf genetically attenuated/altered parasites (GAP) that were early liver stage-arresting replication deficient (EARD) [Citation142]. In a clinical trial, an EARD GAP with deletions in three genes (P52, P36, and SAP1) administered by mosquito bite provided protection from mosquito bite challenge in 50% of immunized volunteers [Citation143]. Subsequent studies using rodent malaria models demonstrated superior efficacy when immunizing with late liver-stage arresting replication competent (LARC) GAP as compared to both EARD GAP and radiation-attenuated sporozoites [Citation144]. This finding led to the creation, using CRISPR/Cas 9 technology, of the first Pf LARC GAP, with a deletion in the PlasMei2 gene [Citation145] (US Pat. 10905,753). Further genetic engineering of this LARC GAP led to the creation of Sanaria® PfSPZ-LARC2 Vaccine, with deletions in both PlasMei2 and LINUP (liver stage nuclear protein) [145]. PfSPZ-LARC2 Vaccine shows no breakthroughs in a humanized mouse model of liver stage-to-blood stage transition (Goswami, unpublished). PfSPZ-LARC2 Vaccine will undergo initial clinical trials in 2023-2024.
Leiden University Medical Center: Investigators at Leiden University Medical Center, Nijmegen University Medical Center, and Sanaria created an EARD GAP with deletions in two genes (B9 and SLARP (=SAP1)). The injectable vaccine based on this EARD GAP, Sanaria® PfSPZ-GA1 Vaccine was safe and well tolerated following DVI administration but did not provide significant protection against mosquito bite CHMI in an initial clinical trial [Citation146]. Investigators at Leiden University Medical Center, working independently of the Seattle team, then used CRISPR/Cas 9 technology to delete the PlasMei2 gene, successfully producing a GAP (GA2) with LARC phenotype. Sequencing the complete chromosomal genome of the new LARC parasite revealed no changes from the PfNF54 background other than the deletion of the PlasMei2 gene. Permissions for a clinical trial were obtained from the Dutch Ministry of Infrastructure and the Environment [GGO IM-MV 20-018; December 2020] and from the Dutch Central Committee on Research Involving Human Subjects [CCMO; NL75577.000.21, July 2021]. Two months later, the team conducted the first study of a LARC GAP in humans using mosquito bite administration [Citation147].
Innovations under Development
With the goal of increasing the scale and efficiency of manufacture, potency of the vaccines, and reducing COGs, additional innovations are being developed:
Cryopreservation of mosquito eggs: There has been no method for cryopreserving the eggs of Anopheles spp. mosquitoes [Citation148]. Therefore, anopheline colonies have to be maintained continuously. Sanaria developed methods to cryopreserve Anopheles stephensi and Anopheles gambiae eggs (USSN 17/626,381; WO/2021/011503), allowing the creation of A. stephensi master egg banks of several genetically modified A. stephensi lines that have been developed as part of Sanaria’s research to improve Pf infectivity of mosquitoes [Citation148].
Robotic dissection: In the current manufacturing process, salivary glands harboring PfSPZ are isolated from mosquitoes by manual dissection. Improvements in equipment and technique (US Pat. 10781,419; US Pat. Pub. 21/0045375) have allowed increased rates of dissection from an average of about 50 mosquitoes per dissector per hour to greater than 300 per dissector per hour, with individual dissectors ranging from 280 to 440 mosquitoes per hour. Sanaria is working with Johns Hopkins University to incorporate elements of robotic dissection into the manufacturing process [Citation149]. Another research group developed an SPZ purification approach that is independent of dissection [Citation150].
In vitro culture of PfSPZ: Building on earlier work [Citation151], Sanaria staff have developed techniques for in vitro production of PfSPZ, negating the need for mosquitoes (US Pats: 9,878,026; 10441,646; 11207,395; USSN 17/529,812) [Citation9]. Using these techniques, PfSPZ can now be produced in culture plates and hollow fiber culture bioreactors. When available, in vitro culture in bioreactors should significantly lower COGs and enable the scale up in manufacturing required to meet Sanaria’s goals for MVPs for the regional elimination of malaria.
Monoclonal antibodies: Three different laboratories have used PBMCs collected from PfSPZ-immunized trial participants to generate human anti-PfCSP monoclonal antibodies [Citation152–155]. Two, one co-invented by Sanaria (US Pat: 11021,535) have completed successful clinical trials [Citation155–157]. These, or other anti-PfCSP monoclonal antibodies being developed, may provide additional weapons in the armamentarium against malaria.
3. PfSPZ technologies
3.1. Developing PfSPZ vaccine technologies aiming for safety and high-level efficacy
The discussion of PfSPZ vaccine technologies co-developed by Sanaria and collaborators is organized according to . Panel a of the figure [Citation8] depicts the steps whereby the immunogen – PfSPZ – arrives in the liver sinusoid via the vasculature, traverses Kupffer cells and hepatocytes, invades a hepatocyte through invagination, forms a parasitophorous vacuole, rounds up, enlarges, and replicates asexually over 6–7 days to produce 60,000 or more merozoites [Citation158]. In the absence of attenuation, progeny merozoites are released into the blood stream to begin the pathogenic cycle of blood stage asexual replication and sexual-stage transmission. The journey of PfSPZ and their development in the liver are the subject of intense scientific inquiry and are reviewed elsewhere [Citation159,Citation160].
Figure 2. PfSPZ platform technologies. (a) Illustration of a liver sinusoid showing the invading sporozoites (green progressing to red as they replicate and differentiate). H = hepatocyte, KC = Kupffer cell, EC = endothelial cell. Reproduced from Nature [Citation8] with permission. Design credit: Sumana Chakravarty. (b) radiation-attenuated and first-generation genetically attenuated (GA) PfSPZ invade hepatocytes and partially develop, but do not replicate. Infectious PfSPZ in PfSPZ-CVac invade hepatocytes and partially develop if a liver-active antimalarial is administered (e.g. pyrimethamine, azithromycin) or fully develop including normal replication (releasing merozoites into the blood stream) if a blood-stage active antimalarial is administered (e.g. chloroquine). The extent of development therefore depends on which drug is used and when it is administered. Second-generation GA parasites have a late-arresting phenotype and halt development before the release of merozoites. Potency appears to increase with further development, whereas safety concerns arise should the parasite release merozoites into the blood.
![Figure 2. PfSPZ platform technologies. (a) Illustration of a liver sinusoid showing the invading sporozoites (green progressing to red as they replicate and differentiate). H = hepatocyte, KC = Kupffer cell, EC = endothelial cell. Reproduced from Nature [Citation8] with permission. Design credit: Sumana Chakravarty. (b) radiation-attenuated and first-generation genetically attenuated (GA) PfSPZ invade hepatocytes and partially develop, but do not replicate. Infectious PfSPZ in PfSPZ-CVac invade hepatocytes and partially develop if a liver-active antimalarial is administered (e.g. pyrimethamine, azithromycin) or fully develop including normal replication (releasing merozoites into the blood stream) if a blood-stage active antimalarial is administered (e.g. chloroquine). The extent of development therefore depends on which drug is used and when it is administered. Second-generation GA parasites have a late-arresting phenotype and halt development before the release of merozoites. Potency appears to increase with further development, whereas safety concerns arise should the parasite release merozoites into the blood.](/cms/asset/47f7c977-e8f2-4d8a-b4f6-bd364fa4e192/ierv_a_2245890_f0002_oc.jpg)
Panel b shows examples of PfSPZ vaccine platforms, each attenuated by a different approach and characterized by the point of developmental arrest. The point of arrest is a key phenotype representing a potential trade-off: whereas growth and differentiation in the liver appear to be positively associated with increasing vaccine potency [Citation161], development to the late liver stages may be negatively associated with vaccine safety by increasing the possibility of breakthrough blood stage infection from the vaccine inoculum.
The initial method for PfSPZ attenuation was irradiation, achieved by x- or gamma-ray (e.g. 60Co). Radiation-attenuated PfSPZ are metabolically active, motile, can invade hepatocytes, express new antigens not expressed in PfSPZ (e.g. PfLSA1, which is used by Sanaria as a potency assay) and arrest early in liver stage development without replicating (early-arresting, replication-deficient, or EARD). The random DNA damage caused by administration of approximately 150 gray (Gy) of ionizing radiation disrupts development so that hepatic schizonts do not form [Citation1,Citation51]. Breakthrough blood stage infection has never occurred, despite the injection of 6538 doses of PfSPZ Vaccine into 2046 humans, comprising over 6.0 billion PfSPZ produced under cGMPs. Radiation-attenuated PfSPZ represent the first successful PfSPZ-based approach and are the most advanced in clinical development. The vaccine based on radiation attenuation, PfSPZ Vaccine, has been studied in 23 Phase 1 and Phase 2 completed or ongoing clinical trials, 13 of which have been in Africa (references for completed trials are [Citation1–7,Citation51,Citation65,Citation112,Citation124,Citation126,Citation129,Citation136,Citation137,Citation162–164] and Jongo, in press, Journal of Clinical Investigation and Jongo, in press, American Journal of Tropical Medicine and Hygiene [NCT03420053], Diawara unpublished [NCT03510481 and NCT03989102], Agnandji unpublished [NCT03521973], and Kublin unpublished [NCT04966871]). PfSPZ Vaccine is being considered for Phase 3 testing and licensure.
The first genetically attenuated (or genetically altered) (GA) parasite (GAP) vaccines tested in the clinic also arrested early in liver stage development without replication (EARD phenotype) and showed potency similar to PfSPZ Vaccine. GAP vaccines offer the theoretical advantage of a discrete gene deletion or deletions as the source of attenuation rather than the diffuse damage caused by radiation, but it is unclear how this impacts potency or safety including risk of breakthrough infection. The GAP technology also reduces safety concerns for personnel handling infected mosquitoes during manufacturing (since all parasites are attenuated) and simplifies manufacturing by eliminating the radiation step. Injectable early arresting GA PfSPZ have been manufactured according to cGMPs (Sanaria® PfSPZ-GA1 Vaccine) and studied in a Phase 1 trial [Citation146]. Three additional Phase 1 trials have been conducted using mosquito bite administration of other early arresting GAPs [Citation143,Citation165,Citation166].
The chemo-attenuated PfSPZ approach, which uses non-attenuated, fully infectious PfSPZ that are attenuated in vivo by a co-administered drug, can span the full spectrum of liver stage development. The PfSPZ undergo early or mid-stage arrest if the attenuating drug has liver stage activity (such as atovaquone or pyrimethamine) or may progress all the way to the blood stages causing transient parasitemia when the activity of the attenuating drug is restricted to the blood (such as chloroquine). Additional drugs of interest are antibiotics that target the apicoplast (e.g. clindamycin or azithromycin); they attenuate late during liver stages but before release into the blood, which may lead to a better tolerability profile than for chloroquine. The chemo-attenuated approach is called PfSPZ-CVac (chemoprophylaxis vaccination) when using GMP parasites. Ten Phase 1 trials and one Phase 2 trial of PfSPZ-CVac vaccines have been completed or are under way (references for completed trials are [Citation8,Citation68,Citation122,Citation126–128,Citation167,Citation168] and Sagara unpublished [NCT03952650]).
The newest, third generation PfSPZ vaccines are next-generation GAP that are late liver stage-arresting and replication competent (LARC) [Citation169]. LARC parasites undergo asexual multiplication in the liver before halting development prior to the formation and release of merozoites into the blood. Single-gene deletion (plasmei2−) Pf LARC sporozoites [Citation170] administered by the bites of 50 infected mosquitoes have been assessed in the Netherlands by Leiden University Medical Center (LUMC) and Radboud University Medical Center (Radboudumc). Immunizations by the bites of mosquitoes infected with Pf LARC sporozoites were well tolerated, safe (with no breakthrough Pf infections) and induced protective immune responses [Citation147]. A double deletion Pf LARC SPZ vaccine (plasmei2−/linup−) developed at the Seattle Children’s Research Institute (SCRI), PfSPZ-LARC2 Vaccine, has been manufactured in compliance with cGMPs and will go undergo initial clinical assessment of tolerability, safety, and VE against heterologous CHMI in 2023–2024 [Citation171].
Growth and replication amplify the pre-erythrocytic stage antigens expressed by LARC parasites, thereby exposing the host immune system to a wider array and greater abundance of epitopes. This two-tiered expansion of the immunome (biomass increase + diversification) increases potency and improves the absolute level of protection that can be achieved [Citation68]. However, it also increases the risk of breakthrough blood stage infection [Citation165,Citation172,Citation173].
3.2. Radiation-attenuated PfSPZ
Stephen Hoffman and Thomas Luke first envisioned that the classic mosquito bite model could be translated into a cGMP-compliant injectable product for vaccination against malaria [Citation87]. The biotechnology company Sanaria Inc. was founded in 2003 for this purpose, named after the Italian words for ‘healthy air’ as a riposte to this disease that has caused so much suffering. Malaria was thought to originate from ‘bad air’ (medieval Italian: mal’aria) such as the air emanating from the Pontine Marshes located to the south of Rome [Citation174]. A series of manufacturing innovations led to the successful production of aseptic, purified, cryopreserved PfSPZ using aseptic mosquitoes as the production platform [Citation88]. After 6 years of development, including the conduct of non-clinical studies and appropriate regulatory and ethical reviews, the first human volunteers were injected on 25 May 2009 at the National Naval Medical Center in Bethesda, Maryland, under the leadership of Judith Epstein, followed by a second group three weeks later at the Center for Vaccine Development (CVD) at the University of Maryland in Baltimore, under the leadership of Kirsten Lyke. This was the first of the 23 clinical trials testing PfSPV Vaccine. These studies have involved clinical teams from 15 institutions in the U.S.A., Germany, the Netherlands, Mali, Tanzania, Equatorial Guinea, Burkina Faso, Kenya, Gabon, and Indonesia. All have contributed greatly to the exploration and refinement of PfSPZ vaccinology (Box 2).
Table 1. Key milestones and innovations
As this was the first cGMP-produced whole-cell eukaryotic vaccine tested in humans, there was little guidance from the literature regarding how to immunize: what should be the dose size, number of doses and interval between injections? Mosquito bite immunizations leading to protection against a first CHMI had ranged from 5 to 10 biting sessions administered every 3 to 24 weeks resulting in exposure to the bites of 379 to 1681 infectious mosquitoes over 99 to 547 days [Citation25,Citation175]. Seminal studies established that greater than 1000 infectious bites were needed for consistent >90% protection (although one volunteer was protected after 379 bites), but otherwise precedents about how to optimally immunize were scant. A major knowledge gap was how many PfSPZ were injected by the immunizing mosquitoes in the early human studies. In a mouse model, observed counts in the skin after one mosquito bite were 0–1293 (median of 223) after 3 min of feeding and 0–1921 (median 315) after 15 min [Citation176]. Later, a different mouse model showed that Anopheles gambiae, an important vector for malaria in Africa, was able to cannulate capillaries with its proboscis and inject SPZ directly into the blood vessel lumen [Citation177]. It is not clear how these alternate routes of deposition (intradermal or intravascular) contribute to inducing infection in nature, or, in the case of SPZ administered by mosquito bite as immunogens, to inducing protective immunity. The issue is made more complex by the fact that SPZ deposited in the skin may partially develop [Citation178] and play an immunomodulatory role [Citation179–181], with substantial numbers migrating to lymph nodes proximal to the mosquito bite [Citation182,Citation183].
With this background, the initial PfSPZ Vaccine trial conducted by NMRC and CVD, with Sanaria sponsorship of the investigational new drug (IND) application and support from PATH and the Bill & Melinda Gates Foundation, administered four to six doses by the subcutaneous (SC) or intradermal (ID) routes, attempting to reproduce the probing behavior of mosquitoes. Since mouse studies had shown superiority of IV immunization, this route had been considered for the initial trial but had been judged too risky for first-in-humans testing (Epstein, personal communication). The ID and SC doses were escalated gradually from 2 × 103 to 1.35 × 105 PfSPZ. PfSPZ Vaccine was safe and well tolerated when administered by these routes but only 2/44 (4.5%) participants remained free of parasitemia after mosquito-bite induced homologous CHMI [Citation51].
To better understand the suboptimal protection results, investigations were undertaken by Robert Seder and colleagues at the US National Institutes of Health (NIH) Vaccine Research Center (VRC), comparing SC and IV immunization with PfSPZ Vaccine in rhesus monkeys. These studies showed that PfSPZ-specific, IFN-γ–producing, CD8 and CD4 T cells after IV administration comprised, respectively, a mean of 3.2% (range 2.8–3.6%) and 2.7% (range 1.7–4.6%) of the liver-resident mononuclear cell population 3–4 months after immunization, versus low to undetectable levels in SC immunized monkeys or controls [Citation51]. This finding suggested that protection would be improved in humans by administering the PfSPZ IV, providing passive carriage of the PfSPZ to the liver sinusoids rather than depositing the PfSPZ in the skin or subcutaneous tissues, from which possibly only a few could escape through gliding motility.
These findings in rhesus monkey livers post immunization [Citation51] were consistent with earlier murine studies showing the superiority of IV immunization over other routes [Citation179,Citation184]. In addition, the pivotal studies immunizing mice with radiation-attenuated PbSPZ at New York University published in 1967 [Citation16] as well as other early murine studies with Plasmodium yoelii (Py) SPZ at NMRI [Citation185] had used the IV route. Based on this experience, Sanaria approached the US Food and Drug Administration (FDA) with a proposal to conduct a second study assessing IV administration of PfSPZ Vaccine. Because there was little to no experience with IV administration of a preventative, anti-infectious disease vaccine, there were extensive discussions with the FDA before the approach was accepted. Anthony Fauci at NIAID, NIH was then approached regarding funding this trial, and when told that the FDA had recently approved the clinical trial plan, he agreed to fund the trial at the NIH Clinical Center. In this trial, conducted by the VRC under Robert Seder’s leadership, PfSPZ Vaccine was administered through an IV cannula rather than SC or ID. At each dosing level, a pilot group of three participants received two IV doses to assess for hypersensitivity reactions followed by FDA safety review before subsequent immunizations in the three pilot subjects and in larger groups were permitted. The trial reached its target of four to five doses of 1.35 × 105 purified PfSPZ administered by IV push through a catheter without any concerning safety signals. After mosquito-bite induced, homologous CHMI, 6/9 and 6/6 of participants receiving 4 or 5 vaccine doses, respectively, remained free of parasitemia, compared to 1/6 non-immunized controls, providing a vaccine efficacy (VE) of 60% and 100% in the 4 and 5 dose groups, respectively [Citation1]. Presumably the improved VE over the first study (ID/SC administration) reflected greater access of the PfSPZ to the liver, although the studies in rodent models have shown that even with approximately equal liver loads, IV may be superior to ID, possibly because deposition of SPZ in the skin favors regulatory immune responses that negatively impact protection [Citation178–181].
Greater than 90% sterile protection had never been achieved before in humans by any candidate malaria vaccine (excluding mosquito-bite administration of PfSPZ). This success launched multiple clinical trials of PfSPZ Vaccine over the ensuing 10 years (see the Appendix) sponsored by Sanaria or supported by Sanaria through cross-referenced regulatory filings, with several key findings:
Direct venous inoculation (DVI) became the preferred method of administration: After the first IV trial, Sanaria, VRC, and the CVD at the University of Maryland conducted one more study administering PfSPZ through an IV cannula, but all 20 subsequent studies have used DVI. A vein is punctured with minimal discomfort with a small (25-gauge) needle and vaccine is injected over a few seconds in 0.3 to 0.5 mL of diluent (phosphate buffered saline with human serum albumin), a procedure that in most recipients is well tolerated and causes no local adverse events (AEs) beyond the trauma of venipuncture [Citation114]. The improved protection resulting from IV administration of attenuated PfSPZ parallels the greater infectivity of non-attenuated PfSPZ when administered by the IV as opposed to the intramuscular (IM), ID or SC routes [Citation113,Citation114,Citation117–121,Citation186].
The degree of protection against CHMI depended on the dose of PfSPZ administered: In CHMI studies, whether performed by mosquito bite or by DVI of non-irradiated, fully infectious PfSPZ (Sanaria® PfSPZ Challenge – see Box 1), there has been a clear dose response for protection induced by PfSPZ Vaccine [Citation1], seen up to doses of about 9.0 × 105 PfSPZ per injection. As individual doses were increased above 9.0 × 105 PfSPZ, further improvements were not always seen and in some instances VE diminished [Citation2]. The underlying mechanism for this finding has not been explained, nor has the threshold been precisely defined in various target populations.
The number of doses influenced protection: In a trial of PfSPZ Vaccine by Peter Kremsner, Benjamin Mordmüller and colleagues at the Institute for Tropical Medicine (ITM), University of Tübingen in Germany, two attempts at immunizing with two doses of PfSPZ Vaccine (1.35 × 106 PfSPZ/dose and 2.7 × 106 PfSPZ/dose) showed only 50–67% protection against homologous CHMI performed using PfSPZ Challenge (NF54), but three doses protected 5/5 participants,Footnote6 even though the total numbers of PfSPZ administered by two or three doses were the same [Citation6]. The difference between three and five doses has been less marked than the difference between two and three doses [Citation187], and for this reason a three-dose regimen has been selected as the standard for further development based on practicality.
The protection induced by PfSPZ Vaccine extended to heterologous parasites but did not reach 100%: In the field, especially in regions of high malaria transmission, the parasites transmitted by mosquitoes are heterogeneous, differing antigenically from the vaccine strain because of natural variability in malaria proteins and the outbred structure of the parasite population. To assess the ability of PfSPZ Vaccine to induce cross-strain protection using CHMI, a challenge strain was selected that was heterologous to the vaccine strain. Sanaria and collaborators chose Pf7G8 for this purpose as it fulfills two important criteria. First, it is a reasonably good gametocyte producer in culture and can be manufactured as PfSPZ Challenge (7G8) or used to infect mosquitoes that can later be used for CHMI. Second, Pf7G8 ‘brackets’ strains in Africa by being more distant at the genome, proteome, and epitome level from PfNF54 (the vaccine strain) than all other Pf isolates from across Africa sequenced in a comparative study (n = 704) [Citation111]. Pf7G8 is a clone of the Brazilian Pf strain IMTM22 [Citation188], whereas PfNF54 [Citation110] is a Pf strain with a genome consistent with West African originFootnote7 [Citation189–191]. In the first assessment of PfSPZ Vaccine for heterologous protection, conducted by Judith Epstein and colleagues, a 5-dose regimen was tested at NMRC/WRAIR and protected 4/5 (80%) volunteers against heterologous Pf7G8 CHMI performed by mosquito bite 3 weeks after vaccination [Citation187]. This indicated that protective cross-strain immunity was induced. In a subsequent study at the CVD conducted by Kirsten Lyke and colleagues, six research subjects protected against homologous mosquito-bite CHMI at 19 weeks underwent a second mosquito-bite CHMI 14 weeks later at 33 weeks after last vaccine dose using Pf7G8 and 5/6 were protected (83%) [Citation5]. In a trial at ITM in Germany conducted by Benjamin Mordmüller, 10/12 (83.3%) volunteers were protected against DVI with PfSPZ Challenge (7G8) three or 10 weeks after immunization, confirming the earlier NMRC/WRAIR and CVD results [Citation6]. These findings were consistent with the cross-strain protection seen in the original studies using mosquito bite administration [Citation25].
PfSPZ Vaccine gave protection lasting >1 year: In the second VRC trial using IV administration, led by Robert Seder in collaboration with Kirsten Lyke at the University of Maryland CVD, 5/5 (100%) volunteers protected against mosquito-induced homologous CHMI at ~20 weeks after immunization were sterilely protected against repeat mosquito-induced homologous CHMI at 59 weeks indicating that protection is durable for 14 months [Citation4], at least in the setting of having immunity boosted by a first CHMI. At the CVD, as just described, 5/6 (83%) volunteers protected against mosquito-induced homologous CHMI at 19 weeks were sterilely protected against mosquito-induced heterologous (7G8) CHMI at 33 weeks, indicating that heterologous protection was also durable [Citation5].
Condensed regimens with a multi-dose prime were protective: Experience with the typhoid vaccine Ty21a, which like PfSPZ is thought to protect through CD8 T cells [Citation192], indicated that a typhoid vaccine regimen of three doses administered at 1- to 2-day intervals was superior to a regimen with more widely spaced doses [Citation193]. Attempting to reproduce this finding from Salmonella, a Day 1, 3, 5, 7 multi-dose prime regimen with a 16-week fifth dose was assessed and gave the best heterologous protection in a regimen comparison trial of PfSPZ Vaccine performed at the CVD by Kirsten Lyke and NMRC by Judith Epstein. Six/15 (40%) of vaccinees remained without parasitemia compared with 0/8 controls, following a stringent mosquito-induced Pf7G8 CHMI conducted at 13 weeks after immunization. This was twice as high as the comparator 3-dose regimens administered over 0, 8, and 16 weeks, despite a lower total dose of PfSPZ (2.25 × 106 total PfSPZ vs. 2.7, 5.4, and 6.3 × 106 total PfSPZ in the other three groups) [Citation163]. A trial at ITM, University of Tübingen, conducted by Peter Kremsner and Benjamin Mordmüller, then showed favorable results for a condensed regimen using a simpler two-dose multi-dose prime (Days 1 and 8) with the final dose at week 4 rather than week 16: 14/17 (78.8%) volunteers were protected against 3-week or 10-week heterologous CHMI by DVI of PfSPZ Challenge (7G8) after 3 doses of 9.0 × 105 PfSPZ administered on Days 1, 8, and 29 [Citation6]. A subsequent study conducted in Equatorial Guinea conducted by Said Jongo and Vicente Urbano Nchama showed that the Day 1, 8 and 29 regimen was more protective against homologous CHMI by DVI of PfSPZ Challenge (NF54) than the day 1, 3, 5, 7, week 16 regimen (14/21 or 67% vs. 7/18 or 39% of vaccinees remained without parasitemia) [Citation129], and for this reason the Tübingen condensed regimen (day 1, 8 and 29) developed by Peter Kremsner and Benjamin Mordmüller was selected for further development.
Antibody levels to Pf circumsporozoite protein (CSP) measured by ELISA have correlated with protection against CHMI: Antibody levels to the main surface protein of PfSPZ, the Pf circumsporozoite protein (PfCSP), as measured by ELISA, have in some (but not all) studies correlated with protection in malaria-naïve individuals undergoing CHMI [Citation4,Citation187], and in malaria-exposed individuals infected in African field studies [Citation3,Citation7,Citation65]. Although differences at the group level between protected and non-protected individuals have been statistically significant, extensive overlap in PfCSP antibody levels between the protected and non-protected groups have prevented the definition of a threshold that predicts protection for a specific individual. The association is complicated by the fact that CSP antibody levels tend to increase with higher doses of PfSPZ, while protection plateaus or even diminishes [Citation2]. Thus, the antibody levels in those protected at a lower dose may be lower than the antibody levels of those unprotected at a higher dose (see B above). These findings reinforce the conclusion that although anti-CSP antibody responses may contribute to protection, they are not the main mechanistic correlate.
PfSPZ Vaccine induces durable protection against naturally transmitted Pf malaria infection in African adults: A randomized, double-blind, placebo-controlled trial was conducted in Mali by the Malaria Research and Training Center (MRTC), University of Bamako, led by Ogobara Doumbo, Mahamadou Sissoko, and colleagues, in collaboration with Patrick Duffy and Sara Healy at the LMIV, NIAID. This study assessed five doses of vaccine (2.7 × 105 PfSPZ/dose) at 0, 4, 8, 12 and 20 weeks administered by DVI. The trial measured VE against Pf infection by microscopic examination of thick-blood smears (TBS) every 2 weeks (or whenever a participant presented with symptoms) during a 24-week follow-up period. Because TBS were performed and read at least every 2 weeks, the trial could assess VE against Pf infection (not just against clinical malaria as assessed in trials of RTS, S, and R21). VE against Pf infection was 52% by 1 minus the estimated hazard ratio (time-to-event analysis, log-rank p = 0·01) and 29% by 1 minus the estimated risk ratio (proportional analysis, log-rank p = 0·006) [Citation65] (). Similar results were obtained in two follow-up field trials with randomized, double-blind, placebo-controlled designs. The first was a follow-on trial in Mali conducted by the MRTC/LMIV team (VE = 51% by 1 – hazard ratio, log-rank p = 0·004; and 24% by 1 – risk ratio, log-rank p = 0·031) [Citation3] and the second was in Burkina Faso conducted by the National Centre for Malaria Research and Training (CNRFP) under the leadership of Sodiomon Sirima in collaboration with Matthew Laurens at the CVD, University of Maryland (VE 48% by 1 – hazard ratio, log-rank p = 0.061, and 38% by 1 – risk ratio, log-rank p = 0.017) [Citation7]. Both follow-up trials used three rather than five doses. The dose of PfSPZ was 1.8 × 106 PfSPZ per injection in the second Mali trial and 2.7 × 106 PfSPZ per injection in Burkina Faso, administered at 0, 8, and 16 weeks. These two studies confirmed the earlier findings in the U.S.A. that three doses could protect as well as 5, as long as the dose of PfSPZ per injection was increased to maintain the same total dose [Citation187]. In the CNRFP study, VE was sustained at 46% for 18 months (through two consecutive July-to-December rainy seasons) by time-to-event analysis (log-rank p = 0.018), supporting the earlier findings in the U.S.A. using CHMI that protection can be sustained for more than a year. Importantly, in all three initial field trials, clearance of any existing parasitemias was performed in all participants before the first immunization to reduce the immunosuppressive effects of blood stage infection on the induction of protective immunity (further discussed below). Clearance was then performed a second time prior to the third immunization, to avoid the immunosuppressive effects of blood stage infection acquired during the intervening period and to enable accurate determination of the incidence of new infections. After the completion of these three trials in West Africa in which immunization was done over 16 to 20 weeks, two additional field trials have been conducted in Malian adults using the condensed 4-week, 3-dose regimen first demonstrated in the University of Tübingen trial discussed in F above [Citation6] both led by Halimatou Diawara and Sara Healy. The first trial [NCT03510481, MLSPZV3] omitted parasite clearance before the first immunization, whereas clearance was performed during the second trial [NCT03989102, MLSPZV4], which was restricted to women of childbearing potential (WOCBP). Preliminary analyses suggest that efficacy is seen when parasites are cleared by administration of antimalarials before vaccination, but not when parasite clearance is omitted (Diawara unpublished [NCT03989102]) [Citation112].
Figure 4. Protective efficacy of PfSPZ Vaccine against naturally transmitted Pf infection in Malian adults (MLSPZV1 clinical trial). Vaccine efficacy was analyzed by time to first positive blood smear, with day 0 at 28 days after the fifth vaccination. The inverse survival curves include participants who received all five vaccinations and were evaluable for the primary exploratory efficacy endpoint. Five participants (one in the PfSPZ Vaccine group and four in the placebo group) were censored from the primary efficacy analysis because they had a positive blood smear before 28 days after the fifth vaccination. PfSPZ=Plasmodium falciparum sporozoite. Reproduced with permission from the Lancet ID (no changes were made) [Citation3].
PfSPZ Vaccine induces minimal protection against naturally transmitted malaria infection in African infants who did not receive treatment to clear parasitemia before the first dose: Children age 5–11 months at the time of immunization by DVI with PfSPZ Vaccine were followed over 12 months for incident malaria in Western Kenya and did not show statistically significant protection compared to controls at the primary six-month timepoint, although protection was significant during the first 3 months after immunization in the highest dose group (1.8 × 106 PfSPZ administered by DVI weeks 0, 8, and 16) [Citation164]. The results of this study, performed by Martina Oneko and colleagues at the Kenya Medical Research Institute (KEMRI) in collaboration with Robert Seder and colleagues at the VRC, Laura Steinhardt, and colleagues at the US Centers for Disease Control and Prevention (CDC) and the Liverpool School of Tropical Medicine (LSTM), were interpreted to reflect the reduced capacity of the infant immune system to develop potent T cell responses, as no SPZ-specific cellular responses were detected after vaccination [Citation164]. However, the failure to presumptively treat any existing parasitemias before immunization, compared to four of the five Mali trials all four of which showed protection, likely contributed to poor VE. A clinical trial in which presumptive treatment is administered before the first (or each) dose is proposed as a next step to determine if infants can be protected by this vaccine.
PfSPZ Vaccine induces protection against clinical malaria in African infants [Citation164]: The field trials of PfSPZ Vaccine described in H and I above have been powered to detect differences in Pf infection rates between vaccinees and placebo recipients. Because not all new infections are symptomatic, the trials have not been equivalently powered to show differences in clinical malaria episodes, which occur with lower frequency than infections, particularly in malaria-exposed adults. Protection against both Pf infection (VE 41%, p = 0.031) and clinical malaria (all episodes) (VE 45.8%, p = 0.027) was shown in the highest dose group at the three-month time point in the trial in Kenyan infants described above (1.8 × 106 PfSPZ weeks 0, 8 and 16). In the unpublished trial in Malian WOCBP (NCT03989102, MLSPZV4), protection against both Pf infection and clinical malaria has been demonstrated in pregnant and non-pregnant women through two malaria transmission seasons without administration of an intervening boost [Citation112] in 2023.
Antibody levels and cellular responses induced by PfSPZ Vaccine in malaria-exposed Africans were lower than when the same regimen was administered to malaria-naïve adults in the U.S.A. and are lower in African adults than in African children: When the same regimen was administered in the U.S.A. and in Africa, antibody responses to PfSPZ Vaccine in semi-immune adults in Africa [Citation3,Citation65] were 9–28 times lower than the antibody responses in malaria-naïve subjects in the U.S.A. immunized with the same regimen [Citation163,Citation187] (), despite the fact that the adult participants in both African studies were cleared of any existing parasitemia prior to immunization. Similarly, T lymphocyte responses were fivefold lower in malaria-exposed African adults [Citation124] than the responses to the same immunization regimen in American adults [Citation187] () (in this set of studies, African adults were not cleared prior to immunization). In addition, there was an age-related gradient in immune responses in malaria-exposed Africans. When antibody responses to PfCSP were compared among malaria-exposed Tanzanian infants, 1–5-year-olds, 6–10-year-olds, 11–17-year-olds and 18–45-year-olds immunized with three doses of 9.0 × 105 PfSPZ of PfSPZ Vaccine in a study led by Salim Abdulla and Said Jongo, there was progressive hyporesponsiveness with older age groups: infants showed the same high responses (net levels 14,335) as malaria-naive adults in the U.S.A. (13,174), with generally diminishing responses in 1–5-year-olds (8,331), 6–10-year-olds (2,557), 11–17-year-olds (5,040) and 18–45-year-olds (1,402) [Citation136] (in this study, there was also no clearance prior to immunization). There were similar findings with respect to T cell responses – lower responses in teenagers and adults compared to 6–10-year-olds. However, T cell responses were lower in 1–5-year-olds than in 6–10-year-olds and absent in infants, likely caused by an immature cellular immune response in the early stages of life [Citation194]. These findings are further discussed in section 4.1.1 (vaccine hyporesponsiveness) below.
Figure 5. Differential antibody and T-cell responses to PfSPZ Vaccine by age in Tanzanian adults, adolescents, children, and infants. Panel a. Antibody levels to Pf circumsporozoite protein as measured by enzyme-linked immunosorbent assay (difference between two-weeks-post-immunization and pre-immunization) after immunizing 18–45-year-olds, 11–17-year-olds, 6–10-year-olds, 1–5-year-olds, and 6–11-month-olds with three doses of 9.0x105 PfSPZ of PfSPZ Vaccine at eight-week intervals. VRC314, an earlier trial in which malaria-naive US adults were immunized with the same dosage regimen and antibody levels were assessed using the same PfCSP ELISA conducted in the same laboratory, is shown as a comparison (for VRC314, filled in circles indicate volunteers protected against CHMI, empty circles the unprotected volunteers). Medians with interquartile ranges are shown. Panel b. PfSPZ-specific memory CD4 T-cell responses pre- and post-vaccination after incubation with PfSPZ, expressed as the percent of cells in the blood expressing interferon gamma (IFN-γ), interleukin 2 (IL-2), or tumor necrosis factor alpha (TNF-α) at pre-immunization or 2 weeks after the first and third doses of PfSPZ Vaccine (9.0×105). VRC314 data (malaria-naive adults) are included as a comparison. Reproduced with permission from the American journal of Tropical Medicine and Hygiene (no changes were made) [Citation136].
3.2.1. Safety
The findings described above were derived from trials of PfSPZ Vaccine in which 6538 doses of PfSPZ were injected into 2046 subjects aged 5 months to 61 years in Tanzania, Kenya, Mali, Burkina Faso, Gabon, Equatorial Guinea, Germany, the Netherlands, and the U.S.A., including 873 doses administered by DVI to 330 infants. Seventeen of the 20 trials utilized a randomized, double-blind placebo-controlled design with an inert placebo comparator, normal saline. PfSPZ Vaccine and normal saline are clear, colorless, odor free, non-viscous solutions indistinguishable by clinical teams or trial participants, allowing masked allocation. These double-blind, placebo-controlled trials have permitted direct comparison of the adverse event profile after administration of PfSPZ Vaccine with background rates in the community because normal saline placebo should not cause any local or systemic reactogenicity. To record adverse events, a relatively standardized list of local and systemic signs and symptoms was solicited after injections in these trials, generally for 7 days after each immunization. Investigators asked about local adverse events including pain, tenderness, erythema, induration, swelling, and pruritus and about systemic adverse events. For verbal individuals, systemic adverse events were subjective fever, headache, fatigue, myalgia, and arthralgia, and for pre-verbal children, drowsiness, irritability/fussiness, and inability/refusal to eat or drink. Body temperature was measured in all individuals to assess the presence of objective fever, and the presence of non-focal rash/urticaria/pruritus was assessed in children. Laboratory tests were performed pre- and post-vaccination, including leukocyte count, neutrophil count, lymphocyte count, platelet count, hemoglobin, alanine aminotransferase (ALT), aspartate aminotransferase (AST) and serum creatinine.
A meta-analysis of safety was performed by Sanaria (Church, unpublished data) using the 10 randomized trials from Africa where unblinded safety data were available [Citation3,Citation7,Citation65,Citation124,Citation129,Citation136,Citation137,Citation162,Citation164] (Jongo, in press, Journal of Clinical Investigation and Jongo, in press, American Journal of Tropical Medicine and Hygiene [NCT03420053 and NCT02859350]). These included three trials enrolling children and infants. There were no differences between vaccinees and normal saline placebo recipients in total AEs or laboratory abnormalities (or specific AEs or specific laboratory abnormalities) in adults, children, or infants, indicating that PfSPZ Vaccine had caused few, if any, adverse events, in malaria-exposed Africans, at doses up to 2.7 × 106 PfSPZ in adults and 1.8 × 106 PfSPZ in infants and children. A larger database is needed to estimate the frequency of uncommon adverse reactions if such occur. Of note, one of the 10 trials enrolled 9 Tanzanian adults infected with HIV; the irradiated PfSPZ were safe and well tolerated in these individuals (Jongo, in press, Journal of Clinical Investigation [NCT03420053]), likely because the intrinsic, radiation-induced attenuation of PfSPZ Vaccine prevents replication without any dependence on an intact immune system to halt parasite development.
As a cost-saving measure, PfSPZ Vaccine studies conducted in the U.S.A. have not included blinded, randomized controls injected with normal saline placebo, making it difficult to distinguish between adverse events caused by vaccination and background rates [Citation1,Citation4,Citation5,Citation51,Citation163]. Thus, less information is available about the adverse event profile in malaria-naive individuals. A trial of PfSPZ Vaccine conducted by the ITM in Tübingen [Citation6] and a second in the Netherlands [Citation146] did include normal saline controls, however. In the Tübingen trial, in the group that received 9 × 105 PfSPZ per dose on Days 1, 8 and 29 (n = 17), a small number of systemic reactions were observed; in the group receiving a higher dose of 1.35 × 106 PfSPZ on Days 1 and 8, 4/6 subjects had noteworthy systemic symptoms 10–24 h after administration including mild fever, headache, chills, sweats, and fatigue; in the group receiving the still higher dose 2.7 × 106 PfSPZ on Days 1 and 8, 1/6 subjects experienced Grade 3 fever, chills, sweating, myalgia, fatigue, and vomiting the evening of the day of the second immunization [Citation6]. Although there were no statistically significant differences in AE rates between PfSPZ Vaccine and placebo recipients in these groups, the lack of statistical significance may have been an artifact of the small sample size. Since the dose planned for future use is 9.0 × 105 PfSPZ, it is anticipated that overall, the tolerability of PfSPZ Vaccine at the planned dose will be acceptable in both malaria-exposed and malaria-naive individuals. However, more data are needed in malaria-naive individuals.
Two serious adverse events occurred in a trial in Equatorial Guinea and were assessed as possibly related to PfSPZ Vaccine administration, primarily on the basis of timing ([Citation126] and Jongo, in press, American Journal of Tropical Medicine and Hygiene [NCT02859350]). In the first event, a 19-year-old woman became pregnant at the same time as receiving a first dose of 2.7 × 106 PfSPZ by DVI and experienced a spontaneous abortion at 9 weeks. In the second event, a 15-year-old boy experienced a seizure 3½ hours after receiving a third dose of 1.8 × 106 PfSPZ by DVI, with no recurrence. Electroencephalogram (EEG) revealed underlying abnormalities consistent with generalized epilepsy. A consulting neurologist posited that the general inflammatory response to the vaccine may have lowered the seizure threshold in this individual who appeared predisposed to having a seizure disorder. No additional SAEs deemed possibly related to PfSPZ Vaccine have been recorded in any trials.
3.2.2. Next steps
Trials in endemic areas: MRTC, LMIV, and Sanaria have initiated a fifth field trial of PfSPZ Vaccine in Mali, enrolling 6-to-10-year-olds (NCT04940130), led by Issaka Sagara of MRTC and David Cook of LMIV. In parallel, a first field trial has been initiated in Indonesian soldiers (NCT03503058), a collaboration between the Faculty of Medicine, University of Indonesia, the Oxford University Clinical Research Unit, Jakarta (OUCRU, Jakarta), the Health Research Center, Army of the Republic of Indonesia, the Eijkman Center for Molecular Biology, National Research and Innovation Agency, Jakarta, and Sanaria, led by Erni Nelwan and Kevin Baird. Both trials are testing the down-selected days 1, 8, and 29 regimens (9.0 × 105 PfSPZ per dose). The Mali trial is notable because it is the first pediatric field trial in which participants have been cleared with antimalarial drugs (artemether-lumefantrine) before both the first and third (last dose) of vaccine in an effort to eliminate preexisting parasitemia. This contrasts with the Kenya trial and a trial in Gabonese children, which both yielded disappointing efficacy results ([Citation164], Agnandji unpublished [NCT03521973]). In adults, clearance seems essential to vaccine potency when immunizing malaria-exposed individuals, and it is likely that the same is true in children. The Indonesia trial is notable because it is the first Phase 2 field trial in which the participants are malaria-naive and immunized before their first exposure to endemic malaria. This is possible because the soldiers are based in a transmission-free area of western Indonesia and have been deployed to a highly malarious area in the eastern part of the country. According to Indonesian military medical doctrine, chemoprophylaxis is not administered, making it possible to assess VE against both Pf and Pv (both intensely transmitted in eastern Indonesia) by comparing attack rates between vaccinees and blinded normal saline controls. This trial is a particularly stringent test of field efficacy because of the antigenic distance between the West African PfNF54 vaccine strain and the Pf parasites transmitted in New Guinea [Citation110].
Finally, a first trial is planned in pregnant Malian women with immunization in the third trimester, and, if this is safe, the second trimester. This is a collaboration between Halimatou Diawara and Alassane Dicko at MRTC, Sara Healy, and Patrick Duffy at LMIV and Sanaria. Pregnancy malaria is a major source of maternal, fetal, and neonatal morbidity and mortality throughout sub-Saharan Africa despite the institution of intermittent preventative treatment in pregnancy (IPTp) as recommended by WHO, and a vaccine is needed (reviewed in [Citation195–197]). This trial and planned follow-up VE trials in pregnant women are expected to provide the data to support end-of-Phase 2 meetings with regulatory agencies in preparation for confirmatory Phase 3 studies in women of childbearing potential and pregnant women.
Trials in the US: One trial is nearly completed, and another is under way to assess the efficacy against heterologous CHMI (Pf7G8) of the day 1, 8, and 29 regimen (9.0 × 105 PfSPZ per dose) in malaria-naive adults: the Warfighter 3 trial (USSPZV6) (NCT04966871) and the TravSPZV1 trial (USSPZV7) (NCT05604521). Both trials are comparing early heterologous CHMI (2 or 3 weeks after the last immunization) with delayed heterologous CHMI (10 or 12 weeks). The first trial has been performed by James Kublin at the Fred Hutchinson Cancer Research Center and the Seattle Children’s Research Institute (SCRI) in Seattle and the second is underway at the University of Maryland CVD led by Kirsten Lyke. Results from these studies should be available in 2023–2024.
3.3. Chemo-attenuated PfSPZ
The chemo-attenuated PfSPZ vaccine approach uses live, non-attenuated PfSPZ, allowing varying degrees of liver stage development before the administration of a partner drug to kill the parasite in vivo (). This is currently the most potent approach to immunizing humans with PfSPZ. As with radiation-attenuated SPZ, proof of concept was provided in rodents [Citation44,Citation185,Citation198] and then demonstrated in humans immunized via mosquito bite. The latter, a seminal study by Robert Sauerwein, Meta Roestenberg, Matthew McCall, and colleagues at Radboud University Medical Center (Radboudumc), Nijmegen, showed 100% protection in humans 8 weeks after immunization using ‘chemoprophylaxis with sporozoites’ (CPS). Persistent sterile immunity was demonstrated in 4 of 6 vaccinees who underwent repeat CHMI 28 months after vaccination [Citation83,Citation199]. Only 36–45 bites of mosquitoes infected with Pf sporozoites were required to induce this durable protection as opposed to >1000 for radiation-attenuated PfSPZ, indicating much greater potency. CD4 and CD8 T cells expressing cytotoxic markers were associated with immunity [Citation200]. Follow-on trials indicated that protection was dose dependent [Citation201].
These original studies were done using chloroquine (CQ) as the partner drug, a selective blood schizonticide allowing normal liver stage development and release of progeny merozoites into the blood stream to invade erythrocytes before being killed (). CQ can be replaced with other drugs, including mefloquine, provided that the immunizing strain is susceptible [Citation202]. CQ and mefloquine rapidly kill the parasites in the blood, but nevertheless vaccinees experience transient parasitemia, usually peaking on days 7 or 8 after each vaccine dose. Depending on the density, this can cause the typical signs and symptoms of clinical malaria, including fever, chills, headache, and myalgia. These signs and symptoms generally occur after the first dose of vaccine and with the highest dose regimens and can be largely prevented by presumptive treatment with non-steroidal anti-inflammatory drugs [Citation68]. The chemo-attenuated approach entails the safety concern that if drug levels are not adequate, parasitemia might recrudesce, leading to unchecked and potentially serious blood stage infection.
Sanaria has developed a vaccination approach called PfSPZ-CVac (chemoprophylaxis vaccination) mirroring CPS but using injectable PfSPZ (PfSPZ Challenge – see Box 1), and has conducted studies to systematically improve VE, to simplify the immunization and drug regimens, to move from protection against homologous CHMI with PfNF54 to protection against heterologous CHMI with Pf7G8, and to achieve VE against heterologous CHMI with a partner drug that prevents transient parasitemia and associated symptoms. These studies are described next and are listed in the Appendix.
3.3.1. Improving PfSPZ-CVac efficacy
PfSPZ-CVac (CQ) was initially tested at Radboudumc (Nijmegen) by Robert Sauerwein and colleagues using ID administration of three doses of 7.5 × 104 PfSPZ at 4-week intervals and was not protective against homologous CHMI at 10 weeks (14/14 vaccinees developed parasitemia) [Citation122]. PfSPZ-CVac (CQ) was then tried by Peter Kremsner and Benjamin Mordmüller at the ITM, University of Tübingen using DVI instead of the ID route, and achieved 100% protection at 10 weeks against homologous CHMI. The Tübingen trial administered three injections at four-week intervals, with a clear dose-response: 33% VE with 3 doses of 3.2 × 103 PfSPZ (3/9 protected), 67% VE with 3 doses of 1.28 × 104 PfSPZ (6/9 protected), and 100% VE with 3 doses of 5.12 × 104 PfSPZ (9/9 protected) [Citation8]. This transition from no protection to 100% protection by changing the route of administration from ID to DVI and the clear dose response mirrored the experience with PfSPZ Vaccine. However, far fewer PfSPZ were needed to achieve 100% protection against homologous CHMI at 10 weeks with PfSPZ-CVac (three doses of 5.12 × 104 PfSPZ = total of 1.536 × 105 PfSPZ) than to achieve 100% protection against homologous CHMI at 3 weeks with PfSPZ Vaccine (5 doses of 1.35 × 105 PfSPZ = total of 6.75 × 105 PfSPZ) [1] – a greater than fourfold difference in potency with large implications for the potential cost of goods (COGS) when vaccines are marketed for use. Subsequent to the initial work in Tübingen, PfSPZ-CVac (CQ) immunization using the identical regimen (5.12 × 104 PfSPZ weeks 0, 4 and 8) gave 80% VE (4/5 protected) against homologous CHMI at 13 weeks after last dose of vaccine in a study by Sara Healy and Agnes Mwakingwe-Omari at LMIV, NIH, confirming the Tübingen findings [Citation68].
3.3.2. Simplifying the immunization and drug regimen
In the above studies, a loading dose of 10 mg/kg CQ base was administered 2 days before the first dose of PfSPZ and 5 mg/kg CQ was administered weekly thereafter for a total of 10 doses including the loading dose, with the last dose 5 days after the last injection with PfSPZ Challenge. Adding these visits to the three visits for immunization, 13 visits were required to complete the regimen, too complex for practical application. To address this concern, investigators at ITM, Tübingen, had compared, in their first study, the standard regimen of three doses at 4-week intervals to two condensed regimens: three doses at 14-day intervals and three doses at 5-day intervals. The 5-day interval regimen allowed the administration of the second and third doses of PfSPZ before the occurrence of the transient parasitemia from the preceding doses, which occurred on days 6–8 after PfSPZ injection. The 5-day interval also allowed the investigators to see if administration of only three doses of CQ, given on the same days as PfSPZ injection, would provide adequate drug coverage. Both condensed regimens used the 5.12 × 104 PfSPZ dose. VE at 10 weeks against homologous CHMI was 63% (5/8 protected) for the 5-day interval regimen and 67% (6/9 protected) for 14-day interval regimen, compared to 100% for the gold standard 28-day intervals, indicating that shortening the interval led to a loss of VE [Citation8,Citation203]. Of note, the administration of three doses of CQ at the same time as the administration of PfSPZ Challenge in the 5-day interval regimen proved effective in clearing all transient parasitemias. After this initial trial, a higher dose, 1 × 105 PfSPZ, was subsequently tested at the Kaiser Permanente Washington Health Research Institute in Seattle using the 5-day interval regimen in an attempt to compensate for the loss of VE; VE at 10 weeks was 75% (6/8 protected), appearing to narrow the gap between the condensed and standard regimens [Citation127]. These studies proved that simple (3-visit) regimens were possible.
In their second PfSPZ-CVac trial, ITM assessed a new approach to improve potency, which was to extend the interval before the third dose of the 5-day regimen to 21 days (e.g. dosing on days 1, 6 and 29), hypothesizing that a delayed third dose would boost more effectively [Citation128]. A dose of 1.1 × 105 PfSPZ of PfSPZ Challenge was used for each injection. CQ (10 mg/kg of CQ base) was administered three times just before PfSPZ injection, maintaining the 3-visit regimen. Immunization was well tolerated and all transient parasitemias cleared. Heterologous CHMI at 12 weeks gave 77% protection (10/13 protected), equivalent to VE in Seattle where 3 doses at 5-day intervals had provided 75% protection against homologous CHMI (6/8 protected). A trial providing a direct comparison of the day 1, 6, and 11 and the day 1, 6, and 29 regimens using the same dose of PfSPZ is needed to definitively confirm the benefit of the delayed third dose.
3.3.3. Moving from protection against homologous CHMI to protection against heterologous CHMI
Initial studies of heterologous protection conducted at Radboudumc (Nijmegen) in which relatively low doses of PfSPZ were administered by mosquito bite to immunize the participants, only a minority of participants protected against homologous CHMI were subsequently protected against a repeat CHMI using a heterologous parasite [Citation201]. However, higher PfSPZ doses in a trial conducted at the NIH Clinical Center by the LMIV team led by Agnes Mwakingwe-Omari and Sara Healy using PfSPZ-CVac (CQ), the best heterologous protection ever for a malaria vaccine was recorded. In this trial, PfSPZ Challenge was administered as 3 doses of 2 × 105 PfSPZ every 4 weeks using CQ as the partner drug according to the gold standard 13-visit regimen. This resulted in 100% VE against heterologous CHMI with Pf7G8 at 12 weeks (6/6 protected) [Citation68]. shows the remarkable reduction in transient parasitemia after each consecutive immunization. After one immunization, 3/8 and after two immunizations, 6/7 study subjects were already fully protected against the next dose of 2.0 × 105 PfSPZ. 2.0 × 105 infectious PfSPZ is 62.5-fold higher than the 100% infective dose of 3.2 × 103 PfSPZ (NF54). This indicates that not only is PfSPZ-CVac dose-sparing because of greater potency, it can achieve levels of VE higher than PfSPZ Vaccine can achieve at any dose (PfSPZ Vaccine has never achieved 100% protection against heterologous CHMI).
Figure 6. Parasitemia detected by qPCR after the first, second and third dose of 2×105 PfSPZ for PfSPZ-CVac (CQ) and PfSPZ-CVac (PYR). Median parasitaemia values and interquartile ranges are shown for positive PfSPZ-CVac (CQ) participants (no PfSPZ-CVac (PYR) participants were positive, since pyrimethamine kills the parasites during liver stage development). Dose 1, PfSPZ Challenge inoculation under CQ or PYR treatment cover with follow-up for 14 days; doses 2 and 3, PfSPZ Challenge inoculation under CQ or PYR treatment cover with follow-up for 10 days. The table shows (from left to right in each cell): the number of participants who were positive by qPCR/the number of injected participants; the median peak parasite density of positive participants (parasites per ml); and the mean day of peak parasite density (positive participants). Six PfSPZ-CVac (CQ) recipients went on to heterologous CHMI and 6/6 (100%) were protected; nine PfSPZ-CVac (PYR) recipients went on to heterologous CHMI and 7/9 (78%) were protected. ND, not detected; n/a, not applicable. Reproduced with permission from Nature (no changes were made) [Citation68].
![Figure 6. Parasitemia detected by qPCR after the first, second and third dose of 2×105 PfSPZ for PfSPZ-CVac (CQ) and PfSPZ-CVac (PYR). Median parasitaemia values and interquartile ranges are shown for positive PfSPZ-CVac (CQ) participants (no PfSPZ-CVac (PYR) participants were positive, since pyrimethamine kills the parasites during liver stage development). Dose 1, PfSPZ Challenge inoculation under CQ or PYR treatment cover with follow-up for 14 days; doses 2 and 3, PfSPZ Challenge inoculation under CQ or PYR treatment cover with follow-up for 10 days. The table shows (from left to right in each cell): the number of participants who were positive by qPCR/the number of injected participants; the median peak parasite density of positive participants (parasites per ml); and the mean day of peak parasite density (positive participants). Six PfSPZ-CVac (CQ) recipients went on to heterologous CHMI and 6/6 (100%) were protected; nine PfSPZ-CVac (PYR) recipients went on to heterologous CHMI and 7/9 (78%) were protected. ND, not detected; n/a, not applicable. Reproduced with permission from Nature (no changes were made) [Citation68].](/cms/asset/0003f74e-401a-47af-956a-5381a4fad986/ierv_a_2245890_f0006_oc.jpg)
3.3.4. Achieving VE against heterologous CHMI with a partner drug that prevents transient parasitemia and associated symptoms
In the same study [Citation68], LMIV investigators demonstrated that when a liver active drug, pyrimethamine (PYR), which prevents development of asexual erythrocytic stage parasitemia, was administered on days 2 and 3 after each dose of PfSPZ Challenge, there was 88% and 78% VE against homologous and heterologous CHMI at 12 weeks after last dose of vaccine (7/8 and 7/9 protected, respectively). Likely because PYR disrupts liver stage development rather than allowing normal liver stage growth and replication as does CQ, VE following administration of the same SPZ dose appeared lower than when CQ was used as the partner drug (although confidence intervals overlapped). However, as the vaccinees did not experience transient parasitemia with PfSPZ-CVac (PYR), adverse events on days 6–9 were minimal, and there was no need to administer NSAIDs. PfSPZ-CVac (PYR) is currently undergoing field testing in Mali (Sagara and Cook, unpublished [NCT03952650]) and may be a promising approach for further development.
Primaquine is another drug active against liver stage Pf and has also been studied, in a collaboration between the Seattle group and LMIV [Citation204]: immunizations were done by 12–15 mosquito bites administered three times at 4-week intervals combined with a 45 mg dose of primaquine base on days +2 or +3 after each immunization as the primary partner drug, with chloroquine administered as well for safety. As transient parasitemia still occurred in some study subjects, and VE was low, the approach has not been pursued further. In another approach, a single dose of liver-active atovaquone/proguanil (1,000 mg/400 mg) was administered just before each of three doses of PfSPZ Challenge (5.12 × 104 or 1.5 × 105 PfSPZ per dose in two separate groups) at a study conducted by ITM in Tübingen. Although there were no break-through parasitemias, protection against 10-week homologous CHMI was low (4/18 protected) [Citation168], and this approach has likewise not been pursued further. Finally, azithromycin stops parasite development late during the liver stages and has been successfully used in rodents [Citation205]. A single dose of 2 g extended-release azithromycin on the day of DVI led to blood stage parasitemia and the approach was therefore abandoned, as multiple dosings with azithromycin during immunization to prevent parasitemia was deemed impractical [Citation203].
3.3.5. The impact of parasitemia at time of immunization
When administering PfSPZ-CVac (CQ), it is important to avoid dosing with PfSPZ Challenge at the time of transient parasitemia resulting from an earlier dose. In the trial in Seattle, before achieving 78% protection after the 5-day interval regimen (days 1, 6 and 11; 1.024 × 105 PfSPZ/dose), a regimen utilizing 7-day intervals and a lower dose (5.12 × 104 PfSPZ) was tested (days 1, 8 and 15). This scheduled the second immunization during the period of transient parasitemia caused by the first (), and the third immunization during the period of transient parasitemia caused by the second. None of the seven participants undergoing homologous CHMI 3 weeks later was protected [Citation127], in contrast to the ITM trial in which three doses of 5.12 × 104 PfSPZ at five-day intervals protected 5/8 (63%) participants [Citation8,Citation203]. This abrogation of protection presumably resulted from the immunosuppressive effects of parasitemia on vaccine take [Citation127], discussed further below.
Figure 7. Parasite density estimated by qRT-PCR in participants in a PfSPZ-CVac (CQ) trial conducted at the Kaiser Permanente Washington Health Research Institute, Seattle. (A) Triangle symbols indicate the first, second, and third days of vaccine administration. The numbers above each peak of parasitemia display the number of persons positive with transient parasitemia divided by the total number of vaccine recipients for that dose of vaccine. Days are listed relative to the first dose of vaccine. Group 1 received a PfSPZ Challenge dose of 5.12x104 PfSPZ for each of three doses administered by DVI seven days apart. Group 3 received a PfSPZ Challenge dose of 1.024x105 PfSPZ for each of three doses administered by DVI five days apart. All participants underwent CHMI 10 weeks after the last vaccine dose. 7/7 participants became infected in group 1 (vaccine efficacy 0%) while only 2/8 participants became infected in group 3 (vaccine efficacy 75%). It is notable that administration of PfSPZ-CVac on a schedule where vaccine administrations #2 and #3 coincided with sub-microscopic blood-stage parasitemia (group 1) was associated with an absence of sterile protective immunity, whereas dodging parasitemia (group 3) appeared to restore the expected protective efficacy. Reproduced with permission from PLoS pathogens (no changes were made) [Citation127].
![Figure 7. Parasite density estimated by qRT-PCR in participants in a PfSPZ-CVac (CQ) trial conducted at the Kaiser Permanente Washington Health Research Institute, Seattle. (A) Triangle symbols indicate the first, second, and third days of vaccine administration. The numbers above each peak of parasitemia display the number of persons positive with transient parasitemia divided by the total number of vaccine recipients for that dose of vaccine. Days are listed relative to the first dose of vaccine. Group 1 received a PfSPZ Challenge dose of 5.12x104 PfSPZ for each of three doses administered by DVI seven days apart. Group 3 received a PfSPZ Challenge dose of 1.024x105 PfSPZ for each of three doses administered by DVI five days apart. All participants underwent CHMI 10 weeks after the last vaccine dose. 7/7 participants became infected in group 1 (vaccine efficacy 0%) while only 2/8 participants became infected in group 3 (vaccine efficacy 75%). It is notable that administration of PfSPZ-CVac on a schedule where vaccine administrations #2 and #3 coincided with sub-microscopic blood-stage parasitemia (group 1) was associated with an absence of sterile protective immunity, whereas dodging parasitemia (group 3) appeared to restore the expected protective efficacy. Reproduced with permission from PLoS pathogens (no changes were made) [Citation127].](/cms/asset/8bf782f3-c255-4951-9bfc-9921c5673e90/ierv_a_2245890_f0007_b.gif)
3.3.6. PfSPZ-CVac in malaria-exposed African adults
PfSPZ-CVac has been assessed in three trials in Africa. The first was a double-blind study in Malian adults led by Mahamadou Thera at MRTC and Matthew Laurens at the University of Maryland. Sixty-two participants were randomized to 2.048 × 105 PfSPZ of PfSPZ Challenge (NF54) or normal saline administered by DVI at 0, 4, 8 weeks with weekly CQ and then followed for 6 months including one rainy season. Drug clearance of any existing parasitemias prior to the first and last vaccine doses was not performed,Footnote8 although it is now understood that this is required for the induction of protective responses (see below). The vaccine was safe and well tolerated, without any evidence of symptoms relatable to transient parasitemia, but there was no significant protection against Pf infection, likely because there was no clearance of parasitemia prior to immunizations [Citation167]. Because qPCR was not attempted on days +7, +8, and +9 after each immunization as has been done in other studies, it is not known whether the participants experienced transient parasitemia, which could have been suppressed by naturally acquired immunity. In the second study, also in Malian adults, led by Issaka Sagara at MRTC and David Cook at LMIV, a larger dose, 4.0 × 105 PfSPZ, or normal saline was administered by DVI on the same schedule, and this time participants were cleared of parasitemia prior to first and last doses (NCT03952650). Pyrimethamine was selected as the partner drug, and was administered on the day of each immunization (75 mg) or on days +2 and +3 after each immunization (75 mg each day), both regimens that in malaria-naive adults receiving 2.0 × 105 PfSPZ of PfSPZ Challenge are sufficient to kill liver stage parasites and prevent parasitemia [Citation68]. One hundred and sixty-eight (168) participants were vaccinated with one of the PfSPZ-CVac (PYR) regimens or normal saline and followed over the first transmission season (and a subset over a second transmission season, some after receiving a single boost). Vaccinations were well tolerated, and efficacy results are pending [Sagara and Cook, unpublished]. In the third study conducted in Equatorial Guinea, 1.0 × 105 PfSPZ of PfSPZ Challenge (NF54) or normal saline were administered at approximately 4-week intervals, without presumptive treatment prior to any of the immunizations. Vaccinations were well tolerated and VE against homologous CHMI by DVI at a median of 13 weeks after vaccination was 55%, twice as high as a comparator group immunized with PfSPZ Vaccine receiving 27 times more PfSPZ (3 doses of 2.7 × 106 PfSPZ) [Citation126].
3.3.7. Next steps
The protection data from the trial of PfSPZ-CVac (PYR) in Mali will be important to understanding how VE against heterologous CHMI translates to the field. PfSPZ-CVac (CQ) is now being studied (in parallel with PfSPZ Vaccine) in the trial in Indonesian soldiers (NCT03503058), using the gold standard (for malaria-naive individuals) of three doses of 2.0 × 105 PfSPZ administered by DVI every 4 weeks along with 10 doses of chloroquine. It will be of interest to see if this most potent regimen is effective against the vaccine strain-divergent parasites found in eastern Indonesia. A second trial is being considered by ITM in Tübingen for 2023 to assess oral pyronaridine, an antimalarial that in the future could be given by parenteral injection. This is an effort to develop a PfSPZ-CVac regimen that assures adequate drug levels through IM injection thereby reducing the safety concerns associated with an orally administered partner drug.
3.4. Genetically attenuated (altered) SPZ
The goal with a genetically attenuated/altered (GA) parasites (GAP) is to develop a PfSPZ vaccine that has the tolerability and safety advantages of PfSPZ Vaccine and the potency/cost of goods advantages of PfSPZ-CVac, but does not require a drug partner, thereby circumventing the safety concerns associated with PfSPZ-CVac. This would be a parasite that can invade hepatocytes and develop to the late liver stage, but cannot produce merozoites to infect erythrocytes – a late liver stage-arresting replication competent (LARC) GAP [Citation169]. In addition, GAPs can be further genetically modified to express transgenes that enhance immunogenicity and genes from other human Plasmodium species to enhance cross-species protection.
3.4.1. Early arresting GAP
The first PfSPZ GA vaccines were phenotypically similar to irradiated PfSPZ, early arresting and replication deficient (EARD). They were metabolically active, but non-replicating. As with other whole SPZ approaches, proof of concept was obtained in rodent models, with the deletion of UIS3 and UIS4 [Citation172,Citation206,Citation207], P36/P36p (also known as P52) [Citation173,Citation208–210], B9 [Citation211,Citation212], and SLARP/SAP1 [Citation212,Citation213] by teams at the Seattle Biomedical Research Institute (now the Seattle Children’s Research Institute, SCRI) led by Stefan Kappe, Ashley Vaughan, and colleagues [Citation213,Citation214], Leiden University Medical Center (LUMC, The Netherlands) led by Chris Janse, Shahid Khan and colleagues, Radboudumc (Nijmegen) led by Robert Sauerwein and colleagues [Citation215] and the University of Heidelberg led by Ann-Kristin Mueller and Kai Matuschewski [Citation206].
Protection in mice was dependent on CD8 T cells and IFN-γ as with other whole SPZ approaches [Citation207,Citation216]. Orthologous Pf constructs were created by SCRI [Citation142,Citation217] and advanced to clinical testing at the Walter Reed Army Institute of Research (WRAIR) using mosquito bite administration. The first trial assessed a p36−/p52− double knock-out GAP (GAP2KO) [Citation142] administered by up to 200 mosquito bites. One of six research subjects receiving this dose developed parasitemia 12 days after immunization, indicating insufficient attenuation. The breakthrough parasite was later identified as the vaccine construct [Citation165]. Alternative EARD constructs have since been developed by SCRI, including a triple knock-out GAP (GAP3KO) with deletions in three genes (p36−/p52−/sap1−) [Citation218] that did not show breakthrough infections in 10 research subjects after administration via 150 to 200 mosquito bites in a clinical trial conducted in Seattle by the Center for Infectious Disease Research (formerly Seattle Biomedical Research Institute, now SCRI), the University of Washington, the Fred Hutchinson Cancer Research Center and NIAID [Citation166]. In a follow-on trial by the Kaiser Permanente Washington Health Research Institute in partnership with the same collaborators, when the GAP3KO was assessed for VE after immunizing participants with three or five sessions of approximately 200 mosquito bites, protection against homologous CHMI at 4 weeks was approximately 50% [Citation143]. SCRI and Sanaria considered the production of an injectable vaccine composed of aseptic, purified, cryopreserved p36−/p52−/sap1− parasites (Sanaria® PfSPZ-GAP3KO), but based on progress with LARC PfSPZ (see below), this has not gone forward to GMP production.
In parallel, LUMC (Leiden), Radboudumc (Nijmegen), and Sanaria collaborated to produce Sanaria® PfSPZ-GA1 Vaccine, composed of aseptic, purified, cryopreserved b9−/slarp− PfSPZ [Citation212]. PfSPZ-GA1 Vaccine showed no breakthrough infection after doses as high as 9.0 × 105 PfSPZ administered by DVI to 26 research subjects at LUMC and Radboudumc. However, only 3/25 research subjects immunized with three doses of 4.5 × 105 or 9.0 × 105 PfSPZ were protected against mosquito bite CHMI with homologous PfNF54 conducted 3 weeks after immunization [Citation146]. Because a comparator group immunized with PfSPZ Vaccine also showed poor VE, it is unclear how to compare the results of this trial with the many other trials in which PfSPZ Vaccine has shown high-level protection, but VE is likely similar for PfSPZ-GA1 and PfSPZ Vaccine, in keeping with their shared EARD phenotype of early developmental arrest.
3.4.2. Late liver stage-arresting replication competent (LARC) GAP
To exceed the protection afforded by the first-generation, EARD GAPs, LARC parasites have now been genetically engineered [Citation169,Citation219]. The plasmei2 knock-out emerged as the initial leading candidate [Citation145,Citation170], first developed by the Kappe lab at SCRI in the rodent malaria model [Citation220] and subsequently in Pf [Citation145]. LUMC (Leiden), in collaboration with Radboudumc (Nijmegen), has assessed plasmei2− parasites (called GA2) in humans using mosquito bite immunization in a trial led by Meta Roestenberg, Chris Janse, Blandine Franke-Fayard and Matthew McCall, and demonstrated safety, with no breakthrough infections in participants immunized 3 times by the bites of 50 infected mosquitoes (150 bites in total). The immunizations induced significantly higher protective immune responses compared to those achieved using similar mosquito-bite immunization with early arresting GA1 parasites [Citation147]. However, because the rodent malaria plasmei2− LARC GAP showed occasional breakthrough infection when high Py sporozoite doses were injected, the Kappe lab at SCRI decided to introduce a second deletion, of a gene called LINUP (liver stage nuclear protein) [Citation221]. The rodent malaria Py dual gene deletion (plasmei2−/linup−) double knockout GAP showed no breakthrough infection in mice (Goswami, unpublished) and the Pf plasmei2−/linup− double knockout GAP showed no breakthrough infections in a humanized mouse model (Goswami, unpublished). Sanaria and Kappe’s team at SCRI have used this gene-deleted parasite to develop a vaccine called PfSPZ-LARC2 Vaccine.
These aseptic, purified, cryopreserved plasmei2−/linup− PfSPZ will be administered by DVI and assessed for VE against CHMI with homologous and heterologous parasites and field transmitted Pf. PfSPZ-LARC2 Vaccine demonstrated complete attenuation when 1 × 106 PfSPZ were injected into FRG-huHep mice with humanized livers, five times the planned human dose (Goswami, unpublished). Studies in rodent models and humans indicate that LARC parasites should be more potent than EARD parasites [Citation144,Citation147]. It is anticipated that potency will rival that of PfSPZ-CVac (CQ) without the safety issues. LARC parasites also induce a degree of anti-blood stage immunity in mice [Citation144,Citation222].
3.4.3. Next steps
Planning for the first trials of PfSPZ-LARC2 Vaccine is underway, with the intention of assessing the vaccine in both malaria-naive and malaria-exposed individuals during the first year of testing. Two trials in malaria-naive adults, one in Tübingen at ITM and one in Seattle are designed to assess for breakthrough infections and for protection against 12-week heterologous CHMI using PfSPZ Challenge (7G8). An IND will be submitted to the FDA and an IMPD to the EMA in late 2023 or early 2024 to support these trials. The first trial in Africa will assess for breakthrough in adults and will then transition to an age de-escalation study in preparation for subsequent Phase 2 field trials in adults and children. This trial is planned as a collaboration between Groupe de Recherche Action en Santé (GRAS), the University of Maryland and Sanaria.
Box 2. Contributions of the International PfSPZ Consortium
The development of PfSPZ-based vaccines has been strongly collaborative, based on shared concern over the public health toll exacted by malaria, belief in the potential for vaccines to make a difference, and intrigue with the biology underlying immunity to parasitic protozoa. Funding has been largely through competitive grants, with NIAID, NIH the largest contributor. Other major funders include African governments (Equatorial Guinea, Tanzania), the US Department of Defense, foundations and oil and gas industries. The recently discontinued EU Malaria Fund also provided significant funding.
Collaborators are members of the International PfSPZ Consortium (i-PfSPZ-C), an informal association of scientists, physicians, public health officials, and funders that meets in person and/or by teleconference once or twice per year to share the most recent data, critique each other’s approaches, and brainstorm to determine the best pathways forward. Open discussion and shared data are hallmarks of the consortium, allowing members to optimally transform ideas and insights into vaccine progress. shows the in-person attendees of the most recent i-PfSPZ-C meeting, which took place in November 2022 in Seattle, Washington, U.S.A. provides key contributions to injectable PfSPZ development by consortium members. The clinical trials described in the listed publications have been sponsored by Sanaria, NIH, or University of Tübingen (cross-referencing Sanaria’s master files and INDs when appropriate).
Figure 3. The International PfSPZ Consortium. Seattle Children’s Research Institute hosted 289 researchers from 86 institutions from 28 countries in person and virtually at the International Plasmodium falciparum Consortium (i-PfSPZ-C) meeting held November 3–4, 2022, to present data and set research and clinical development strategies for PfSPZ-based vaccines and products.

4. Challenges to the development of PfSPZ vaccines
The challenges facing the development of PfSPZ vaccines can be divided conceptually into those relating to the intrinsic qualities of PfSPZ as an immunogen (‘biological challenges’) and those related to practical aspects of fielding a PfSPZ-based vaccine to improve public health (‘feasibility challenges’). From the outset, developers have been more concerned about the former, which include safety, as these intrinsic properties will ultimately determine the impact that can be achieved against malaria. The ability of PfSPZ to induce high level protective immunity is foremost in this category, and Sanaria and collaborators have focused most resources on defining the dose, route and regimen of immunization that maximize protection and on improving the PfSPZ platform by assessing different methods of attenuation, genetic alteration, and adjuvanting. However, feasibility will also affect success, and will grow in importance as PfSPZ vaccines approach licensure and deployment. Challenges in both categories are considered below.
4.1. Biological challenges
PfSPZ vaccines have achieved 90–100% VE against homologous or heterologous CHMI conducted 3 to 12 weeks after immunization in 6 clinical trials in the U.S.A. [Citation1,Citation68,Citation187], Germany [Citation8], Tanzania [Citation2], and Mali [Citation3]. No other malaria vaccine has provided this degree of protection. However, significant gaps remain, particularly with respect to the 48–57% VE seen in field studies in African adults. In the quest to improve field efficacy, two important questions stand out. One is how to successfully immunize residents of endemic areas for whom ongoing malaria infections and prior malaria-exposure seem to compromise their ability to respond robustly to PfSPZ vaccination. The second is to determine whether vaccines based on the West African parasite PfNF54 can protect against all Pf variants in nature as effectively as they can protect against Pf7G8. These factors – hyporesponsiveness to vaccination in malaria-exposed individuals, and the requirement for cross-strain immunity, may explain why, in four successful studies in adults in Mali and Burkina Faso, the best VE against Pf infection in each trial during the first 6 months after the last dose has never exceeded 61% for a primary endpoint [Citation3,Citation7,Citation65,Citation112] (Diawara unpublished [NCT03989102]). Although these represent the only data showing this level of protection against Pf infection in adults induced by any malaria vaccine, protection is lower than the developers’ (and WHO’s) target of 90%.
4.1.1. Hyporesponsiveness to PfSPZ vaccines in Africans living in malaria endemic areas
Most of the infections for which vaccines are available are acute diseases that naturally induce long-term (in some instances life-long) immunity. However, if a vaccine-preventable infection has a chronic phase, vaccination in the presence of chronic infection is often not effective, as illustrated by hepatitis B [Citation223,Citation224]. Parasitic diseases generally follow this rule, modulating the host immune system so that it cannot achieve parasite clearance. For malaria, this impacts both malaria-specific antigens [Citation225] as shown by the poor responses to PfSPZ Vaccine in African adults () and non-malarial antigens, such as those in vaccines targeting other diseases [Citation226–228]. This hyporesponsiveness can additionally compromise the ability of the immune system to control concurrent infections with other pathogens [Citation229,Citation230].
Mechanisms of immunomodulation in malaria are diverse [Citation231]. Some malaria epitopes are immunodominant [Citation232], causing other, potentially protective epitopes to remain cryptic [Citation233,Citation234], and other epitopes serve as altered peptide ligands inducing downregulatory responses [Citation231,Citation235,Citation236]. Malaria infections downregulate Kupffer cell surface expression of MHC class I molecules [Citation237], decrease myeloid dendritic cell expression of HLA-DR [Citation238], exhaust B cells [Citation239,Citation240] and CD8 T cells [Citation241], inhibit NK cells [Citation242], reduce the frequency of parasite-specific CD4 cells [Citation243], increase the frequency of regulatory T cells [Citation244,Citation245] and inhibit dendritic cell function through uptake of the pigment hemozoin [Citation246,Citation247]. Many immunosuppressive processes are brought into play by deposition of SPZ in the skin, as reviewed in [Citation180]. Although the interplay of these mechanisms is not well understood, the net effect is that natural exposure to malaria infection rarely, if ever, leads to sterile immunity, meaning the ability to clear all invading sporozoites/liver stages with no development of parasitemia despite ongoing exposure. As evidence, if those with lifelong exposure are cleared of parasitemia through drug treatment, they promptly reacquire the infection if re-exposed [Citation77,Citation78,Citation248]. Malaria apparently causes a double hit to the immune system, the first being the immunosuppressive properties of parasitemia, and the second the fact that even in those cleared of parasitemia, there is a residual anergy to plasmodial proteins.
In this setting, a vaccine has to induce immunity that is more potent than that induced naturally. However, even if vaccination could induce sterile immunity to new infections in a chronically infected individual, it would not achieve full benefit for the individual (keeping them free of the infection) if the current infection remains untreated. Anti-disease vaccines have undisputed value, but the best outcome is preventing infection altogether, thereby maximizing the recipient’s health and halting further transmission, the latter a critical step to containment (e.g. in areas with emerging drug resistance), to sustained effects on incidence, and to (ultimately) malaria elimination. Therefore, developers of vaccines against parasites are faced with the need to treat existing infections when immunizing persons living in endemic areas, although this need will diminish progressively as control efforts reduce the parasite burden. In addition to freeing the individual from infection, treatment is intended to reduce the inhibitory influence of parasitemia on the immune system. The optimal timing of such treatment is yet to be determined; in particular, it is not known how long an individual needs to remain parasitemia-free for the immune system to fully recover, if indeed full recovery is possible.
Immunomodulatory effects of parasitemia on malaria vaccine potency. The ability of parasitemia to inhibit the response to sporozoite and liver stage antigens was first shown in rodent models [Citation249,Citation250] and seems true for humans as well, as shown by the beneficial effect of using drugs to clear any existing parasitemias before immunization. This approach was taken in several of the completed field trials of PfSPZ vaccines conducted in African adults and children, seven of these assessing PfSPZ Vaccine and two assessing PfSPZ-CVac. In five of these nine trials, parasitemia was cleared from all trial participants before the first vaccine dose, and statistically significant protection was recorded in all five trials, four of which are published [Citation3,Citation7,Citation65,Citation112]. In the four trials where clearance was not performed, protection was not observed using the pre-specified endpoint [Citation112,Citation164,Citation167] (Agnandji unpublished [NCT03521973]). Based on this evidence, Sanaria anticipates stating in the package inserts for PfSPZ vaccines that recipients who live in malaria-endemic areas will require pre-treatment before immunization. The current practice is to require a one- to two-week window between treatment and immunization, but longer intervals could lead to better results by allowing the suppressive effects of parasitemia on the immune system to wane. This hypothesis is based on preliminary results that clearance immediately before vaccination may not be adequate in adults who are parasitemic (Diawara, unpublished [NCT03989102]) or in children (Sagara unpublished [NCT04940130]). Longer intervals between pre-treatment and vaccination run the risk that individuals might re-acquire infections while awaiting vaccination, presenting a quandary for vaccination programs. This could be solved in areas with seasonal malaria, such as the Sahel, by clearing individuals at the end of the transmission season, providing several months for the immune system to recover, and then vaccinating prior to the start of the next transmission season. For areas with perennial transmission, institution of chemoprophylaxis for a period of time might be needed.
The negative impact of Pf parasitemia on the VE of PfSPZ vaccines has also been seen outside of Africa in studies of PfSPZ-CVac (CQ). In a trial in Tübingen that was previously discussed, when administered at 5-day intervals, 3 doses of 5.12 × 105 PfSPZ of PfSPZ Challenge protected 63% (5/8) subjects against homologous CHMI at 10 weeks after the last dose of vaccine, which was lower than the 100% VE seen when the same dose was administered at 4-week intervals [Citation8,Citation203], indicating that condensed regimens were less effective than regimens of longer duration. A study in Seattle was then undertaken to determine if increasing the dose of PfSPZ in the 5-day interval regimen would restore VE to 100% [Citation127]. The first step was to repeat the work from Tübingen. However, for practical reasons, the interval between doses was changed from 5 days to 7 days. When the subjects underwent homologous CHMI at 10 weeks, VE was 0%, compared to 63% in Tübingen, even though vaccinees received three immunizations with the same dose. Because the PfSPZ-CVac approach using CQ as the partner drug caused transient parasitemias on days 6–8 after immunization, the seven-day interval meant that the second and third doses were administered during the transient, subpatent parasitemia caused by the first and second doses. Injection of PfSPZ during these periods of parasitemia appears to have abrogated protection.
To test this hypothesis, the immunization schedule in the Seattle trial was adjusted back to the 5-day intervals used at the ITM in Tübingen, so that PfSPZ were injected before the transient parasitemia from the preceding dose (). In addition, the dose was increased to 1.024 × 106 PfSPZ per injection. These seemingly minor changes restored protection from 0% to 75% against homologous CHMI 10 weeks after immunization. The parasite densities to which the PfSPZ were exposed using the 7-day interval were low (46 to 8929 parasites/mL by qRT-PCR, mostly below the limit of detection by thick blood smear), yet the resulting inhibition of vaccine effect appeared to be profound. The mechanism by which parasitemia appears to have abolished VE in the Seattle trial is an important area for further investigation.
Anergy with respect to malaria antigens. A second immunomodulatory effect can be seen in studies of PfSPZ Vaccine in Africa in which pre-treatment was given. Even with clearance, immune responses remained far below those of malaria-naive counterparts in the U.S.A. [Citation3,Citation7,Citation65,Citation112,Citation129] (Diawara unpublished [NCT03989102]) (see paragraph K in Section 3.2). Potential approaches to overcoming this problem include the use of adjuvants [Citation251], the inclusion through gene editing of immunostimulatory transgenes into the vaccine [Citation252], prime and trap approaches to immunization [Citation55,Citation251], or altering the stage at which antigens are expressed (i.e. expression on the sporozoite surface as opposed to in the hepatocyte) [Citation253]. It is also possible (as mentioned above) that in regions with a long dry season such as the Sahel that residual parasitemia could be treated months before the beginning of the rainy season, without risk of reinfection before immunization, providing a long rest period without malaria exposure, which could promote immunological recovery and increase immune responsiveness to the vaccine. This hypothesis urgently needs testing, given the potential of this simple step to significantly boost VE.
4.1.2. Cross-strain protection
The second biological challenge discussed here is the antigenic diversity of Pf in the field. In geographic regions where intensity of malaria transmission is high, the malaria parasite populations infecting humans are panmictic, continuously recombining the greater than 5000 genes in the genome [Citation254]. Multiplicity of infection in the blood of a single individual is common [Citation255,Citation256], each clone potentially reproducing sexually with the others in the mosquito midgut if gametocytes from more than one clone are imbibed together. Moreover, many parasite proteins are polymorphic, including pre-erythrocytic stage proteins that are important vaccine candidates, such as CSP [Citation257]. While the most variant gene families are expressed by blood stages, some variant families, such as STEVOR proteins, are expressed by sporozoites [Citation258]. The challenge presented by antigenic diversity has been emphasized by vaccine developers [Citation259] and regulatory agencies [Citation260], documented through genetic and epitomic analyses [Citation110] and leaves open the question whether products, such as PfSPZ Vaccine, based on a single West African strain of Pf, will be effective across Africa or outside the African continent.
Although this question remains unanswered, the developers of PfSPZ vaccines believe that immunization induces immune responses against perhaps 100s to 1000s of protective epitopes, and thus should provide protection against the diverse strains of parasites in Africa. The protection seen against CHMI with Pf7G8, a Brazilian isolate more genetically distant from the PfNF54 vaccine strain than any of the 704 Pf isolates from across Africa that were included in a comparative study [Citation111], supports this contention. In addition, variability in the sporozoite and liver stages may be less than in the blood stages, where most of the proteins from variant gene families are expressed. Furthermore, the consistent VE results in adults from two different countries, Mali, and Burkina Faso, are encouraging, and are as good as or better than the protection seen against heterologous CHMI in malaria-naive adults [Citation111]. Genome-wide sieve analyses of the parasite genotypes of incident infections in vaccine and control groups are currently underway as an approach to better understand antigenic escape.
The concern of parasite variability is being addressed in the clinical trial of PfSPZ Vaccine and PfSPZ-CVac in malaria-naive Indonesian soldiers who have been immunized in a malaria-free area and then deployed to a malaria endemic area where both Pf and Pv are heavily transmitted. This trial (NCT03503058) will assess the efficacy of PfSPZ Vaccine and PfSPZ-CVac (CQ), both based on West African PfNF54 parasite, against the highly divergent Pf parasites of New Guinea [Citation110]. If strong protective efficacy is seen, it will indicate that a universal Pf vaccine based on PfSPZ is feasible. If not, the finding will emphasize the need for developing hybrid strains, vaccines combining strains from different parts of the world, or regional immunization approaches.
As more and more potent vaccines and vaccination regimens are developed, cross-strain protection should improve. This is the context in which to view the minimal cross-strain protection recorded at Radboudumc (Nijmegen) using CPS (CQ) after mosquito-bite immunization with doses of PfSPZ that were highly protective against homologous CHMI [Citation201,Citation261]. Whereas these studies suggested limited cross-strain protection, dose escalation using injectable PfSPZ Challenge in the PfSPZ-CVac approach at LMIV significantly improved cross-strain protection [Citation68]. Heterologous strains other than Pf7G8 now need to be tested, such as strains isolated from Southeast Asia. These strains are being developed at Sanaria.Footnote9 If cross-strain immunity is not adequate, options remain for regionally targeted vaccines or for developing multi-strain or hybrid vaccines to broaden cross-strain protection. Genetic knock-in of antigens may also be used to strengthen cross-protection as described in Section 5 below.
4.1.3. PfSPZ safety and tolerability
No characteristic of PfSPZ vaccines will be more important to their success than safety and tolerability. Asexual blood stage Pf parasites are pathogenic, especially at high densities, causing the febrile syndrome ‘malaria,’ and if not treated with antimalarial drugs can be fatal to infants, young children, and pregnant women living in endemic areas and to malaria-naive individuals of any age. In contrast, there appear to be no signs or symptoms caused by SPZ deposition in the skin (other than the reactogenicity of mosquito saliva), by their journey through the blood stream, or by their development in hepatocytes. Fortunately, these aspects of malaria biology have been borne out in clinical studies of PfSPZ vaccines, which have been safe and well tolerated even when injected at doses as high as 2.7 × 106 PfSPZ [Citation7,Citation126], doses which correspond to several thousand infectious mosquito bites [Citation176]. As PfSPZ vaccines enter Phase 3 testing in the years ahead, careful monitoring will need to continue so that any uncommon but important side effects can be identified. Excellent tolerability, which at this point appears to be intrinsic to the SPZ platform, will be critical to the success of mass vaccination programs.
4.2. Feasibility challenges
The need to address feasibility challenges is growing in importance as Sanaria and collaborators move closer to licensure and deployment. Can PfSPZ vaccines be manufactured at scale and at an affordable cost? Can they be distributed effectively in LNVP and administered to the target populations using DVI?
As described in Box 1, the manufacturing processes required to produce aseptic, purified PfSPZ that meet regulatory standards are almost entirely novel. They include the production of aseptic mosquitoes, the feeding of these mosquitoes on gametocytemic blood cultures to generate the PfSPZ, manual harvest of salivary glands followed by filtration to purify the PfSPZ, and cryopreservation and storage in LNVP for stabilization. In-process and release testing (and stability testing) combine standard USP assessments with novel assays for viability and attenuation. Describing the details of these procedures is beyond the scope of this review. However, several key aspects of production, cryopreservation, and vaccine deployment raise questions regarding feasibility and are addressed here. These questions are particularly relevant to the principle of social justice requiring fair and equitable vaccine distributions.
4.2.1. Manufacturing at scale and at an affordable cost
While all processes used in manufacturing of PfSPZ are theoretically scalable, there is concern regarding cost of goods (COGs) and the ability to meet the world’s needs for a malaria vaccine, which could involve hundreds of millions of regimens per year if, for example, all pregnant women at risk or all children are targeted (see Applications section below). To address this concern, Sanaria plans to transition to in vitro production of PfSPZ (iPfSPZ), eliminating mosquitoes from the manufacturing process. Recent advancements with this technology were published in Nature in 2022 [Citation9]. iPfSPZ invade human hepatocytes in culture and develop to mature liver-stage schizonts expressing Pf merozoite surface protein 1 (PfMSP1) in numbers comparable to mosquito-produced PfSPZ (mPfSPZ). When injected into FRGhuHep mice containing humanized livers, iPfSPZ invade the human hepatocytes and develop to PfMSP1-expressing late liver stage parasites at 45% of the quantity of cryopreserved mPfSPZ. In a key study, human blood from FRGhuHep mice infected with iPfSPZ produced asexual and sexual erythrocytic-stage parasites in culture, and gametocytes developed to PfSPZ when fed to mosquitoes, completing the Pf life cycle from infectious gametocyte to infectious gametocyte without using mosquitoes [Citation9]. Sanaria is now striving to optimize iPfSPZ production and adapt it to rigorous GMP standards. Once these are established, Sanaria anticipates manufacture at least 100 billion PfSPZ in a refrigerator-sized incubator over the course of a month. If the dose of PfSPZ-LARC2 Vaccine is 2 × 105 PfSPZ, that would be 500,000 doses from a single incubator in a month or 6 million doses per year. It is also expected that the technology of iPfSPZ production will be fully transferrable, allowing production in African or Asia-Pacific countries where the need is greatest.
Sanaria estimates that the cost of iPfSPZ will be about 10% of mPfSPZ. The company envisions a profitable traveler’s market with the price of the vaccine matching that of other travel vaccines to balance a low cost for endemic countries.
4.2.2. Cryopreservation, storage and distribution in LNVP
Stabilization in LNVP (below −135°C, the glass transition temperature of water, Tg) is an absolute requirement for most eukaryotic cells, and for human injectables the recommendation is below −150°C [USP <1044>] [Citation262]; there is no prospect of PfSPZ storage other than at cryogenic temperatures. PfSPZ distribution cannot, therefore, be slotted into an existing standard 2–8°C cold-chain such as is used for the Expanded Programme on Immunization. However, PfSPZ vaccines are not the first vaccine to have this requirement. Deployment of mRNA vaccines for SARS-CoV-2 as well as focused delivery of the Ebola vaccine Ervebo to outbreak sites in Africa required a novel −80°C to −60°C cold chain [Citation263], evidence that public health systems across the world can accommodate this approach when combatting deadly contagious diseases. As described previously, the sporozoite-based Theileria parva (East Coast Fever) vaccine for cattle has been distributed in Africa for decades using a liquid nitrogen cold chain that is specific for this vaccine and pathogen [Citation264].
LNVP distribution of a PfSPZ vaccine has been modeled in collaboration with Johns Hopkins University for Tanzania, a representative malaria-endemic country which has strongly supported the development of PfSPZ-based vaccines (see Box 2): Capital and recurrent costs for storage, transportation, labor, energy usage, and facilities were determined for the birth cohorts in Tanzania over 5 years. Costs were calculated using WHO/UNESCO calculators applied both to a 2–8°C distribution model with national, regional, district, and health facility levels, and for a cryopreserved PfSPZ vaccine using a ‘modified hub-and-spoke’ LNVP distribution system comprising a central national store, peripheral health facilities and one intermediate district-level trans-shipment stop. The system for PfSPZ distribution generated costs comparable to those of the 2–8°C cold chain over the projected 5-year campaign [Citation115], meaning that expenses of a liquid nitrogen cold chain were comparable to costs for existing cold chains.
A LNVP cold chain may have advantages over cold chains requiring refrigeration. Traditional cold chains rely on electricity and a series of refrigeration units at each node and within each transport vehicle. This places particular strain at the periphery of the cold chain where electrical supply is often erratic or may be absent. Refrigeration units require maintenance, calibration, and continuous temperature monitoring to ensure the tight 2–8°C thresholds are met, and breakdowns are not an infrequent occurrence. Vaccine wastage rates are frequently significant and often the result of freezing damage [Citation265]. In contrast, other than liquid nitrogen production units at key central locations (which already exist across Africa to support many industries), the LNVP cold chain requires no electricity and no container units with moving parts, unlike refrigerators. Dry shippers have known hold times and their refrigerant status can be assessed by weighing the container. Payload temperature remains within specification (below −150°C) if liquid nitrogen is present in the dry shipper. In the hub and spoke model, dry shippers are taken all the way to the end user (vaccination clinic, health worker’s office) where they can remain for extended periods, while immunizations are being conducted. Liquid nitrogen replenishment is performed using Dewars maintained at strategic locations. Although vaccine loss can certainly occur in the LNVP system as well as in traditional systems, the independence from electricity, the reduced number of intermediate stops and standardized timing for refrigerant recharging (determined by weight) make the LNVP cold chain more predictable and more efficient. Failures are common for 2–8°C cold chains [Citation266], while lower wastage rates are anticipated for LNVP cold chains. The ongoing concern over traditional cold-chain failures has sparked a movement toward so-called ‘passive cold storage devices.’ Many of these are actually similar to LNVP dry shippers but are designed for other temperature ranges and use different refrigerants [Citation267].
In addition to using the LNVP cold chain in all clinical trials, Sanaria has also tested the LNVP cold chain at several travel medicine and military immunization clinics in the U.S.A. (James, unpublished data). Clinic personnel were trained in the receipt, maintenance on-site, and use of dry shippers with liquid nitrogen delivered on a regular schedule. They conducted simulated vaccine dose retrievals and cryovial thawing, maintained inventory records and monitored vaccine stock temperatures using data loggers. Personnel quickly became adept at handling all operational components and no wastage occurred during each of the four 4-week trials. There will be a need to supply cryopreserved PfSPZ vaccines to clinics and dispensaries that vary considerably in the numbers of doses required per day or week and there exists a range of dry shippers and packaging with a range of capacities to satisfy these requirements.
4.2.3. Direct venous inoculation
Intravenous administration is the most efficient and consistent route for inducing infection using PfSPZ Challenge and for immunization using PfSPZ vaccines, likely because it maximizes the opportunity for the injected PfSPZ to reach the liver sinusoids, invade hepatocytes, and develop to blood stages (if non-attenuated) or induce protective immunity in liver resident lymphocytes (if attenuated). Initial intravenous injections were done using a pre-placed catheter [Citation1,Citation4,Citation5,Citation8] but the clinical teams conducting trials sponsored by Sanaria soon transitioned to DVI as an easy, rapid, well tolerated method for IV administration. DVI eliminates the second, more painful phase of IM, SC, or ID injections (needle insertion being the first, injection of fluid disrupting tissue planes being the second), and because the needle diameter is 25-gauge, insertion is minimally painful. Furthermore, since only 0.3 to 0.5 mL volumes are injected, the process takes only seconds. The majority of DVI recipients subjectively characterize the procedure as causing ‘minimal pain’ or ‘no pain.’ For example, in a study of Tanzanian adults, 97% of 237 injections were rated as causing ‘no pain’ using a 4-point scale that included no, mild, moderate, and severe pain as options [Citation124]. In a subsequent study using the same subjective scale involving 6- to 45-year-old Tanzanians, 97% of 161 injections were classified as ‘mild pain’ or ‘no pain’ [Citation136]. In a study of adults from Equatorial Guinea, 91/96 injections (95%) were rated as causing ‘no pain’ and 5/96 (5%) as causing ‘mild pain’ [Citation162]. In a study of infants in Kenya, 16.1% of the children slept or nursed through the procedure without crying [Citation138], indicating that even in this sensitive population the pain induced was minimal in many infants. Regarding the technical skill needed to perform DVI, clinical teams state that it is equivalent to the technical skill needed for any blood draw. Since these often constitute the same personnel who administer vaccines, most medical personnel who use syringes already possess the required skills.
The drawback of DVI becomes apparent in those with few superficial veins and in small children and infants whose veins may be hidden by subcutaneous fat – the identical population for which any blood draw is difficult. A higher level of skill is needed for this population. Feasibility was assessed in a large study of Kenyan infants. The physicians doing the injections showed different levels of competence with the procedure, and a learning curve was apparent. A near infrared light device was used for many injections to assist in locating the best veins. The principal investigator, Martina Oneko from the Kenya Medical Research Institute, concluded in her published report that ‘Administration by DVI is feasible in infants and young children with a phlebotomy team composed of skilled injectors. Ultimately, more than 90% of vaccinations could be administered to infants with a single injection. DVI should therefore be considered an acceptable route for vaccination if it provides a higher level of protection than more standard routes of administration’ [Citation138].
In this early era where the supply of PfSPZ is limited by the complexity of the manufacturing process, DVI has been critical to the development of PfSPZ vaccines, as it is dose sparing compared to other routes of administration. However, intramuscular (IM) injection is also protective using larger doses of PfSPZ [Citation4]. When in vitro production removes the restrictions on PfSPZ supply, it is possible that alternative routes of administration such as IM can be considered for those populations with limited venous access or indeed for all individuals.
5. Vaccines based on rodent malaria SPZ expressing Pf antigens
This approach to SPZ vaccination is banking heavily on cross-species protection. It has been demonstrated multiple times that natural infections with one species of human malaria parasite provide a degree of protection against a second species, although it is not sterile immunity (reviewed by [Citation268]). These findings are consistent with murine models, in which vaccination with one murine species can provide protection against a second [Citation210,Citation269–273]. Investigators at the University of Lisbon led by Miguel Prudêncio have developed an SPZ vaccine based on the murine malaria P. berghei hypothesizing that adequate doses will cross-protect against Pf. The parasite has been genetically altered to express the Pf circumsporozoite protein (CSP) on the PbSPZ surface to boost the chance of success [Citation274]. The vaccine, called PbVac, was recently studied in a dose-escalation trial conducted at Radboudumc in Nijmegen. The high-dose group (n = 12) received four immunizations with 75 mosquito bites at four- to eight-week intervals followed by Pf CHMI via five mosquito bites 3 weeks after the last vaccine dose. Although there was no sterile protection at the dose tested, significant delays in patency (2.2 days, P = 0.03), and decreased parasite density were observed, corresponding to an estimated 95% reduction in Pf liver parasite burden (P = 0.010) [Citation275]. The question thus remains open whether, through ongoing optimization, such a cross-species vaccine can induce sterile protection against Pf and other human malaria species.
6. PvSPZ vaccines
As with Pf, immunization of humans by mosquito bite with irradiated PvSPZ in the 1970’s demonstrated the induction of sterile immunity, albeit only a few volunteers were studied [Citation20,Citation25]. One volunteer was immunized by mosquito bite with PfSPZ, protected against heterologous PfCHMI, further immunized with 539 bites by mosquitoes infected with PvSPZ (Chesson strain) and protected against PvCHMI 2 weeks later (Chesson strain). A second volunteer without prior PfSPZ immunization was immunized with 728 bites by mosquitoes infected with PvSPZ (El Salvador strain), was not protected on PvCHMI 1 week later (El Salvador strain), was further immunized with 1,251 bites by mosquitoes infected with PvSPZ (Chesson strain), underwent three PvCHMI’s with Chesson strain at 1 week (protected), 23 weeks (not protected) and 27 weeks (protected), and then at 40 weeks underwent PvCHMI with El Salvador strain and was protected. These anecdotal data from irradiated PvSPZ immunization are consistent with the larger data sets available for irradiated PfSPZ immunization, indicating that PvSPZ immunization should be protective against Pv.
A trial of irradiated PvSPZ immunization in humans was conducted in Cali, Colombia, by Socrates Herrera and colleagues at the Malaria Vaccine and Drug Development Center (MVDC) and Universidad del Valle [Citation276]. Because of the difficulty of generating multiple sequential batches of Pv-infected mosquitoes from fresh blood obtained from Pv-infected patients, only a median of 434 infective bites was achieved for each immunized volunteer. PvCHMI was performed 8 weeks after immunization and 5/12 (42%) immunized research subjects were protected compared to 0/2 non-immunized controls. This level of protection was about the same as for PfSPZ after this few immunizing bites, supporting the contention that the same high levels of efficacy achieved against Pf using larger PfSPZ inoculations should translate to high-level efficacy against Pv using larger PvSPZ inoculations.
PvSPZ vaccine development will need to address the arrested Pv liver stages (hypnozoites) that resume development weeks to months to more than a year after primary infection, causing relapsing malaria [Citation277,Citation278]. At this point, there are no published data to indicate whether PvSPZ-induced immunity can block the development of hypnozoites and thus prevent relapsing Pv, although recent studies with monoclonal antibodies targeting PvSPZ indicate that this is possible [Citation279]. This question is paramount as up to 80–90% of new Pv parasitemias may be relapses [Citation280,Citation281].
The challenges of manufacturing PvSPZ at scale are greater than for Pf. Pv blood stages require reticulocytes as the host cell and culturing the parasite is difficult. To circumvent this problem of how to feed the mosquitoes, researchers have used fresh blood from Pv-infected humans as was done in Colombia or from specific pathogen-free (SPF) Pv-infected Saimiri monkeys as is being pursued at Sanaria under FDA oversight. It is anticipated that the Pv-infected Saimiri monkeys certified as SPF using FDA-approved testing should allow Sanaria to produce sufficient aseptic PvSPZ to supply research institutions across the world with parasites for PvCHMI, assuming that no more than a few thousand PvSPZ will be needed to induce infection. Production in SPF Saimiri monkeys may also allow Sanaria to produce PfSPZ-CVac for initial clinical trials, but it will be difficult to generate enough PvSPZ for an irradiated PvSPZ Vaccine.
7. Applications for PfSPZ vaccines
Sanaria and its partners in the International PfSPZ Consortium are currently targeting multiple malaria-susceptible groups for implementation of PfSPZ vaccines (). In the short to medium term, the goal is to use PfSPZ vaccines for prevention of malaria in travelers to malaria endemic areas and in women of childbearing potential (WOCBP), pregnant women and children living in malaria endemic areas. An initial focus will be seasonal malaria prevention in the African Sahel. The long-term goal is to use PfSPZ vaccines as the central intervention in geographically focused malaria elimination programs, including efforts to eliminate foci of drug resistant Pf, since preventing blood stage infection makes the vaccine a VIMT (vaccine to interrupt malaria transmission).
Figure 8. Target populations for PfSPZ vaccines. The objective for PfSPZ vaccine development is high-level prevention of Pf infection. Major risk groups potentially benefiting from such a vaccine are listed. It is anticipated that licensure will occur first in the EU with an indication to prevent malaria in travelers (including travelers who travel within their own country from areas without malaria to areas with malaria), with licensure in the U.S.A. and malaria-endemic areas thereafter. Major risk groups are identified. The ultimate objective is use in MVPs to achieve focal and regional elimination.

7.1. Protection against malaria in individuals
7.1.1. Travelers, including residents of countries with malaria who travel from malaria-free regions to malaria-endemic regions
The protection recorded in malaria-naive adults in the U.S.A. and Germany point to an important group that could benefit from vaccination: malaria-naive residents of non-endemic areas traveling to endemic areas. This includes both international travelers and residents of non-malarious areas of malaria-endemic countries traveling domestically to endemic areas. Deploying military personnel have similar needs and represent another important target population (and market sector) although duration of protection suitable to cover a long military deployment needs to be demonstrated. Licensure for such travelers in the U.S.A. or EU would provide reassuring evidence of vaccine quality and safety before licensure in endemic areas. A trial of PfSPZ Vaccine in malaria-naive adults using heterologous CHMI to assess vaccine efficacy has been under way during 2022 in the U.S.A. (NCT04966871), although it is possible that PfSPZ-LARC2 Vaccine will prove more protective at lower doses and supplant PfSPZ Vaccine for a traveler’s indication.
7.1.2. Women of childbearing potential and pregnant women
The need for a vaccine to prevent pregnancy malaria is acute, as this disease is responsible for an estimated 200,000 stillbirths [Citation282] and nearly a million low birth weight babies [Citation283] per year, the latter a strong risk factor for neonatal and childhood mortality. Efforts to develop such a vaccine have been reviewed recently and include targeting VAR2CSA, the Pf protein responsible for parasite sequestration in the placenta [Citation195–197]. PfSPZ Vaccine may be ideal for preventing pregnancy malaria, given its safety, administration in a condensed 4-week regimen (to induce protective responses as early in pregnancy as possible) and the long-lasting protection afforded by liver resident memory CD8 T cells [Citation83]. Of note, PfSPZ and VAR2CSA vaccines could serve complementary roles, the former preventing parasitemia, and the latter reducing the ability of any breakthrough blood stage infections to cause harm to the mother and fetus. In addition to immunizing pregnant women, pre-pubertal girls and/or non-pregnant women of childbearing age could be immunized with a primary vaccination series and then boosted during pregnancy. A study has been completed in Mali by LMIV and MRTC in WOCBP led by Halimatou Diawara, Alassane Dicko, Sara Healy, and Patrick Duffy that showed PfSPZ Vaccine safety, favorable tolerability, and efficacy against naturally transmitted malaria over the 6-month rainy season as the primary efficacy outcome [Citation112] (Diawara unpublished [NCT03989102]). More than 150 of the women in this trial became pregnant after immunization, and safety and VE data are now being collected to compare pregnancy outcomes between vaccinees and controls. VE is being assessed across rainy seasons in two successive years without a booster. The first Phase 1 trial assessing safety of PfSPZ Vaccine in pregnant women is planned by LMIV and MRTC for 2024 as described earlier. The success of condensed regimens, which could achieve rapid induction of immunity early in pregnancy, supports the proposed use of this vaccine for preventing pregnancy malaria.
7.1.3. Children
Children bear the greatest burden of morbidity and mortality from malaria. With the advent of better malaria control, the burden has been shifting from infants and very young children to older children [Citation284,Citation285]. In addition, the largest contribution to transmission in an African community is usually from children. Thus, children aged 2–10 are an important target group. A subset of these children is those living in the Sahel, where malaria transmission is strongly seasonal. Currently, seasonal malaria chemoprophylaxis (SMC) using drugs such as sulfadoxine – pyrimethamine plus amodiaquine is recommended by WHO during the rainy season in pre-school children living in this region [Citation286] and a PfSPZ vaccine could serve as a substitute if highly efficacious or could augment the protection afforded by SMC [Citation287]. A trial of PfSPZ Vaccine in 6- to-10-year-olds is under way in Mali as described previously (NCT04940130), with plans to study PfSPZ-LARC2 Vaccine in 2024 in an age de-escalation study also aiming for efficacy in children. Absence of protection seen in a trial of children in Gabon where no clearance was provided (Agnandji, unpublished) and the limited protection seen in infants in western Kenya, where clearance was likewise not done [Citation164], suggests that the issue of clearance pre-immunization may be particularly important for children. At present, other than the transient protection seen at 3 months in the trial of Kenyan infants [Citation164], there has been no demonstration of protection in children by PfSPZ vaccines.
7.1.4. Refugees and other populations with disrupted health services or in emergency situations
These are additional vulnerable groups in which PfSPZ vaccines could provide protection against malaria, which often occurs as an outbreak in displaced populations [Citation288]. Additionally, those with underlying medical conditions, such as sickle cell disease or HIV could benefit from immunization. PfSPZ Vaccine has been tested in a small group of HIV positive Tanzanian adults, and although it was safe and well tolerated, vaccination did not protect against CHMI conducted using homologous PfNF54 3 weeks after immunization (Jongo, in press, Journal of Clinical Investigation [NCT03420053]), indicating the need to use PfSPZ vaccines (or regimens) with enhanced potency in the immunocompromised.
7.2. Malaria elimination campaigns
The ability to protect against blood stage infection and thus prevent the development of gametocytes classifies PfSPZ vaccines as vaccines to interrupt malaria transmission (VIMTs). The development of such vaccines has recently been prioritized by WHO [Citation289]. Sanaria and collaborators’ ultimate objective is to use PfSPZ vaccines in mass vaccination programs (MVPs) in which all residents of a geographically defined area would be targeted for immunization as part of a comprehensive malaria elimination strategy including vector control and effective surveillance and case management. The use of MVPs might be particularly helpful in settings that have already achieved pre-elimination status, but an important role is also possible in areas with intense transmission. Since vaccination would prevent, but not treat malaria infection, drug clearance in vaccinees would be required in order to halt ongoing transmission. This meshes with the need discussed earlier for pre-treatment before immunization in individuals residing in endemic areas. The recognition that mass drug administration (MDA) would accompany MVPs supporting malaria elimination raises the option that the administration of long-acting antimalarials as part of MDA could provide the drug partner for a chemo-attenuated PfSPZ approach. In accordance with this plan, a trial of PfSPZ-CVac using pyronaridine as the partner drug is planned for 2023 or 2024 at the ITM in Tübingen, with the potential for a follow-on field trial in Burkina Faso. Pyronaridine offers the possibility of parenteral injection, which would assure compliance and improve safety, and is also an ideal drug when combined with artemisinin derivatives for MDA. However, due to the safety concerns associated with PfSPZ-CVac, it is likely that a fully attenuated parasite will be a better option. On the assumption that PfSPZ-LARC2 Vaccine is shown to be fully attenuated, an example of an MVP using this vaccine is provided in .
Figure 9. A hypothetical mass vaccination program (MVP) using PfSPZ-LARC2 Vaccine. As envisioned by the authors, a malaria elimination campaign would be designed for a target geographical region including appropriate community engagement. Mass drug administration (MDA) such as artesunate + pyronaridine [Citation290] would be conducted prior to immunization to reduce the suppression of vaccine responses by existing parasitemia and to eliminate the human parasite reservoir. Intensified vector control would help to eliminate the mosquito reservoir. Immunization with PfSPZ-LARC2 Vaccine would then be performed to protect the population against the acquisition of new infections. An additional round of MDA might be needed post immunization to achieve elimination. Primaquine would be used to eliminate infectious gametocytes. AS = artesunate; PYRON = pyronaridine; PQ = primaquine.
![Figure 9. A hypothetical mass vaccination program (MVP) using PfSPZ-LARC2 Vaccine. As envisioned by the authors, a malaria elimination campaign would be designed for a target geographical region including appropriate community engagement. Mass drug administration (MDA) such as artesunate + pyronaridine [Citation290] would be conducted prior to immunization to reduce the suppression of vaccine responses by existing parasitemia and to eliminate the human parasite reservoir. Intensified vector control would help to eliminate the mosquito reservoir. Immunization with PfSPZ-LARC2 Vaccine would then be performed to protect the population against the acquisition of new infections. An additional round of MDA might be needed post immunization to achieve elimination. Primaquine would be used to eliminate infectious gametocytes. AS = artesunate; PYRON = pyronaridine; PQ = primaquine.](/cms/asset/0d5eca6a-0fcb-4ea1-bfa3-6edf1d4b76c3/ierv_a_2245890_f0009_oc.jpg)
An important aspect of MVPs will be to combine interventions to achieve synergistic effects. Vector control, protection from mosquito bites, efficient case identification and treatment, and control of imported infections will all be critical. Vaccines targeting the sexual stages of the parasite, preventing transmission to the mosquito, are a promising tool [Citation291] that could readily be combined with PfSPZ vaccines. As transmission should be reduced progressively by using two VIMTs in combination, the parasite burden in individuals will be reduced, helping to alleviate the need for drug treatment. Another powerful intervention on the horizon is monoclonal antibodies which, although possibly limited by short duration of efficacy, are highly efficacious in field studies [Citation157] and may greatly support the success of MVPs.
8. Booster doses
Sanaria anticipates that periodic booster doses will be required for several of these applications, including women living in endemic areas who are newly pregnant and have previously received a full primary series, previously vaccinated travelers contemplating a new trip to a malaria-endemic area, children undergoing seasonal vaccination in the Sahel, and residents of defined geographic regions where a malaria elimination campaign is underway.
Field studies in the Sahel are assessing the need for a booster dose. In the trial in Burkina Faso, participants were followed during a second transmission season without repeat drug clearance and without a booster dose; although there were no significant differences in malaria incidence between vaccinees and controls during the second transmission season, the inverse parasite-free survival curves of the two groups remained separated and VE over the full 76-week follow-up period remained statistically significant, indicating that the benefits of vaccination were durable () [Citation7]. Trials in Mali are assessing protection in the second year after vaccination, either with or without booster doses, both for PfSPZ Vaccine [Citation112] (Diawara unpublished [NCT03989102]) and for PfSPZ-CVac (PYR) (Sagara, unpublished [NCT03952650]). Further studies therefore are needed to clarify the need for and timing of booster doses in each epidemiological context.
Figure 10. Efficacy of PfSPZ Vaccine against naturally transmitted Pf infection in Burkina Faso adults. Inverse survival curves for time after the last vaccination to first positive thick blood smear (asymptomatic + symptomatic infection) are shown. Efficacy was analyzed as 1-hazard ratio for the primary follow-up period (0–24 weeks post third vaccination) and the extended follow-up period (0–76 weeks post third vaccination). The survival curves include 79 participants who received all three vaccinations and were evaluable for the vaccine efficacy endpoint. Reproduced with permission from Science Translational Medicine (no significant changes were made) [Citation7].
![Figure 10. Efficacy of PfSPZ Vaccine against naturally transmitted Pf infection in Burkina Faso adults. Inverse survival curves for time after the last vaccination to first positive thick blood smear (asymptomatic + symptomatic infection) are shown. Efficacy was analyzed as 1-hazard ratio for the primary follow-up period (0–24 weeks post third vaccination) and the extended follow-up period (0–76 weeks post third vaccination). The survival curves include 79 participants who received all three vaccinations and were evaluable for the vaccine efficacy endpoint. Reproduced with permission from Science Translational Medicine (no significant changes were made) [Citation7].](/cms/asset/64297e6a-d6fa-4063-88e0-cab94d9c1a42/ierv_a_2245890_f0010_oc.jpg)
9. Plans for licensure
Among the different PfSPZ vaccine platforms, only PfSPZ Vaccine currently has a dossier justifying advancement to pivotal Phase 3 clinical trials in support of marketing authorization. This is based on the results of Phase 2 trials in Africa (including the not-yet-published MLSPZV4 trial in women of childbearing potential) that demonstrate significant protection against Pf infection and clinical malaria in African adults. Although PfSPZ Vaccine has not achieved the >90% VE against heterologous CHMI at 12 weeks that Sanaria has established as optimal, it could nevertheless benefit target groups such as women who want to become pregnant and travelers, and its licensure could add a powerful weapon to the arsenal of antimalarial interventions.
Developers of LARC parasites, such as PfSPZ-LARC2 Vaccine expect this new technology to achieve higher efficacy than PfSPZ Vaccine, and do so at significantly lower doses, simultaneously increasing the potential for public benefit and reducing cost of goods (COGs). The results of the trials planned for 2023–2024 will determine whether PfSPZ-LARC2 Vaccine supplants PfSPZ Vaccine as the lead candidate for licensure.
The initial indication for licensure, anticipated in the EU, is planned to be, ‘prophylaxis of Pf malaria in adult travelers to Africa’ and will be expanded within 1–2 years through an FDA biologics license application (BLA) for the target indication ‘prophylaxis of Pf malaria in residents of malaria-endemic areas,’ initially women of childbearing potential (WOCBP) to prevent malaria in pregnancy, and then other populations.
10. Conclusions
The developers of whole PfSPZ vaccines have achieved major advances, including an innovative manufacturing process, effective cryopreservation suitable for a eukaryotic whole-cell vaccine, and novel routes and regimens for immunization. Multiple platforms have been developed, including radiation-attenuated, chemo-attenuated, and genetically attenuated PfSPZ. In addition, the rodent malaria parasite P. berghei has been genetically engineered to express the major PfSPZ surface protein, PfCSP.
The first generation, irradiated PfSPZ vaccine (PfSPZ Vaccine) has proved well tolerated and safe and has provided sustained efficacy against heterologous CHMI in malaria-naive individuals at 8 months and against naturally transmitted malaria in Mali and Burkina Faso for 18–19 months without boosting (assessment of vaccine efficacy over longer periods is now underway). In these field trials showing protection, all subjects were treated presumptively with an antimalarial drug before the first and last doses to clear existing parasitemias. In trials in which treatment was not administered before the first dose, there was no significant VE at the primary endpoint.
Even among malaria-experienced adults who received presumptive treatment, immune responses to PfSPZ vaccination are substantially lower than in malaria-naïve vaccinees in the U.S.A. and Europe who receive the same regimens. Overcoming vaccine hyporesponsiveness will be critical to increasing VE in malaria-experienced adults from the 50–60% to the 90% target. Thus, while PfSPZ Vaccine could be advanced to pivotal Phase 3 clinical trials to support marketing authorization, it has not achieved the >90% VE against heterologous CHMI at 12 weeks after last vaccine dose that Sanaria believes is needed to meet all development objectives.
The second generation, chemo-attenuated PfSPZ vaccine, PfSPZ-CVac (CQ) has achieved 100% VE against heterologous CHMI with a divergent South American parasite 12 weeks after immunization in malaria-naive adults [Citation68] at a dose 22% of the radiation-attenuated PfSPZ Vaccine that achieved 78% VE against heterologous CHMI at 9–10 weeks [Citation6]. 100% VE for 3 months against heterologous CHMI is unequaled in the malaria vaccine field and establishes that the target VE is achievable. However, PfSPZ-CVac (CQ) can cause significant adverse events on days 7 and 8 after administration of the first dose unless an NSAID such as ibuprofen is taken and could cause severe malaria if the CQ is not absorbed or taken properly. Therefore, it is not ideal from a tolerability and safety perspective.
The third generation, genetically attenuated LARC vaccine, PfSPZ-LARC2 Vaccine, should provide the tolerability and safety of PfSPZ Vaccine and the VE of PfSPZ-CVac (CQ) at ~20% the dose of PfSPZ Vaccine, and at the same time eliminate the potential risks and side effects of PfSPZ-CVac (CQ). Clinical trials are set to begin in 2023–2024. The foundation provided by PfSPZ Vaccine and PfSPZ-CVac should facilitate rapid transition of PfSPZ-LARC2 Vaccine from Phase 1 to 3 clinical trials and licensure if the anticipated milestones of safety and VE are met.
11. Expert opinion
Countries on the geographic margins of malaria transmission continue to make progress, with several, such as China and El Salvador recently obtaining WHO certification that they are malaria free. Since 2017, the WHO E-2020 Initiative has supported 21 countries to achieve zero indigenous cases, with eight of these countries reporting zero cases by 2020 [Citation292]. In contrast, progress against malaria has stalled in those countries most burdened by this disease, such as in sub-Saharan Africa [Citation283], indicating that existing control measures are insufficient, at least in the context of current funding. A new tool, such as a vaccine with high-level efficacy against infection, is needed in the fight against the malaria parasite. WHO has recently emphasized this need [Citation289]. PfSPZ vaccines are being developed to meet WHO’s call for >90% VE against Pf infection.
The malaria vaccines poised for deployment are RTS,S/AS01 and biosimilar R21, partially effective subunit vaccines based on the PfCSP. RTS,S/AS01 has completed pilot implementation in three African countries and has been recommended by WHO for further roll-out [Citation293], and R21 [Citation107,Citation294] has recently been licensed in two African countries [Citation103,Citation104]. These vaccines are designed to reduce childhood morbidity. Potency against infection (as opposed to disease) was not a primary outcome for the Phase 3 trials of RTS,S or R21, and data on sterile protection beyond the conduct of periodic (e.g. every 6 months) cross-sectional prevalence assessments generally have not been reported from field studies [Citation107,Citation295], although it can be presumed that partial protection against infection is provided.
Regional malaria elimination will require mass vaccination programs (MVPs) reaching enough of the population to achieve herd immunity. PfSPZ Vaccine meets the tolerability and safety requirement for MVPs but, with VEs in the Sahel ranging from 50% to 60% over 18–19 months, has not achieved the >90% VE target. PfSPZ-CVac (CQ) has met the VE goal against CHMI but may not meet the tolerability and safety requirements. For this reason, the focus is on PfSPZ-LARC2 Vaccine, expected to induce equivalent VE as PfSPZ-CVac (CQ) without the tolerability problems and safety risk. The ability to block transmission in MVPs could be enhanced by combining PfSPZ with a VIMT targeting sexual stages [Citation296].
In addition to high efficacy, safety and tolerability, MVPs must be feasible. The use of DVI for administration and of LNVP for storage and distribution have raised concerns. However, DVI has been well received by field teams and has been used successfully in adults, children, and infants [Citation2,Citation3,Citation7,Citation65,Citation124,Citation126,Citation129,Citation136–138,Citation164]. LNVP stabilizes the vaccine in the absence of electricity and is widely used in Africa to distribute veterinary vaccines [Citation116]. Thus, the establishment of a new human vaccine distribution network based on LNVP could build on existing capabilities. Sanaria and collaborators intend to explore the feasibility of MVPs through the conduct of cluster-randomized trials in which villages receive a program of MDA and vaccination, to see whether MVPs can be conducted using DVI and LNVP distribution, and whether vaccination combined with existing malaria control interventions significantly reduces transmission.
In our opinion, the biggest challenge is not DVI, cryopreservation or the execution of MVPs. Rather, it is achieving the required level of VE – ≥90% sterile protective efficacy – in the endemic setting where ongoing parasitemia is inhibiting vaccine potency and life-long exposure diminishes the capacity to respond to malaria antigens. Drug clearance before vaccination will allow partial immunological recovery, but studies have not yet demonstrated that the resulting protection is high enough, or that the immunological disadvantage of prior and ongoing exposure can be fully circumvented, with achieving this in children a major concern.
Five years from now, we envision that PfSPZ-LARC2 Vaccine will be licensed for use in travelers and women of childbearing potential. If this vaccine meets key milestones – exhibiting the safety of PfSPZ Vaccine, VE approaching that of PfSPZ-CVac, and reduced COGs based on lower dosing – it will supplant PfSPZ Vaccine as the lead product. On the other hand, if PfSPZ-LARC2 does not meet milestones, PfSPZ Vaccine will be licensed for use in the same populations, with ongoing efforts under way to improve the VE of PfSPZ vaccines beyond what has currently been achieved. A dramatic reduction in COGs is also anticipated, based on more efficient and more scalable approaches to manufacturing, such as in vitro production of PfSPZ in bioreactors [Citation9] and the introduction of a dose-sparing adjuvant [Citation251].
The success to date of PfSPZ vaccine development has depended on overcoming challenges through innovative approaches and technologies. The expectation is that continued efforts following the same principles of open science, collaboration, and innovation will bring this enterprise to a successful outcome.
Article highlights
Since the 1970s, radiation-attenuated Plasmodium falciparum (Pf) sporozoites (SPZ) inoculated into humans by mosquito bite have been known to induce high-level immunity to Pf malaria infection, but this was never considered a practical approach to vaccination.
Sanaria Inc. has developed regulatory-compliant methods for the production of aseptic, purified, vialed, cryopreserved PfSPZ using mosquitoes as the ‘bioreactor,’ which can be injected with a needle and syringe to protect travelers and residents of malaria-endemic areas.
First generation, radiation-attenuated PfSPZ, called Sanaria® PfSPZ Vaccine, are safe, well tolerated, and protect 80–100% of recipients against homologous (same strain) controlled human malaria infection (CHMI) at 3 weeks and up to 60% of recipients against naturally transmitted Pf malaria for at least 18–19 months without boosting.
Second generation, chemo-attenuated PfSPZ, called Sanaria® PfSPZ-CVac, replicate in the liver, amplifying the immunogen and increasing potency, and achieve 100% protection against heterologous (variant strain) CHMI at 12 weeks at ~20% the dose of PfSPZ Vaccine, but pose a safety risk due to the requirement for co-administered drug.
Third generation, genetically attenuated PfSPZ, such as Sanaria®PfSPZ-LARC2 Vaccine, replicate in the liver like chemo-attenuated PfSPZ but spontaneously arrest development without the need for a partner drug, and will be tested in humans in 2023-2024. PfSPZ-LARC2 Vaccine is expected to have the safety of PfSPZ Vaccine and the efficacy of PfSPZ-CVac.
Sanaria is developing in vitro production of PfSPZ to replace current production in aseptic mosquitoes. Once standardized for GMP manufacture, in vitro production will provide ample PfSPZ to meet the world’s needs as well as a 90% reduction in the cost of goods.
Biological challenges faced by developers include the reduced immunogenicity of PfSPZ vaccines in malaria-exposed persons living in endemic areas and the antigenic diversity of the Pf malaria parasite.
PfSPZ vaccines are optimally administered by direct venous inoculation in 0.3 to 0.5 mL, a quick (seconds) and nearly painless procedure representing a new skill to be acquired by clinical teams.
PfSPZ vaccines require a liquid nitrogen vapor phase (LNVP) cold chain for cryostabilization, which allows delivery to remote locations without the need for electricity, ideal for mass vaccination programs designed to halt transmission and eliminate malaria from defined geographic areas.
We estimate that, with adequate funding, a PfSPZ vaccine will be licensed for use within the next 5 years.
Declaration of interests
Thomas L. Richie, L.W. Preston Church, Tooba Murshedkar, Peter F. Billingsley, Eric R. James, Mei-Chun Chen, Yonas Abebe, Natasha KC, Sumana Chakravarty, David Dolberg, B. Kim Lee Sim, and Stephen L. Hoffman are salaried, full-time employees of Sanaria Inc. B. Kim Lee Sim and Stephen L. Hoffman own stock in Sanaria. Benjamin Mordmüller has a consultancy agreement on vaccine development with Nobelpharma Co., Ltd., Japan. Robert W. Sauerwein has stock ownership in TropIQ Health Sciences, Nijmegen, The Netherlands. The authors have no other relevant affiliations or financial involvement with any organization or entity with a financial interest in or financial conflict with the subject matter or materials discussed in the manuscript apart from those disclosed.
Reviewer disclosures
Peer reviewers on this manuscript have no relevant financial or other relationships to disclose.
Disclaimer
The findings and conclusions in this report are those of the authors and do not necessarily represent the official position of the Centers for Disease Control and Prevention, or the National Institutes of Health.
Author contributions
All authors have contributed to the conception and design of the review article and interpreting the relevant literature and have been involved in writing the review article or revised it for intellectual content.
Dedication
This review is dedicated to Shahid Kahn, our dear friend, inspirational colleague and brilliant pioneer in the field of genetically altered parasites, and to Ogobara Doumbo, extraordinary leader, beloved mentor, clinical trial champion, shepherd of international good will, and lion in the fight against malaria. Both are sorely missed.
Acknowledgments
We warmly thank the many institutions, investigators, and their teams who were or are involved in the conduct of completed, ongoing, and planned trials of PfSPZ products, for their collaboration and, in many cases, financial support (listed by institution in alphabetical order):
Biostatistics Research Branch, National Institute of Allergy and Infectious Diseases, NIH, Rockville, MD, United States: Zonghui Hu, Martha Nason, Jing Wang, Erin E. Gabriel; Centers for Disease Control, Atlanta, GA, United States: Julie Gutman, Ryan Wiegand, Aaron Samuels, Patrick Kachur; Centre National de Recherche et de Formation sur le Paludisme (CNRFP) and Groupe de Recherche Action en Santé (GRAS), Ouagadougou, Burkina Faso: Alphonse Ouedraogo, Alfred Tiono, Edith Bougouma, Issa Diarra; CERMEL (Centre de Recherches Médicales de Lambaréné), Lambaréné, Gabon: Anita Lumeka Kabwende, Jean-Claude Dejon Agobe, Jeannot Zinzou, Bertrand Lell, Pamela Minsoko, Armel Mbouna, Ayodele Alabi; Equatorial Guinea Ministry of Health and Social Welfare, Malabo, Equatorial Guinea: Mitoha Ondo’o Ayekaba, Diosdado Nsue Milang, Vicente Urbano Nsue Ndong Nchama, Juan Carlos Momo Besaha, Antonio Enrique Ngua Sama Roca, Genaro Nsue Nguema, Beltran Ekua Pasialo, Martín Eka Ondó Mangue, Gertrudis Owono Bidjimi, Marta Alene Owono Eyang, Escolastica Raquel Mansogo Maye, José Raso, Fortunata Lobede, Dolores Mbang, Esther Eburi; Emmes Corporation: David Styers, Claire Stablein, Gail Potter; Ifakara Health Institute, Bagamoyo, United Republic of Tanzania: Ali Hamad, Ali Mtoro, Kamaka Ramadhani, Maximillian Mpina, Elizabeth Nyakarungu, Mwajuma Chemba, Thabit Athuman, Ummi Abdul; Institute for Genome Sciences, University of Maryland School of Medicine, Baltimore: Ankit Dwivedi, Kara Moser, Myron Levine; Institute of Tropical Medicine, University of Tübingen, Tübingen, Germany: Mihály Sulyok, Rolf Fendel, Zita Sulyok, Steffen Borrmann, Albert Lalremruata, Annette Knoblich, Markus Gmeiner, Jaana Heinze, Diane Egger-Adam; Kaiser Permanente Washington Health Research Institute (formerly Group Health), Seattle, WA, United States: Lisa Jackson; Kenya Medical Research Institute (KEMRI), Wellcome Trust, and Centre for Research in Therapeutic Sciences (CREATES), Nairobi, Kenya: Simon Kariuki, Philip Bejon, Kephas Otieno, Tony Sang, Reuben Yego Cherop; Laboratory of Malaria Immunology and Vaccinology, National Institute of Allergy and Infectious Diseases, NIH, Bethesda, MD, United States: Agnes Mwakingwe-Omari, Irfan Zaidi, J. Patrick Gorres, Aye Diallo, Blair J. Wylie, Alemush Imeru, Emily Higbee, Kelly Rausch, Rathy Mohan, Viyada Doan, Kelly Ding, Regina White, Sharon Wong-Madden, Maria Conner, Jacquelyn Lane, Freda Omaswa; Leiden University Medical Center, Leiden, the Netherlands: Shahid Kahn; Liverpool School of Tropical Medicine: Feiko Ter Kuile; Malaria Research Group, Center for Vaccine Development and Global Health, University of Maryland School of Medicine, Baltimore, MD, United States: Andrea Berry, Colleen Boyce, Alison Kwon; Malaria Research and Training Center, University of Bamako, Bamako, Mali: Ogobara Doumbo, Mahamadou Thera, Drissa Coulibaly, Amagana Dolo, Mahamadoun H. Assadou, Abdoulaye Katile, Bourama Kamate, Djibrilla Issiaka, Kourane Sissoko, Amatigue Zeguime, Merepen A. Guindo, M’Bouye Doucoure, Kalifa Diarra, Oumar Attaher, Amadou Niangaly, Balla Diarra, Karamoko Niare, Ismaili Thera; Marathon Oil: Carl Maas, Bonifacio Manguire Nlavo; Medical Care Development International, Malabo, Equatorial Guinea: Guillermo Garcia, Chris Schwabe, Carlos Cortes Falla, Wonder Phiri, Jeremias Nzamio; Jordan Smith; Naval Medical Research Center, Silver Spring, MD, United States: Alexandra Singer, Martha Sedegah, Eileen Villasante; Oxford University Clinical Research Unit Indonesia (OUCRU Indonesia), University of Indonesia Faculty of Medicine, Eijkman Institute of Molecular Biology, Indonesian Army, Jakarta, Indonesia: Erni Nelwan, J. Kevin Baird, Krisin Chand, Amin Soebandrio, Rintis Noviyanti, Nur Abdul Ghoni, Yogi Ertanto, Decy Subekti, Rizka Fahmia, Mutia Rahardjani, Fitri Wulandari; Michael Christian, Samuel Manalu, Khoriah Indrihutami, Margareta Oktaviani, Rara Aulia, Marillyn Tamburian, Agus Rachmat, Yulia Widya; Radboud University Medical Center, Nijmegen, the Netherlands: Guido Bastiaens, Else Bijker, Cornelus Hermsen, Ben van Schaijk; Sanaria Inc./Protein Potential, Rockville, MD, United States: The manufacturing, quality, regulatory, clinical, logistics, immunology, pharmaceutical operations, administrative, legal, and project management teams including, Abraham Eappen, Tao Li, Minglin Li, Jeremy Guth, Anita Manoj, Anusha Gunasekera, Asha Patil, LiXin Gao, Rui Xu, Faith Beams, Maria Orozco, Bing Jiang, Shachi Shah, Thomas Stabler, Raul Chuquiyauri, Steve Manock, Pouria Riyahi, Elizabeth Saverino, Ravi Punjabi, Diana Perez, Sue Rupprecht, Alexander Hoffman, Chris Carmona, Laurence Lemiale; Sanaria contractors: Almudena Llegarda, Ninky Acevedo; Seattle Children’s Research Institute, Seattle, WA, USA: Deba Goswami, Nelly Camargo, Will Betz, Janna Gibson, Kenza Oualim, Silvia Arredondo; Seattle Malaria Clinical Trials, Fred Hutchinson Cancer Center: Elizabeth Duke, Dianna Hergott, Gift Nwanne; Swiss Tropical and Public Health Institute, Basel, Switzerland: Tobias Schindler, Tobias Rutishauser, Anna Deal; University of Massachusetts: Anne Moormann; University of Washington, Seattle, WA, United States: Annette M. Seilie, Ming Chang; Vaccine Research Center, National Institute of Allergy and Infectious Disease, NIH, Bethesda, MD, United States: Robert Seder, Julie Ledgerwood.
We gratefully acknowledge the past and current support of the following funding institutions: the Division of Microbiology and Infectious Diseases, NIAID, NIH, which has supported discovery research, preclinical development including manufacturing and quality control release, clinical trials and nonclinical activities; the Laboratory of Malaria Immunology and Vaccinology, NIAID, NIH; the Vaccine Research Center, NIAID, NIH; the Government of Equatorial Guinea and Marathon Oil Corporation (in partnership with EG LNG, AMPCO, and Noble Energy); the US Navy Advanced Medical Development Program; the Military Infectious Disease Research Program; the Congressionally Directed Medical Research Program; the US Army Medical Materiel Development Activity; PATH Malaria Vaccine Initiative (Bill & Melinda Gates Foundation [BMGF]); Institute for OneWorld Health (BMGF); the BMGF; TI Pharma; the European Union Malaria Fund; the German Centre for Infection Research (DZIF); the Swiss State Secretariat for Education, Research and Innovation; the Tanzanian Commission on Science and Technology (COSTECH); the European & Developing Countries Clinical Trials Partnership (EDCTP); the Wellcome Trust; the Broad Institute; and the Medicines for Malaria Venture.
We express our appreciation to the 2046 research volunteers who have participated to date in trials of Sanaria PfSPZ vaccines.
Additional information
Funding
Notes
1. Heterologous = challenge strain genetically and antigenically distinct from the vaccine strain, making heterologous CHMI a more stringent assessment of VE than homologous CHMI.
2. Homologous = challenge strain the same as the vaccine strain.
3. The terms sterile immunity and sterile protection as used in this review mean that no parasitemia is detected during CHMI follow-up, indicating complete blockade of parasite development prior to the blood stage. The modifier ‘sterile’ is used because partially effective vaccines have been credited with inducing immunity when liver burdens have been reduced or the prepatent period to blood stage infection has been lengthened, even though trial participants undergoing CHMI have developed parasitemia.
4. Adenovirus, Mycobacterium tuberculosis, Vibrio cholera, dengue virus, Ebola vírus, influenza virus, measles virus, mumps virus, rubella virus, rotavirus, variola and monkeypox viruses, Salmonella Typhi, varicella-zoster virus, yellow fever virus and poliovirus (previously licensed; now replaced with inactivated polio virus vaccine).
5. Bacillus anthracis, hepatitis A virus, Japanese encephalitis virus, Yersinia pestis, tick-borne encephalitis virus, rabies virus.
6. 5/5 was the result of the first of three CHMI’s. After all three CHMI’s were conducted in the two phases of this trial, at total of 15/17 participants receiving three doses remained parasite free in the blood after homologous CHMI.
7. NF54, the vaccine strain, was isolated in 1979 from a Dutch farmer living near the Amsterdam Schiphol airport, presumably transmitted by an infected mosquito riding on an airplane from a malaria endemic area, since the farmer had never left the Netherlands and had not received a blood transfusion or any other procedure that might have transmitted malaria; genomic analysis strongly suggests that the strain is West African in origin.
8. Clearance was performed after the third (last) immunization so that new infections could be identified. It was not understood at that time that it should have been performed before the third immunization, fulfilling two purposes at once: allowing new infections to be identified during surveillance and reducing immune suppression during the third immunization.
9. The ability to produce abundant stage V gametocytes in culture and high sporozoite yields in the salivary glands of mosquitoes fed on those cultures are key attributes required for the successful manufacture of PfSPZ Challenge. Pf7G8 has been selected at this point as a primary strain for heterologous CHMI because it is a reasonably good producer of gametocytes/oocysts/sporozoites (although not as good as PfNF54), but Sanaria is now developing divergent strains of PfSPZ Challenge from East Africa (PfNF54 is from West Africa) and from Southeast Asia to expand the stringency and geographic representation of heterologous CHMI.
References
- Seder RA, Chang LJ, Enama ME, et al. Protection against malaria by intravenous immunization with a nonreplicating sporozoite vaccine. Science. 2013 Sep 20;341(6152):p. 1359–1365.
- Jongo SA, Church LWP, Mtoro AT, et al. Increase of dose associated with decrease in protection against controlled human malaria infection by PfSPZ Vaccine in Tanzanian adults. Clin Infect Dis. 2020 Nov 29;71(11):p. 2849–2857.
- Sissoko MS, Healy SA, Katile A, et al. Safety and efficacy of a three-dose regimen of Plasmodium falciparum sporozoite vaccine in adults during an intense malaria transmission season in Mali: a randomised, controlled phase 1 trial. Lancet Infect Dis. 2022 Nov 18;22(3):p. 377–389.
- Ishizuka AS, Lyke KE, DeZure A, et al. Protection against malaria at 1 year and immune correlates following PfSPZ vaccination. Nat Med. 2016 May 9;22(6):p. 614–623.
- Lyke KE, Ishizuka AS, Berry AA, et al. Attenuated PfSPZ vaccine induces strain-transcending T cells and durable protection against heterologous controlled human malaria infection. Proc Natl Acad Sci U S A. 2017 Mar 07;114(10):p. 2711–2716.
- Mordmüller B, Sulyok Z, Sulyok M, et al. A PfSPZ Vaccine immunization regimen equally protective against homologous and heterologous controlled human malaria infection. NPJ Vaccines. 2022 Aug 23;7(1):100. doi: 10.1038/s41541-022-00510-z
- Sirima SB, Ouédraogo A, Tiono AB, et al. A randomized controlled trial showing safety and durable efficacy of a whole sporozoite vaccine against endemic malaria. Sci Transl Med. 2022;14(674):p. eabj3776.
- Mordmüller B, Surat G, Lagler H, et al. Sterile protection against human malaria by chemoattenuated PfSPZ vaccine. Nature. 2017 Feb 23;542(7642):p. 445–449.
- Eappen AG, Li T, Marquette M, et al. In vitro production of infectious Plasmodium falciparum sporozoites. Nature. 2022;612(7940):p. 534–539.
- Sergent E, Sergent E. Sur l’immunité dans le paludisme des oiseaux. Conservation in vitro des sporozoites de Plasmodium relictum. Immunité relative obtenue par inoculation de ces sporozoites. CR Acad Sci. 1910;151:407–409.
- Mulligan HW, Russell P, Mohan BN. Active immunization of fowls against Plasmodium gallinaceum by injections of killed homologous sporozoites. J Malaria Inst India. 1941;4:25–34.
- Russell PF, Mohan BN. The immunization of fowls against mosquito-borne Plasmodium gallinaceum by injections of serum and of inactivated homologous sporozoites. J Exp Med. 1942 Nov 1;76(5):477–495.
- Freund J, Thomson KJ, Sommer HE, et al. Immunization of rhesus monkeys against malaria infection (P. knowlesi) with killed parasites and adjuvants. Science. 1945 Aug 24;102(2643):202–204.
- Freund J, Sommer HE, Walter AW. Immunization against malaria: vaccination of ducks with killed parasites incorporated with adjuvants. Science. 1945 Aug 24;102(2643):200–202.
- Richards WGH. Active immunization of chicks against Plasmodium gallinacium by inactivated homologous sporozoites and erythrocytic parasites. Nature. 1966;212(5069):1492–1494. doi: 10.1038/2121492a0
- Nussenzweig RS, Vanderberg J, Most H, et al. Protective immunity produced by the injection of X-irradiated sporozoites of Plasmodium berghei. Nature. 1967;216(5111):160–162. doi: 10.1038/216160a0
- Clyde DF, McCarthy VC, Miller RM, et al. Specificity of protection of man immunized against sporozoite-induced falciparum malaria. Am J Med Sci. 1973;266(6):398–403. doi: 10.1097/00000441-197312000-00001
- Clyde DF, Most H, McCarthy VC, et al. Immunization of man against sporozite-induced falciparum malaria. Am J Med Sci. 1973;266(3):169–177. doi: 10.1097/00000441-197309000-00002
- Clyde DF, McCarthy VC, Miller RM, et al. Immunization of man against falciparum and vivax malaria by use of attenuated sporozoites. Am J Trop Med Hyg. 1975;24(3):397–401. doi: 10.4269/ajtmh.1975.24.397
- McCarthy VC, Clyde DF. Plasmodium vivax: Correlation of circumsporozoite precipitation (CSP) reaction with sporozoite-induced protective immunity in man. Exp Parasitol. 1977;41(1):167–171. doi: 10.1016/0014-4894(77)90142-4
- Clyde DF. Immunity to falciparum and vivax malaria induced by irradiated sporozoites: a review of the University of Maryland studies, 1971-75. Bull World Health Organ. 1990;68(Suppl):9–12.
- Rieckmann KH, Carson PE, Beaudoin RL, et al. Sporozoite induced immunity in man against an Ethiopian strain of Plasmodium falciparum. Trans R Soc Trop Med Hyg. 1974;68(3):258–259. doi: 10.1016/0035-9203(74)90129-1
- Rieckmann KH, Beaudoin RL, Cassells JS, et al. Use of attenuated sporozoites in the immunization of human volunteers against falciparum malaria. Bull World Health Organ. 1979;57 Suppl 1(Suppl):261–265.
- Rieckmann KH. Human immunization with attenuated sporozoites. Bull World Health Organ. 1990;68 Suppl(Suppl):13–16.
- Hoffman SL, Goh LM, Luke TC, et al. Protection of humans against malaria by immunization with radiation-attenuated Plasmodium falciparum sporozoites. J Infect Dis. 2002 Apr 15;185(8):1155–1164. doi: 10.1086/339409
- Collins WE, Contacos PG. Immunization of monkeys against Plasmodium cynomolgi by X-irradiated sporozoites. Nat New Biol. 1972 Apr 12;236(67):176–177.
- Gwadz RW, Cochrane AH, Nussenzweig V, et al. Preliminary studies on vaccination of rhesus monkeys with irradiated sporozoites of Plasmodium knowlesi and characterization of surface antigens of these parasites. Bull World Health Organ. 1979;57 Suppl 1(Suppl):165–173.
- Jordan-Villegas A, Perdomo AB, Epstein JE, et al. Immune responses and protection of Aotus monkeys immunized with irradiated Plasmodium vivax sporozoites. Am J Trop Med Hyg. 2011 Feb;84(2 Suppl):43–50. doi: 10.4269/ajtmh.2011.09-0759
- Hoffman SL, Isenbarger D, Long GW, et al. Sporozoite vaccine induces genetically restricted T cell elimination of malaria from hepatocytes. Science. 1989 Jun 2;244(4908):1078–1081. doi: 10.1126/science.2524877
- Weiss WR, Mellouk S, Houghten RA, et al. Cytotoxic T cells recognize a peptide from the circumsporozoite protein on malaria-infected hepatocytes. J Exp Med. 1990 Mar 1;171(3):763–773. doi: 10.1084/jem.171.3.763
- Romero P, Maryanski JL, Corradin G, et al. Cloned cytotoxic T cells recognize an epitope in the circumsporozoite protein and protect against malaria. Nature. 1989;341(6240):323–325. doi: 10.1038/341323a0
- Rodrigues MM, Cordey A-S, Arreaza G, et al. CD8+ cytolytic T cell clones derived against the Plasmodium yoelii circumsporozoite protein protect against malaria. Int Immunol. 1991;3(6):579–585. doi: 10.1093/intimm/3.6.579
- Khusmith S, Sedegah M, Hoffman SL. Complete protection against Plasmodium yoelii by adoptive transfer of a CD8+ cytotoxic T-cell clone recognizing sporozoite surface protein 2. Infect Immun. 1994 Jul;62(7):2979–2983. doi: 10.1128/iai.62.7.2979-2983.1994
- Guebre-Xabier M, Schwenk R, Krzych U. Memory phenotype CD8(+) T cells persist in livers of mice protected against malaria by immunization with attenuated Plasmodium berghei sporozoites. Eur J Immunol. 1999 Dec;29(12):3978–3986. doi: 10.1002/(SICI)1521-4141(199912)29:12<3978:AID-IMMU3978>3.0.CO;2-0
- Weiss WR, Sedegah M, Beaudoin RL, et al. CD8+ T cells (cytotoxic/suppressors) are required for protection in mice immunized with malaria sporozoites. Proc Natl Acad Sci U S A. 1988;85(2):573–576. doi: 10.1073/pnas.85.2.573
- Weiss WR, Berzofsky JA, Houghten RA, et al. A T cell clone directed at the circumsporozoite protein which protects mice against both Plasmodium yoelii and Plasmodium berghei. J Immunol. 1992 Sep 15;149(6):2103–2109.
- Schofield L, Villaquiran J, Ferreira A, et al. γ interferon, CD8+ T cells and antibodies required for immunity to malaria sporozoites. Nature. 1987;330(6149):664–666. doi: 10.1038/330664a0
- Doolan DL, Hoffman SL. IL-12 and NK cells are required for antigen-specific adaptive immunity against malaria initiated by CD8+ T cells in the Plasmodium yoelii model. J Immunol. 1999 Jul 15;163(2):884–892.
- Doolan DL, Hoffman SL. The complexity of protective immunity against liver-stage malaria. J Immunol. 2000 Aug 1;165(3):1453–1462.
- Trimnell A, Takagi A, Gupta M, et al. Genetically attenuated parasite vaccines induce contact-dependent CD8+ T cell killing of Plasmodium yoelii liver stage-infected hepatocytes. J Immunol. 2009 Nov 1;183(9):5870–5878.
- Krzych U, Dalai S, Zarling S, et al. Memory CD8 T cells specific for plasmodia liver-stage antigens maintain protracted protection against malaria. Front Immunol. 2012;3:370. doi: 10.3389/fimmu.2012.00370
- Weiss WR, Jiang CG, Gruner AC. Protective CD8+ T lymphocytes in primates immunized with malaria sporozoites. PLoS One. 2012;7(2):e31247. doi: 10.1371/journal.pone.0031247
- Rodrigues M, Nussenzweig RS, Zavala F. The relative contribution of antibodies, CD4+ and CD8+ T cells to sporozoite-induced protection against malaria. Immunology. 1993;80:1–5.
- Belnoue E, Costa FTM, Frankenberg T, et al. Protective T cell immunity against malaria liver stage after vaccination with live sporozoites under chloroquine treatment. J Immunol. 2004;172(4):2487–2495. doi: 10.4049/jimmunol.172.4.2487
- Overstreet MG, Chen YC, Cockburn IA, et al. CD4+ T cells modulate expansion and survival but not functional properties of effector and memory CD8+ T cells induced by malaria sporozoites. PLoS One. 2011 Jan 4;6(1):e15948.
- Weiss WR, Sedegah M, Berzofsky JA, et al. The role of CD4+ T cells in immunity to malaria sporozoites. J Immunol. 1993 Sep 1;151(5):2690–2698.
- Malik A, Egan JE, Houghten RA, et al. Human cytotoxic T lymphocytes against the Plasmodium falciparum circumsporozoite protein. Proc Natl Acad Sci U S A. 1991 Apr 15;88(8):3300–3304. doi: 10.1073/pnas.88.8.3300
- Wizel B, Houghten RA, Parker KC, et al. Irradiated sporozoite vaccine induces HLA-B8-restricted cytotoxic T lymphocyte responses against two overlapping epitopes of the Plasmodium falciparum sporozoite surface protein 2. J Exp Med. 1995 Nov 1;182(5):1435–1445.
- Doolan DL, Hoffman SL, Southwood S, et al. Degenerate cytotoxic T cell epitopes from P. falciparum restricted by multiple HLA-A and HLA-B supertype alleles. Immunity. 1997 Jul;7(1):97–112. doi: 10.1016/S1074-7613(00)80513-0
- Doolan DL, Southwood S, Freilich DA, et al. Identification of Plasmodium falciparum antigens by antigenic analysis of genomic and proteomic data. Proc Natl Acad Sci U S A. 2003 Aug 19;100(17):9952–9957.
- Epstein JE, Tewari K, Lyke KE, et al. Live attenuated malaria vaccine designed to protect through hepatic CD8+T cell immunity. Science. 2011 Oct 28;334(6055):475–480. doi: 10.1126/science.1211548
- Berenzon D, Schwenk RJ, Letellier L, et al. Protracted protection to Plasmodium berghei malaria is linked to functionally and phenotypically heterogeneous liver memory CD8+ T cells. J Immunol. 2003 Aug 15;171(4):2024–2034.
- Frevert U, Nardin E. Cellular effector mechanisms against Plasmodium liver stages. Cell Microbiol. 2008 Oct;10(10):1956–1967. doi: 10.1111/j.1462-5822.2008.01211.x
- Tse SW, Cockburn IA, Zhang H, et al. Unique transcriptional profile of liver-resident memory CD8+ T cells induced by immunization with malaria sporozoites. Genes Immun. 2013 Jul;14(5):302–309. doi: 10.1038/gene.2013.20
- Olsen TM, Stone BC, Chuenchob V, et al. Prime-and-trap malaria vaccination to generate protective CD8(+) liver-resident memory T cells. J Immunol. 2018 Oct 1;201(7):1984–1993.
- Walk J, Stok JE, Sauerwein RW. Can patrolling liver-resident T cells control human malaria parasite development? Trends Immunol. 2019 Mar;40(3):186–196. doi: 10.1016/j.it.2019.01.002
- Fernandez-Ruiz D, Ng WY, Holz LE, et al. Liver-resident memory CD8+ T cells form a front-line defense against malaria liver-stage infection. Immunity. 2016 Oct 18;45(4):889–902.
- Lefebvre MN, Harty JT. You shall not pass: memory CD8 T cells in liver-stage malaria. Trends Parasitol. 2020 Feb;36(2):147–157. doi: 10.1016/j.pt.2019.11.004
- Tarun AS, Peng X, Dumpit RF, et al. A combined transcriptome and proteome survey of malaria parasite liver stages. Proc Natl Acad Sci U S A. 2008 Jan 8;105(1):305–310.
- Caldelari R, Dogga S, Schmid MW, et al. Transcriptome analysis of Plasmodium berghei during exo-erythrocytic development. Malar J. 2019 Sep 24;18(1):330.
- Murphy SC, Kas A, Stone BC, et al. A T-cell response to a liver-stage Plasmodium antigen is not boosted by repeated sporozoite immunizations. Proc Natl Acad Sci U S A. 2013 Apr 9;110(15):6055–6060.
- Goswami D, Minkah NK, Kappe SHI. Malaria parasite liver stages. J Hepatol. 2022 Mar;76(3):735–737. doi: 10.1016/j.jhep.2021.05.034
- Limbach K, Aguiar J, Gowda K, et al. Identification of two new protective pre-erythrocytic malaria vaccine antigen candidates. Malar J. 2011;10(1):65. doi: 10.1186/1475-2875-10-65
- Aguiar JC, Bolton J, Wanga J, et al. Discovery of novel Plasmodium falciparum pre-erythrocytic antigens for vaccine development. PLoS One. 2015;10(8):e0136109. doi: 10.1371/journal.pone.0136109
- Sissoko MS, Healy SA, Katile A, et al. Safety and efficacy of PfSPZ vaccine against Plasmodium falciparum via direct venous inoculation in healthy malaria-exposed adults in Mali: a randomised, double-blind phase 1 trial. Lancet Infect Dis. 2017 Feb 16;17(5):498–509. doi: 10.1016/S1473-3099(17)30104-4
- Zaidi I, Diallo H, Conteh S, et al. Gammadelta T cells are required for the induction of sterile immunity during irradiated sporozoite vaccinations. J Immunol. 2017 Dec 1;199(11):3781–3788.
- Howard J, Zaidi I, Loizon S, et al. Human Vγ9Vδ2 T lymphocytes in the immune response to P. falciparum infection. Front Immunol. 2018;9:2760. doi: 10.3389/fimmu.2018.02760
- Mwakingwe-Omari A, Healy SA, Lane J, et al. Two chemoattenuated PfSPZ malaria vaccines induce sterile hepatic immunity. Nature. 2021 Jul;595(7866):289–294. doi: 10.1038/s41586-021-03684-z
- Duffy FJ, Hertoghs N, Du Y, et al. Longitudinal immune profiling after radiation-attenuated sporozoite vaccination reveals coordinated immune processes correlated with malaria protection [original Research]. Front Immunol. 2022 [2022 Dec 15];13. doi: 10.3389/fimmu.2022.1042741
- Walk J, Sauerwein RW. Activatory receptor NKp30 predicts NK cell activation during controlled human malaria infection. Front Immunol. 2019;10:2864. doi: 10.3389/fimmu.2019.02864
- Walk J, de Bree LCJ, Graumans W, et al. Outcomes of controlled human malaria infection after BCG vaccination. Nat Commun. 2019 Feb 20;10(1):874.
- Zenklusen I, Jongo S, Abdulla S, et al. Immunization of malaria pre-exposed volunteers with PfSPZ Vaccine elicits long-lived IgM invasion-inhibitory and complement-fixing antibodies. The J Infect Dis. 2018 Feb 8;217(10):1569–1578.
- Suscovich TJ, Fallon JK, Das J, et al. Mapping functional humoral correlates of protection against malaria challenge following RTS,S/AS01 vaccination. Sci Transl Med. 2020;12(553):eabb4757. doi: 10.1126/scitranslmed.abb4757
- Aliprandini E, Tavares J, Panatieri RH, et al. Cytotoxic anti-circumsporozoite antibodies target malaria sporozoites in the host skin. Nat Microbiol. 2018 Nov;3(11):1224–1233. doi: 10.1038/s41564-018-0254-z
- Doolan DL, Dobano C, Baird JK. Acquired immunity to malaria. Clin Microbiol Rev. 2009;22(1):13–36. doi: 10.1128/CMR.00025-08
- Offeddu V, Thathy V, Marsh K, et al. Naturally acquired immune responses against Plasmodium falciparum sporozoites and liver infection. Int J Parasitol. 2012 May 15;42(6):535–548.
- Hoffman SL, Oster CN, Plowe CV, et al. Naturally acquired antibodies to sporozoites do not prevent malaria: vaccine development implications. Science. 1987 Aug 7;237(4815):639–642.
- Owusu-Agyei S, Koram KA, Baird JK, et al. Incidence of symptomatic and asymptomatic Plasmodium falciparum infection following curative therapy in adult residents of northern Ghana. Am J Trop Med Hyg. 2001 Sep;65(3):197–203. doi: 10.4269/ajtmh.2001.65.197
- FDA. Vaccines licensed for use in the United States. 2022. Available at https://www.fda.gov/vaccines-blood-biologics/vaccines/vaccines-licensed-use-united-states.
- Yap XZ, McCall MBB, Sauerwein RW. Fast and fierce versus slow and smooth: Heterogeneity in immune responses to Plasmodium in the controlled human malaria infection model. Immunol Rev. 2020 Jan;293(1):253–269. doi: 10.1111/imr.12811
- CDC. Yellow fever vaccine recommendations. Available at https://www.cdc.gov/yellowfever/vaccine/vaccine-recommendations.html.2021.
- Gotuzzo E, Yactayo S, Córdova E. Efficacy and duration of immunity after yellow fever vaccination: systematic review on the need for a booster every 10 years. Am J Trop Med Hyg. 2013 Sep;89(3):434–444. doi: 10.4269/ajtmh.13-0264
- Roestenberg M, Teirlinck AC, McCall MB, et al. Long-term protection against malaria after experimental sporozoite inoculation: an open-label follow-up study. Lancet. 2011 May 21;377(9779):1770–1776. doi: 10.1016/S0140-6736(11)60360-7
- Teirlinck AC, McCall MB, Roestenberg M, et al. Longevity and composition of cellular immune responses following experimental Plasmodium falciparum malaria infection in humans [journal article Research support non-U S gov’t]. PLOS Pathogens. 2011 Dec;7(12):e1002389. doi: 10.1371/journal.ppat.1002389
- Zarling S, Berenzon D, Dalai S, et al. The survival of memory CD8 T cells that is mediated by IL-15 correlates with sustained protection against malaria. J Immunol. 2013 May 15;190(10):5128–5141.
- Pradel G, Garapaty S, Frevert U. Kupffer and stellate cell proteoglycans mediate malaria sporozoite targeting to the liver. Comp Hepatol. 2004 Jan 14;3(Suppl 1):S47.
- Luke TC, Hoffman SL. Rationale and plans for developing a non-replicating, metabolically active, radiation-attenuated Plasmodium falciparum sporozoite vaccine. J Exp Biol. 2003 Nov;206(Pt 21):3803–3808. doi: 10.1242/jeb.00644
- Hoffman SL, Billingsley P, James E, et al. Development of a metabolically active, non-replicating sporozoite vaccine to prevent Plasmodium falciparum malaria. Hum Vaccines. 2010;6(1):97–106. doi: 10.4161/hv.6.1.10396
- Ballou WR, Hoffman SL, Sherwood JA, et al. Safety and efficacy of a recombinant DNA Plasmodium falciparum sporozoite vaccine. Lancet. 1987 Jun 6;1(8545):1277–1281.
- Sedegah M, Beaudoin RL, Majarian WR, et al. Evaluation of vaccines designed to induce protective cellular immunity against the Plasmodium yoelii circumsporozoite protein: vaccinia, pseudorabies, and Salmonella transformed with circumsporozoite gene. Bull World Health Organ. 1990;68(Suppl):109–114.
- Sedegah M, Chiang CH, Weiss WR, et al. Recombinant pseudorabies virus carrying a plasmodium gene: herpesvirus as a new live viral vector for inducing T- and B-cell immunity. Vaccine. 1992;10(9):578–584. doi: 10.1016/0264-410X(92)90436-N
- Wang HH, Rogers WO, Kang YH, et al. Partial protection against malaria by immunization with Leishmania enriettii expressing the Plasmodium yoelii circumsporozoite protein. Mol Biochem Parasitol. 1995 Feb;69(2):139–148. doi: 10.1016/0166-6851(94)00159-K
- Sedegah M, Hedstrom R, Hobart P, et al. Protection against malaria by immunization with plasmid DNA encoding circumsporozoite protein. Proc Natl Acad Sci U S A. 1994 Oct 11;91(21):9866–9870. doi: 10.1073/pnas.91.21.9866
- Richie TL, Charoenvit Y, Wang R, et al. Clinical trial in healthy malaria-naïve adults to evaluate the safety, tolerability, immunogenicity and efficacy of MuStDO5, a five-gene, sporozoite/hepatic stage Plasmodium falciparum DNA vaccine combined with escalating dose human GM-CSF DNA. Human Vaccines Immunother. 2012;8(11):1564–1584. doi: 10.4161/hv.22129
- Wang R, Doolan DL, Le TP, et al. Induction of antigen-specific cytotoxic T lymphocytes in humans by a malaria DNA vaccine. Science. 1998 Oct 16;282(5388):476–480.
- Sedegah M, Tamminga C, McGrath S, et al. Adenovirus 5-vectored P. falciparum vaccine expressing CSP and AMA1. Part A: safety and immunogenicity in seronegative adults. PLoS One. 2011;6(10):e24586. doi: 10.1371/journal.pone.0024586
- Tamminga C, Sedegah M, Regis D, et al. Adenovirus-5-vectored P. falciparum vaccine expressing CSP and AMA1. Part B: safety, immunogenicity and protective efficacy of the CSP component. PLoS One. 2011;6(10):e25868. doi: 10.1371/journal.pone.0025868
- Sedegah M, Jones TR, Kaur M, et al. Boosting with recombinant vaccinia increases immunogenicity and protective efficacy of malaria DNA vaccine. Proc Natl Acad Sci U S A. 1998 Jun 23;95(13):7648–7653.
- Epstein JE, Charoenvit Y, Kester KE, et al. Safety, tolerability, and antibody responses in humans after sequential immunization with a PfCSP DNA vaccine followed by the recombinant protein vaccine RTS, S/AS02A. Vaccine. 2004 Apr 16;22(13–14):1592–1603.
- Chuang I, Sedegah M, Cicatelli S, et al. DNA prime/Adenovirus boost malaria vaccine encoding P. falciparum CSP and AMA1 induces sterile protection associated with cell-mediated immunity. PLoS One. 2013;8(2):e55571. doi: 10.1371/journal.pone.0055571
- Sedegah M, Kim Y, Peters B, et al. Identification and localization of minimal MHC-restricted CD8+ T cell epitopes within the Plasmodium falciparum AMA1 protein. Malar J. 2010 Aug 24;9(1):241.
- Sedegah M, Kim Y, Ganeshan H, et al. Identification of minimal human MHC-restricted CD8+ T-cell epitopes within the Plasmodium falciparum circumsporozoite protein (CSP). Malar J. 2013;12(1):185. doi: 10.1186/1475-2875-12-185
- Davies L “Ghana is first country to approve Oxford malaria vaccine.” The Guardian, 13 APR 2023, https://www.theguardian.com/global-development/2023/apr/13/ghana-is-first-country-to-approve-oxford-r21-malaria-vaccine.
- Dzirutwe M “Nigeria regulator grants approval to Oxford’s malaria vaccine.” Reuters, 17 APR 2023, https://www.reuters.com/world/africa/nigeria-regulator-grants-approval-oxfords-malaria-vaccine-2023-04-17/
- Regules JA, Cicatelli SB, Bennett JW, et al. Fractional third and Fourth dose of RTS,S/AS01 malaria candidate vaccine: A Phase 2a controlled human malaria parasite infection and immunogenicity study. J Infect Dis. 2016 Sep 1;214(5):762–771.
- Moon JE, Ockenhouse C, Regules JA, et al. A Phase IIa controlled human malaria infection and immunogenicity study of RTS,S/AS01E and RTS,S/AS01B delayed fractional dose regimens in malaria-naive adults. J Infect Dis. 2020 Oct 13;222(10):1681–1691.
- Datoo MS, Natama MH, Somé A, et al. Efficacy of a low-dose candidate malaria vaccine, R21 in adjuvant Matrix-M, with seasonal administration to children in Burkina Faso: a randomised controlled trial. Lancet. 2021 May 5;397(10287):1809–1818.
- Morzaria S, Williamson S Live vaccines for Theileria parva- deployment in eastern, central and southern Africa - Proceedings of an FAO/OAU-IBAR/ILRI workshop held at ILRI, Nairobi, Kenya. In: ILRI (International Livestock Research Institute); Nairobi, Kenya, p. 166. 1999 10-12 Mar 1997.
- Steinaa L, Svitek N, Awino E, et al. Immunization with one Theileria parva strain results in similar level of CTL strain-specificity and protection compared to immunization with the three-component Muguga cocktail in MHC-matched animals. BMC Vet Res. 2018 May 2;14(1):145.
- Moser KA, Drabek EF, Dwivedi A, et al. Strains used in whole organism Plasmodium falciparum vaccine trials differ in genome structure, sequence, and immunogenic potential. Genome Med. 2020 Jan 8;12(1):6.
- Silva JC, Dwivedi A, Moser KA, et al. Plasmodium falciparum 7G8 challenge provides conservative prediction of efficacy of PfNF54-based PfSPZ Vaccine in Africa. Nat Commun. 2022 Jun 13;13(1):3390. doi: 10.1038/s41467-022-30882-8
- Diawara H. Phase 2 safety and efficacy evaluation of radiation attenuated Plasmodium falciparum sporozoite (PfSPZ) vaccine in healthy African adult women of childbearing potential in Ouélessébougou, Mali. Annual Meeting of the American Society of Tropical Medicine and Hygiene. Oral presentation on Friday, Nov 19, 2021 at 11: 05 am. Am J Trop Med Hyg. 2021 Nov;105(5):Abstract 0294, 93.
- Roestenberg M, Bijker EM, Sim BK, et al. Controlled human malaria infections by intradermal injection of cryopreserved Plasmodium falciparum sporozoites. Am J Trop Med Hyg. 2013 Jan;88(1):5–13. doi: 10.4269/ajtmh.2012.12-0613
- Mordmüller B, Supan C, Sim KL, et al. Direct venous inoculation of Plasmodium falciparum sporozoites for controlled human malaria infection: a dose-finding trial in two centres. Malar J. 2015;14(1):117. doi: 10.1186/s12936-015-0628-0
- Garcia CR, Manzi F, Tediosi F, et al. Comparative cost models of a liquid nitrogen vapor phase (LNVP) cold chain-distributed cryopreserved malaria vaccine vs. a conventional vaccine. Vaccine. 2013 Jan 2;31(2):380–386. doi: 10.1016/j.vaccine.2012.10.109
- James ER. Disrupting vaccine logistics. Int Health. 2021 Apr 27;13(3):211–214.
- Sheehy SH, Spencer AJ, Douglas AD, et al. Optimising controlled human malaria infection studies using cryopreserved P. falciparum parasites administered by needle and syringe. PLoS One. 2013;8(6):e65960. doi: 10.1371/journal.pone.0065960
- Hodgson SH, Juma EA, Salim A, et al. Evaluating controlled human malaria infection in Kenyan adults with varying degrees of prior exposure to Plasmodium falciparum using sporozoites administered by intramuscular injection [clinical trial]. Front Microbiol. 2014 Dec 12;5:686.
- Shekalaghe S, Rutaihwa M, Billingsley PF, et al. Controlled human malaria infection of Tanzanians by intradermal injection of aseptic, purified, cryopreserved Plasmodium falciparum sporozoites. Am J Trop Med Hyg. 2014 Sep 3;91(3):471–480. doi: 10.4269/ajtmh.14-0119
- Gomez-Perez GP, Legarda A, Munoz J, et al. Controlled human malaria infection by intramuscular and direct venous inoculation of cryopreserved Plasmodium falciparum sporozoites in malaria-naïve volunteers: effect of injection volume and dose on infectivity rates. Malar J. 2015;14(1):306. doi: 10.1186/s12936-015-0817-x
- Lyke KE, Laurens MB, Strauss K, et al. Optimizing intradermal administration of cryopreserved Plasmodium falciparum sporozoites in controlled human malaria infection. Am J Trop Med Hyg. 2015 Sep 28;93(6):1274–1284.
- Bastiaens GJ, van Meer MP, Scholzen A, et al. Safety, immunogenicity, and protective efficacy of intradermal immunization with aseptic, purified, cryopreserved Plasmodium falciparum sporozoites in volunteers under chloroquine prophylaxis: a randomized controlled trial. Am J Trop Med Hyg. 2016 Dec 28;94(3):663–673.
- Laurens MB, Berry AA, Travassos MA, et al. Dose dependent infectivity of aseptic, purified, cryopreserved Plasmodium falciparum 7G8 sporozoites in malaria-naive adults. J Infect Dis. 2019 Aug 16;220(12):1962–1966.
- Jongo SA, Shekalage SA, Church LWP, et al. Safety, immunogenicity, and protective efficacy against controlled human malaria infection of Plasmodium falciparum sporozoite vaccine in Tanzanian adults. Am J Trop Med Hyg. 2018 Jun 25;99(2):338–349.
- Dejon-Agobe JC, Ateba-Ngoa U, Lalremruata A, et al. Controlled human malaria infection of healthy lifelong malaria-exposed adults to assess safety, immunogenicity and efficacy of the asexual blood stage malaria vaccine candidate GMZ2. Clin Infect Dis. 2019 Dec 18;69(8):1377–1384.
- Jongo SA, Urbano V, Church LWP, et al. Immunogenicity and protective efficacy of radiation-attenuated and chemo-attenuated PfSPZ vaccines in Equatoguinean adults. Am J Trop Med Hyg. 2021 Jan;104(1):283–293. doi: 10.4269/ajtmh.20-0435
- Murphy SC, Deye GA, Sim BKL, et al. PfSPZ-CVac efficacy against malaria increases from 0% to 75% when administered in the absence of erythrocyte stage parasitemia: a randomized, placebo-controlled trial with controlled human malaria infection. PLOS Pathog. 2021 May;17(5):e1009594. doi: 10.1371/journal.ppat.1009594
- Sulyok Z, Fendel R, Eder B, et al. Heterologous protection against malaria by a simple chemoattenuated PfSPZ vaccine regimen in a randomized trial. Nat Commun. 2021 May 4;12(1):2518.
- Jongo SA, Church LWP, Nchama V, et al. Multi-dose priming regimens of PfSPZ Vaccine: safety and efficacy against controlled human malaria infection in Equatoguinean adults. Am J Trop Med Hyg. 2022 Feb 7;106(4):1215–1226.
- Sulyok M, Ruckle T, Roth A, et al. DSM265 for Plasmodium falciparum chemoprophylaxis: a randomised, double blinded, phase 1 trial with controlled human malaria infection. Lancet Infect Dis. 2017 Mar 28;17(6):636–644.
- Murphy SC, Duke ER, Shipman KJ, et al. A randomized trial evaluating the prophylactic activity of DSM265 against preerythrocytic Plasmodium falciparum infection during controlled human malarial infection by mosquito bites and direct venous inoculation. J Infect Dis. 2018 Feb 14;217(5):693–702.
- Lell B, Mordmüller B, Dejon Agobe JC, et al. Impact of sickle cell trait and naturally acquired immunity on uncomplicated malaria after controlled human malaria infection in adults in Gabon. Am J Trop Med Hyg. 2018 Feb;98(2):508–515. doi: 10.4269/ajtmh.17-0343
- Achan J, Reuling I, Yap XZ, et al. Serologic markers of previous malaria exposure and functional antibodies inhibiting parasite growth are associated with parasite kinetics following a Plasmodium falciparum controlled human infection. Clin Infect Dis. 2019 Aug 12;70(12):2544–2552.
- Kapulu MC, Njuguna P, Hamaluba MM. Controlled human malaria infection in semi-immune Kenyan adults (CHMI-SIKA): a study protocol to investigate in vivo Plasmodium falciparum malaria parasite growth in the context of pre-existing immunity. Wellcome Open Res. 2019;3:155. doi: 10.12688/wellcomeopenres.14909.2
- Kapulu MC, Njuguna P, Hamaluba M, et al. Safety and PCR monitoring in 161 semi-immune Kenyan adults following controlled human malaria infection. JCI Insight. 2021 Sep 8;6(17). doi: 10.1172/jci.insight.146443
- Jongo SA, Church LWP, Mtoro AT, et al. Safety and differential antibody and T-cell responses to the Plasmodium falciparum sporozoite malaria vaccine, PfSPZ Vaccine, by age in Tanzanian adults, adolescents, children, and infants. Am J Trop Med Hyg. 2019 Jun;100(6):1433–1444. doi: 10.4269/ajtmh.18-0835
- Steinhardt LC, Richie TL, Yego R, et al. Safety, tolerability, and immunogenicity of PfSPZ Vaccine administered by direct venous inoculation to infants and young children: findings from an age de-escalation, dose-escalation double-blinded randomized, controlled study in western Kenya. Clin Infect Dis. 2020 Sep 26;71(14):1063–1071.
- Oneko M, Cherop YR, Sang T, et al. Feasibility of direct venous inoculation of the radiation-attenuated Plasmodium falciparum whole sporozoite vaccine in children and infants in Siaya, western Kenya. Vaccine. 2020 Jun 15;38(29):4592–4600. doi: 10.1016/j.vaccine.2020.05.008
- Achieng F, Rosen JG, Cherop RY, et al. Caregiver and community perceptions and experiences participating in an infant malaria prevention trial of PfSPZ Vaccine administered by direct venous inoculation: a qualitative study in Siaya County, western Kenya. Malar J. 2020 Jun 24;19(1):226.
- Gola A, Silman D, Walters AA, et al. Prime and target immunization protects against liver-stage malaria in mice. Sci Transl Med. 2018 Sep 26;10(460). doi: 10.1126/scitranslmed.aap9128
- Darrah PA, Zeppa JJ, Maiello P, et al. Prevention of tuberculosis in macaques after intravenous BCG immunization. Nature. 2020 Jan;577(7788):95–102. doi: 10.1038/s41586-019-1817-8
- van Buskirk KM, O’Neill MT, De La Vega P, et al. Preerythrocytic, live-attenuated Plasmodium falciparum vaccine candidates by design. Proc Natl Acad Sci, USA. 2009;106(31):13004–13009. doi: 10.1073/pnas.0906387106
- Murphy SC, Vaughan AM, Kublin JG, et al. A genetically engineered Plasmodium falciparum parasite vaccine provides protection from controlled human malaria infection. Sci Transl Med. 2022 Aug 24;14(659):eabn9709.
- Butler NS, Schmidt NW, Vaughan AM, et al. Superior antimalarial immunity after vaccination with late liver stage-arresting genetically attenuated parasites. Cell Host Microbe. 2011 Jun 16;9(6):451–462.
- Goswami D, Betz W, Locham NK, et al. A replication-competent late liver stage–attenuated human malaria parasite. JCI Insight. 2020 Aug 26;5(13):e135589. doi: 10.1172/jci.insight.135589
- Roestenberg M, Walk J, van der Boor SC, et al. A double-blind, placebo-controlled phase 1/2a trial of the genetically attenuated malaria vaccine PfSPZ-GA1. Sci Transl Med. 2020 May 20;12(544):eaaz5629. doi: 10.1126/scitranslmed.aaz5629
- Franke-Fayard B. Development and clinical evaluation of genetically attenuated parasite that arrests late in the liver. In: Oral presentation Tues, Nov 1, 10:45 am, 2022 Annual Meeting of the American Society for Tropical Medicine and Hygiene, Seattle, Washington. 2022.
- James ER, Wen Y, Overby J, et al. Cryopreservation of Anopheles stephensi embryos. Sci Rep. 2022 Jan 7;12(1):43.
- Phalen H, Vagdargi P, Schrum ML, et al. A mosquito Pick-and-Place system for PfSPZ-based malaria vaccine production. IEEE Trans Autom Sci Eng. 2021 Jan;18(1):299–310. doi: 10.1109/TASE.2020.2992131
- Blight J, Sala KA, Atcheson E, et al. Dissection-independent production of a protective whole-sporozoite malaria vaccine. bioRxiv. 2020:2020.06.22.164756.
- Warburg A, Miller LH. Sporogonic development of a malaria parasite in vitro. Science. 1992 Jan 24;255(5043):448–450.
- Imkeller K, Scally SW, Bosch A, et al. Antihomotypic affinity maturation improves human B cell responses against a repetitive epitope. Science. 2018 Jun 7;360(6395):1358–1362.
- Kisalu NK, Idris AH, Weidle C, et al. A human monoclonal antibody prevents malaria infection by targeting a new site of vulnerability on the parasite. Nat Med. 2018 May;24(4):408–416. doi: 10.1038/nm.4512
- Tan J, Sack BK, Oyen D, et al. A public antibody lineage that potently inhibits malaria infection through dual binding to the circumsporozoite protein. Nat Med. 2018 May;24(4):401–407. doi: 10.1038/nm.4513
- Wu RL, Idris AH, Berkowitz NM, et al. Low-dose subcutaneous or intravenous monoclonal antibody to prevent malaria. N Engl J Med. 2022 Aug 4;387(5):397–407.
- Gaudinski MR, Berkowitz NM, Idris AH, et al. A monoclonal antibody for malaria prevention. N Engl J Med. 2021 Aug 26;385(9):803–814.
- Kayentao K, Ongoiba A, Preston AC, et al. Safety and efficacy of a monoclonal antibody against malaria in Mali. N Engl J Med. 2022 Nov 17;387(20):1833–1842.
- Vaughan AM, Mikolajczak SA, Wilson EM, et al. Complete Plasmodium falciparum liver-stage development in liver-chimeric mice. J Clin Invest. 2012 Oct 1;122(10):3618–3628.
- Frischknecht F, Matuschewski K. Plasmodium sporozoite biology. Cold Spring Harb Perspect Med. 2017 May 1;7(5):a025478.
- Vaughan AM, Kappe SHI. Malaria parasite liver infection and exoerythrocytic biology. Cold Spring Harb Perspect Med. 2017 Jun 1;7(6):a025486.
- Nganou-Makamdop K, Sauerwein RW. Liver or blood-stage arrest during malaria sporozoite immunization: the later the better? Trends Parasitol. 2013 Jun;29(6):304–310. doi: 10.1016/j.pt.2013.03.008
- Olotu A, Urbano V, Hamad A, et al. Advancing Global Health through development and clinical trials partnerships: a randomized, placebo-controlled, double-blind assessment of safety, tolerability, and immunogenicity of Plasmodium falciparum sporozoites vaccine for malaria in healthy Equatoguinean men. Am J Trop Med Hyg. 2018 Oct 30;98(1):308–318.
- Lyke KE, Singer A, Berry AA, et al. Multidose priming and delayed boosting improve PfSPZ Vaccine efficacy against heterologous P. falciparum controlled human malaria infection. Clin Infect Dis. 2021 Sep 12;73(7):e2424–e2435.
- Oneko M, Steinhardt LC, Yego R, et al. Safety, immunogenicity and efficacy of PfSPZ Vaccine against malaria in infants in western Kenya: a double-blind, randomized, placebo-controlled phase 2 trial. Nat Med. 2021 Sep;27(9):1636–1645. doi: 10.1038/s41591-021-01470-y
- Spring M, Murphy J, Nielsen R, et al. First-in-human evaluation of genetically attenuated Plasmodium falciparum sporozoites administered by bite of Anopheles mosquitoes to adult volunteers. Vaccine. 2013 Oct 9;31(43):4975–4983. doi: 10.1016/j.vaccine.2013.08.007
- Kublin JG, Mikolajczak SA, Sack BK, et al. Complete attenuation of genetically engineered Plasmodium falciparum sporozoites in human subjects. Sci Transl Med. 2017 Jan 4;9(371):eaad9099.
- Coulibaly D, Kone AK, Traore K, et al. PfSPZ-CVac malaria vaccine demonstrates safety among malaria-experienced adults: A randomized, controlled phase 1 trial. EClinicalMedicine. 2022 Oct;52:101579.
- Borrmann S, Sulyok Z, Müller K, et al. Mapping of safe and early chemo-attenuated live Plasmodium falciparum immunization identifies immune signature of vaccine efficacy. bioRxiv. 2020:2020.09.14.296152.
- Goswami D, Minkah NK, Kappe SHI. Designer parasites: Genetically engineered Plasmodium as vaccines to prevent malaria infection. J Immunol. 2019 Jan 1;202(1):20–28.
- Franke-Fayard B, Marin-Mogollon C, Geurten FJA, et al. Creation and preclinical evaluation of genetically attenuated malaria parasites arresting growth late in the liver. NPJ Vaccines. 2022 Nov 4;7(1):139.
- Sim BKL Development and clinical evaluation of genetically attenuated, replication competent, late arresting (LARC) PfSPZ vaccines manufactured in mosquitoes or in vitro using bioreactors. In: Oral presentation Tues, Nov 1, 11:25 am, 2022 Annual Meeting of the American Society for Tropical Medicine and Hygiene, Seattle, Washington.
- Mueller AK, Camargo N, Kaiser K, et al. Plasmodium liver stage developmental arrest by depletion of a protein at the parasite-host interface. Proc Natl Acad Sci U S A. 2005 Feb 22;102(8):3022–3027.
- Labaied M, Harupa A, Dumpit RF, et al. Plasmodium yoelii sporozoites with simultaneous deletion of P52 and P36 are completely attenuated and confer sterile immunity against infection. Infect Immun. 2007 Aug;75(8):3758–3768. doi: 10.1128/IAI.00225-07
- Hempelmann E, Krafts K. Bad air, amulets and mosquitoes: 2,000 years of changing perspectives on malaria. Malar J. 2013 Jul 9;12(1):232.
- Hickey BW, Lumsden JM, Reyes S, et al. Mosquito bite immunization with radiation-attenuated Plasmodium falciparum sporozoites: safety, tolerability, protective efficacy and humoral immunogenicity. Malar J. 2016;15(1):377. doi: 10.1186/s12936-016-1435-y
- Jin Y, Kebaier C, Vanderberg J. Direct microscopic quantification of dynamics of Plasmodium berghei sporozoite transmission from mosquitoes to mice. Infect Immun. 2007 Nov;75(11):5532–5539. doi: 10.1128/IAI.00600-07
- Choumet V, Attout T, Chartier L, et al. Visualizing non infectious and infectious Anopheles gambiae blood feedings in naive and saliva-immunized mice. PLoS One. 2012;7(12):e50464. doi: 10.1371/journal.pone.0050464
- Gueirard P, Tavares J, Thiberge S, et al. Development of the malaria parasite in the skin of the mammalian host. Proc Natl Acad Sci U S A. 2010 Oct 26;107(43):18640–18645.
- Haeberlein S, Chevalley-Maurel S, Ozir-Fazalalikhan A, et al. Protective immunity differs between routes of administration of attenuated malaria parasites independent of parasite liver load. Sci Rep. 2017 Sep 4;7(1):10372.
- Guilbride DL, Guilbride PD, Gawlinski P. Malaria’s deadly secret: a skin stage. Trends Parasitol. 2012 Apr;28(4):142–150. doi: 10.1016/j.pt.2012.01.002
- Winkel BMF, Pelgrom LR, van Schuijlenburg R, et al. Plasmodium sporozoites induce regulatory macrophages. PLOS Pathog. 2020 Sep;16(9):e1008799. doi: 10.1371/journal.ppat.1008799
- Amino R, Thiberge S, Martin B, et al. Quantitative imaging of Plasmodium transmission from mosquito to mammal. Nat Med. 2006 Feb;12(2):220–224. doi: 10.1038/nm1350
- Chakravarty S, Cockburn IA, Kuk S, et al. CD8+ T lymphocytes protective against malaria liver stages are primed in skin-draining lymph nodes. Nat Med. 2007;13(9):1035–1041. doi: 10.1038/nm1628
- Spitalny GL, Nussenzweig RS Effect of various routes of immunization and methods of parasite attenuation on the development of protection against sporozoite-induced rodent malaria. In: Proceedings of the Helminthological Society of Washington, Washington, BC; 39. 506–514. 1972.
- Beaudoin RL, Strome CPA, Mitchell F, et al. Plasmodium berghei: immunization of mice against the ANKA strain using the unaltered sporozoite as an antigen. Exp Parasitol. 1977;42(1):1–5. doi: 10.1016/0014-4894(77)90054-6
- Hodgson SH, Juma E, Salim A, et al. Lessons learnt from the first controlled human malaria infection study conducted in Nairobi, Kenya. Malar J. 2015 Jan 28;14(1):182.
- Epstein JE, Paolino KM, Richie TL, et al. Protection against Plasmodium falciparum malaria by PfSPZ Vaccine. JCI Insight. 2017 Jan 12;2(1):e89154. doi: 10.1172/jci.insight.89154
- Burkot TR, Williams JL, Schneider I. Infectivity to mosquitoes of Plasmodium falciparum clones grown in vitro from the same isolate. Trans R Soc Trop Med Hyg. 1984;78(3):339–341. doi: 10.1016/0035-9203(84)90114-7
- Delemarre BJ, van der Kaay HJ. Tropical malaria contracted the natural way in the Netherlands. Ned Tijdschr Geneeskd. 1979 Nov 17;123(46):1981–1982.
- Ponnudurai T, Leeuwenberg AD, Meuwissen JH. Chloroquine sensitivity of isolates of Plasmodium falciparum adapted to in vitro culture. Trop Geogr Med. 1981;33(1):50–54.
- Preston MD, Campino S, Assefa SA, et al. A barcode of organellar genome polymorphisms identifies the geographic origin of Plasmodium falciparum strains. Nat Commun. 2014 Jun 13;5(1):4052.
- Wahid R, Fresnay S, Levine MM, et al. Immunization with Ty21a live oral typhoid vaccine elicits cross reactive multifunctional CD8+ T-cell responses against Salmonella enterica serovar Typhi, S. Paratyphi A, and S. Paratyphi B in humans. Mucosal Immunol. 2015 Nov;8(6):1349–1359. doi: 10.1038/mi.2015.24
- Levine MM, Ferreccio C, Black RE, et al. Large-scale field trial of Ty21a live oral typhoid vaccine in enteric-coated capsule formulation. Lancet. 1987 May 9;1(8541):1049–1052.
- Simon AK, Hollander GA, McMichael A. Evolution of the immune system in humans from infancy to old age. Proc Biol Sci. 2015 Dec 22;282(1821):20143085.
- Fried M, Duffy PE. Designing a VAR2CSA-based vaccine to prevent placental malaria. Vaccine. 2015 Dec 22;33(52):7483–7488.
- Healy SA, Fried M, Richie T, et al. Malaria vaccine trials in pregnant women: an imperative without precedent. Vaccine. 2019 Feb 4;37(6):763–770.
- Gamain B, Chêne A, Viebig NK, et al. Progress and insights toward an effective placental malaria vaccine. Front Immunol. 2021;12:634508. doi: 10.3389/fimmu.2021.634508
- Orjih AU, Cochrane AH, Nussenzweig RS. Comparative studies on the immunogenicity of infective and attenuated sporozoites of Plasmodium berghei. Trans R Soc Trop Med Hyg. 1982;76(1):57–61. doi: 10.1016/0035-9203(82)90019-0
- Roestenberg M, McCall M, Hopman J, et al. Protection against a malaria challenge by sporozoite inoculation. N Engl J Med. 2009 Jul 30;361(5):468–477. doi: 10.1056/NEJMoa0805832
- Bijker EM, Teirlinck AC, Schats R, et al. Cytotoxic markers associate with protection against malaria in human volunteers immunized with Plasmodium falciparum sporozoites. J Infect Dis. 2014 Nov 15;210(10):1605–1615.
- Schats R, Bijker EM, van Gemert GJ, et al. Heterologous protection against malaria after immunization with Plasmodium falciparum sporozoites. PLoS One. 2015;10(5):e0124243. doi: 10.1371/journal.pone.0124243
- Bijker EM, Schats R, Obiero JM, et al. Sporozoite immunization of human volunteers under mefloquine prophylaxis is safe, immunogenic and protective: a double-blind randomized controlled clinical trial. PLoS One. 2014;9(11):e112910. doi: 10.1371/journal.pone.0112910
- Ibanez J, Fendel R, Lorenz FR, et al. Efficacy, T cell activation and antibody responses in accelerated Plasmodium falciparum sporozoite chemoprophylaxis vaccine regimens. NPJ Vaccines. 2022 May 31;7(1):59.
- Healy SA, Murphy SC, Hume JCC, et al. Chemoprophylaxis vaccination: phase I study to explore stage-specific immunity to Plasmodium falciparum in US adults. Clin Infect Dis. 2020 Sep 12;71(6):1481–1490.
- Friesen J, Silvie O, Putrianti ED, et al. Natural immunization against malaria: causal prophylaxis with antibiotics. Sci Transl Med. 2010 Jul 14;2(40):40ra49.
- Mueller AK, Labaied M, Kappe SH, et al. Genetically modified Plasmodium parasites as a protective experimental malaria vaccine. Nature. 2005 Jan 13;433(7022):164–167. doi: 10.1038/nature03188
- Tarun AS, Dumpit RF, Camargo N, et al. Protracted sterile protection with Plasmodium yoelii pre-erythrocytic genetically attenuated parasite malaria vaccines is independent of significant liver-stage persistence and is mediated by CD8+ T cells. J Infect Dis. 2007;196(4):608–616. doi: 10.1086/519742
- van Dijk MR, Douradinha B, Franke-Fayard B, et al. Genetically attenuated, P36p-deficient malarial sporozoites induce protective immunity and apoptosis of infected liver cells. Proc Natl Acad Sci U S A. 2005 Aug 23;102(34):12194–12199. doi: 10.1073/pnas.0500925102
- Ishino T, Chinzei Y, Yuda M. Two proteins with 6-cys motifs are required for malarial parasites to commit to infection of the hepatocyte. Mol Microbiol. 2005 Dec;58(5):1264–1275. doi: 10.1111/j.1365-2958.2005.04801.x
- Douradinha B, van Dijk MR, Ataide R, et al. Genetically attenuated P36p-deficient Plasmodium berghei sporozoites confer long-lasting and partial cross-species protection. Int J Parasitol. 2007 Nov;37(13):1511–1519. doi: 10.1016/j.ijpara.2007.05.005
- Annoura T, van Schaijk BC, Ploemen IH, et al. Two Plasmodium 6-Cys family-related proteins have distinct and critical roles in liver-stage development. FASEB J. 2014 Feb 7;28(5):2158–2170.
- van Schaijk BC, Ploemen IH, Annoura T, et al. A genetically attenuated malaria vaccine candidate based on gene-deficient sporozoites. Elife. 2014 Nov 19;3:e03582. doi:10.7554/eLife.03582
- Aly AS, Mikolajczak SA, Rivera HS, et al. Targeted deletion of SAP1 abolishes the expression of infectivity factors necessary for successful malaria parasite liver infection. Mol Microbiol. 2008 Jul;69(1):152–163. doi: 10.1111/j.1365-2958.2008.06271.x
- Silvie O, Goetz K, Matuschewski K, et al. A sporozoite asparagine-rich protein controls initiation of Plasmodium liver stage development. PLOS Pathogens. 2008 Jun;4(6):e1000086. doi: 10.1371/journal.ppat.1000086
- Othman AS, Marin-Mogollon C, Salman AM, et al. The use of transgenic parasites in malaria vaccine research. Expert Rev Vaccines. 2017 Jul;16(7):1–13. doi: 10.1080/14760584.2017.1333426
- Jobe O, Lumsden J, Mueller AK, et al. Genetically attenuated Plasmodium berghei liver stages induce sterile protracted protection that is mediated by major histocompatibility complex class I-dependent interferon-gamma-producing CD8+ T cells. J Infect Dis. 2007 Aug 15;196(4):599–607.
- van Schaijk BCL, Janse CJ, van Gemert GJ, et al. Gene disruption of Plasmodium falciparum p52 results in attenuation of malaria liver stage development in cultured primary human hepatocytes. PLoS One. 2008;3(10):e3549. doi: 10.1371/journal.pone.0003549
- Mikolajczak SA, Lakshmanan V, Fishbaugher M, et al. A next-generation genetically attenuated Plasmodium falciparum parasite created by triple gene deletion. Mol Ther. 2014 Sep;22(9):1707–1715. doi: 10.1038/mt.2014.85
- Kreutzfeld O, Müller K, Matuschewski K. Engineering of genetically arrested parasites (GAPs) for a precision malaria vaccine. Front Cell Infect Microbiol. 2017;7:198. doi: 10.3389/fcimb.2017.00198
- Dankwa DA, Davis MJ, Kappe SHI, et al. A Plasmodium yoelii Mei2-like RNA binding protein is essential for completion of liver stage schizogony. Infect Immun. 2016 May;84(5):1336–1345. doi: 10.1128/IAI.01417-15
- Goswami D, Arredondo SA, Betz W, et al. A conserved Plasmodium protein that localizes to liver stage nuclei is critical for late liver stage development. bioRxiv. 2022:2022.12.13.519845.
- Sack BK, Keitany GJ, Vaughan AM, et al. Mechanisms of stage-transcending protection following immunization of mice with late liver stage-arresting genetically attenuated malaria parasites. PLOS Pathog. 2015 May;11(5):e1004855. doi: 10.1371/journal.ppat.1004855
- Dienstag JL, Stevens CE, Bhan AK, et al. Hepatitis B vaccine administered to chronic carriers of hepatitis b surface antigen. Ann Intern Med. 1982 May;96(5):575–579. doi: 10.7326/0003-4819-96-5-575
- Pol S, Driss F, Michel ML, et al. Specific vaccine therapy in chronic hepatitis B infection. Lancet. 1994 Jul 30;344(8918):342.
- Ho M, Webster HK, Looareesuwan S, et al. Antigen-specific immunosuppression in human malaria due to Plasmodium falciparum. J Infect Dis. 1986;153(4):763–771. doi: 10.1093/infdis/153.4.763
- McGregor IA, Barr M. Antibody response to tetanus toxoid inoculation in malarious and non-malarious Gambian children. Trans R Soc Trop Med Hyg. 1962;56(5):364–367. doi: 10.1016/0035-9203(62)90005-6
- Greenwood BM, Bradley-More AM, Palit A, et al. Immunosuppression in children with malaria. Lancet. 1972;i:169–172.
- Williamson WA, Greenwood BM. Impairment of the immune response to vaccination after acute malaria. Lancet. 1978;1(8078):1328–1329. doi: 10.1016/S0140-6736(78)92403-0
- Abu-Raddad LJ, Patnaik P, Kublin JG. Dual infection with HIV and malaria fuels the spread of both diseases in sub-Saharan Africa. Science. 2006 Dec 8;314(5805):1603–1606.
- Nyirenda TS, Nyirenda JT, Tembo DL, et al. Loss of humoral and cellular immunity to invasive nontyphoidal Salmonella during current or convalescent Plasmodium falciparum infection in Malawian children. Clin Vaccine Immunol. 2017 Jul;24(7). doi: 10.1128/CVI.00057-17
- Casares S, Richie TL. Immune evasion by malaria parasites: a challenge for vaccine development. Curr Opin Immunol. 2009 Jun;21(3):321–330. doi: 10.1016/j.coi.2009.05.015
- Chatterjee D, Lewis FJ, Sutton HJ, et al. Avid binding by B cells to the Plasmodium circumsporozoite protein repeat suppresses responses to protective subdominant epitopes. Cell Rep. 2021 Apr 13;35(2):108996.
- Egan A, Waterfall M, Pinder M, et al. Characterization of human T- and B-cell epitopes in the C terminus of Plasmodium falciparum merozoite surface protein 1: evidence for poor T-cell recognition of polypeptides with numerous disulfide bonds. Infect Immun. 1997 Aug;65(8):3024–3031. doi: 10.1128/iai.65.8.3024-3031.1997
- Rathore D, Nagarkatti R, Jani D, et al. An immunologically cryptic epitope of Plasmodium falciparum circumsporozoite protein facilitates liver cell recognition and induces protective antibodies that block liver cell invasion. J Biol Chem. 2005 May 27;280(21):20524–20529.
- Lee EA, Flanagan KL, Minigo G, et al. Dimorphic Plasmodium falciparum merozoite surface protein-1 epitopes turn off memory T cells and interfere with T cell priming. Eur J Immunol. 2006 May;36(5):1168–1178. doi: 10.1002/eji.200526010
- D’Ombrain MC, Voss TS, Maier AG, et al. Plasmodium falciparum erythrocyte membrane protein-1 specifically suppresses early production of host interferon-gamma. Cell Host Microbe. 2007 Aug 16;2(2):130–138.
- Steers N, Schwenk R, Bacon DJ, et al. The immune status of Kupffer cells profoundly influences their responses to infectious Plasmodium berghei sporozoites. Eur J Immunol. 2005 Aug;35(8):2335–2346. doi: 10.1002/eji.200425680
- Loughland JR, Minigo G, Burel J, et al. Profoundly reduced CD1c+ myeloid dendritic cell HLA-DR and CD86 expression and increased tumor necrosis factor production in experimental human blood-stage malaria infection. Infect Immun. 2016 May;84(5):1403–1412. doi: 10.1128/IAI.01522-15
- Illingworth J, Butler NS, Roetynck S, et al. Chronic exposure to Plasmodium falciparum is associated with phenotypic evidence of B and T cell exhaustion. J Immunol. 2013 Feb 1;190(3):1038–1047.
- Sullivan RT, Kim CC, Fontana MF, et al. FCRL5 delineates functionally impaired memory B cells associated with Plasmodium falciparum exposure. PLOS Pathog. 2015 May;11(5):e1004894. doi: 10.1371/journal.ppat.1004894
- Horne-Debets JM, Faleiro R, Karunarathne DS, et al. PD-1 dependent exhaustion of CD8+ T cells drives chronic malaria. Cell Rep. 2013 Dec 12;5(5):1204–1213.
- Saito F, Hirayasu K, Satoh T, et al. Immune evasion of Plasmodium falciparum by RIFIN via inhibitory receptors. Nature. 2017 Dec 7;552(7683):101–105.
- Xu H, Wipasa J, Yan H, et al. The mechanism and significance of deletion of parasite-specific CD4(+) T cells in malaria infection. J Exp Med. 2002 Apr 1;195(7):881–892.
- Walther M, Tongren JE, Andrews L, et al. Upregulation of TGF-beta, FOXP3, and CD4+CD25+ regulatory T cells correlates with more rapid parasite growth in human malaria infection. Immunity. 2005 Sep;23(3):287–296. doi: 10.1016/j.immuni.2005.08.006
- Minigo G, Woodberry T, Piera KA, et al. Parasite-dependent expansion of TNF receptor II-positive regulatory T cells with enhanced suppressive activity in adults with severe malaria. PLOS Pathog. 2009 Apr;5(4):e1000402. doi: 10.1371/journal.ppat.1000402
- Skorokhod OA, Alessio M, Mordmüller B, et al. Hemozoin (malarial pigment) inhibits differentiation and maturation of human monocyte-derived dendritic cells: a peroxisome proliferator-activated receptor-gamma-mediated effect. J Immunol. 2004 Sep 15;173(6):4066–4074.
- Scorza T, Magez S, Brys L, et al. Hemozoin is a key factor in the induction of malaria-associated immunosuppression. Parasite Immunol. 1999 Nov;21(11):545–554. doi: 10.1046/j.1365-3024.1999.00254.x
- Tall A, Sokhna C, Perrault R, et al. Assessment of the relative success of sporozoite inoculations in individuals exposed to moderate seasonal transmission. Malaria J. 2009;8:161. doi: 10.1186/1475-2875-8-161
- Orjih AU, Nussenzweig RS. Plasmodium berghei: suppression of antibody response to sporozoite stage by acute blood stage infection. Clin Exp Immunol. 1980;38:1–8.
- Ocana-Morgner C, Mota MM, Rodriguez A. Malaria blood stage suppression of liver stage immunity by dendritic cells. J Exp Med. 2003;197(2):143–151. doi: 10.1084/jem.20021072
- Watson F, Shears M, Matsubara J, et al. Cryopreserved sporozoites with and without the glycolipid adjuvant 7DW8-5 protect in prime-and-trap malaria vaccination. Am J Trop Med Hyg. 2022 Feb 28;106(4):1227–1236.
- Vaughan AM, Kappe SHI. Genetically attenuated malaria parasites as vaccines. Expert Rev Vaccines. 2017 Aug;16(8):765–767. doi: 10.1080/14760584.2017.1341835
- Müller K, Gibbins MP, Roberts M, et al. Low immunogenicity of malaria pre-erythrocytic stages can be overcome by vaccination. EMBO Mol Med. 2021 Apr 9;13(4):e13390.
- Gardner MJ, Hall N, Fung E, et al. Genome sequence of the human malaria parasite Plasmodium falciparum. Nature. 2002 Oct 3;419(6906):498–511.
- Müller DA, Charlwood JD, Felger I, et al. Prospective risk of morbidity in relation to multiplicity of infection with Plasmodium falciparum in São Tomé. Acta Trop. 2001 Feb 23;78(2):155–162.
- Touray AO, Mobegi VA, Wamunyokoli F, et al. Diversity and multiplicity of P. falciparum infections among asymptomatic school children in Mbita, western Kenya. Sci Rep. 2020 Apr 3;10(1):5924.
- Gandhi K, Thera MA, Coulibaly D, et al. Next generation sequencing to detect variation in the Plasmodium falciparum circumsporozoite protein. Am J Trop Med Hyg. 2012 May;86(5):775–781. doi: 10.4269/ajtmh.2012.11-0478
- Dzikowski R, Templeton TJ, Deitsch K. Variant antigen gene expression in malaria. Cell Microbiol. 2006 Sep;8(9):1371–1381. doi: 10.1111/j.1462-5822.2006.00760.x
- Ouattara A, Barry AE, Dutta S, et al. Designing malaria vaccines to circumvent antigen variability. Vaccine. 2015 Dec 22;33(52):7506–7512.
- Chattopadhyay R, Pratt D. Role of controlled human malaria infection (CHMI) in malaria vaccine development: A U.S. food & drug administration (FDA) perspective. Vaccine. 2017 May 15;35(21):2767–2769.
- Walk J, Reuling IJ, Behet MC, et al. Modest heterologous protection after Plasmodium falciparum sporozoite immunization: a double-blind randomized controlled clinical trial [journal article]. BMC Med. 2017 Sep 13;15(1):168.
- USP. Cryopreservation of Cells <1044>. In: USP-NF. Rockville, MD: USP; Sep 27, 2018. Available at https://www.usp.org/sites/default/files/usp/document/our-work/biologics/resources/gc-1044-cryopreservation-of-cells.pdf
- Jusu MO, Glauser G, Seward JF, et al. Rapid establishment of a cold chain capacity of -60°C or colder for the STRIVE Ebola vaccine trial during the Ebola outbreak in Sierra Leone. J Infect Dis. 2018 May 18;217(suppl_1):S48–s55.
- Di Giulio G, Lynen G, Morzaria S, et al. Live immunization against East Coast fever–current status. Trends Parasitol. 2009 Feb;25(2):85–92. doi: 10.1016/j.pt.2008.11.007
- Matthias DM, Robertson J, Garrison MM, et al. Freezing temperatures in the vaccine cold chain: a systematic literature review. Vaccine. 2007 May 16;25(20):3980–3986.
- Comes M, Bergtora Sandvik K, van de Walle BA. Cold chains, interrupted: the use of technology and information for decisions that keep humanitarian vaccines cool. J Humanit Logist Supply Chain Manag. 2018;8(1):49–69. doi: 10.1108/JHLSCM-03-2017-0006
- Pambudi NA, Sarifudin A, Gandidi IM, et al. Vaccine cold chain management and cold storage technology to address the challenges of vaccination programs. Energy Rep. 2022 [2022 Nov 1];8:955–972.
- Richie TL. Interactions between malaria parasites infecting the same vertebrate host. Parasitology. 1988;96(3):607–639. doi: 10.1017/S0031182000080227
- Nussenzweig RS, Vanderberg JP, Spitalny GL, et al. Sporozoite-induced immunity in mammalian malaria. A review. Am J Trop Med Hyg. 1972;21(5_Suppl):722–728. doi: 10.4269/ajtmh.1972.21.722
- Nussenzweig RS, Chen D. The antibody response to sporozoites of simian and human malaria parasites: its stage and species specificity and strain cross-reactivity. Bull World Health Organ. 1974;50(3–4):293–297.
- Sina BJ, Do Rosario VE, Woollett G, et al. Plasmodium falciparum sporozoite immunization protects against Plasmodium berghei sporozoite infection. Exp Parasitol. 1993;77(2):129–135. doi: 10.1006/expr.1993.1069
- Sedegah M, Weiss WW, Hoffman SL. Cross-protection between attenuated Plasmodium berghei and P. yoelii sporozoites. Parasite Immunol. 2007 Nov;29(11):559–565. doi: 10.1111/j.1365-3024.2007.00976.x
- Inoue M, Tang J, Miyakoda M, et al. The species specificity of immunity generated by live whole organism immunisation with erythrocytic and pre-erythrocytic stages of rodent malaria parasites and implications for vaccine development. Int J Parasitol. 2012 Aug;42(9):859–870. doi: 10.1016/j.ijpara.2012.07.001
- Mendes AM, Machado M, Goncalves-Rosa N, et al. A Plasmodium berghei sporozoite-based vaccination platform against human malaria. NPJ Vaccines. 2018;3(1):33. doi: 10.1038/s41541-018-0068-2
- Reuling IJ, Mendes AM, de Jong GM, et al. An open-label phase 1/2a trial of a genetically modified rodent malaria parasite for immunization against Plasmodium falciparum malaria. Sci Transl Med. 2020 May 20;12(544). doi: 10.1126/scitranslmed.aay2578
- Arevalo-Herrera M, Vasquez-Jimenez JM, Lopez-Perez M, et al. Protective efficacy of Plasmodium vivax radiation-attenuated sporozoites in Colombian volunteers: a randomized controlled trial. PLoS Negl Trop Dis. 2016 Oct;10(10):e0005070. doi: 10.1371/journal.pntd.0005070
- White NJ. Determinants of relapse periodicity in Plasmodium vivax malaria. Malar J. 2011 Oct 11;10(1):297.
- Schafer C, Zanghi G, Vaughan AM, et al. Plasmodium vivax latent liver stage infection and relapse: Biological insights and new experimental tools. Annu Rev Microbiol. 2021 Oct 8;75(1):87–106.
- Schafer C, Dambrauskas N, Reynolds LM, et al. Partial protection against P. vivax infection diminishes hypnozoite burden and blood-stage relapses. Cell Host Microbe. 2021 May 12;29(5):752–756 e4.
- Betuela I, Rosanas-Urgell A, Kiniboro B, et al. Relapses contribute significantly to the risk of Plasmodium vivax infection and disease in Papua New Guinean children 1-5 years of age. J Infect Dis. 2012 Dec 1;206(11):1771–1780.
- Robinson LJ, Wampfler R, Betuela I, et al. Strategies for understanding and reducing the Plasmodium vivax and Plasmodium ovale hypnozoite reservoir in Papua New Guinean children: a randomised placebo-controlled trial and mathematical model. PLOS Med. 2015 Oct;12(10):e1001891. doi: 10.1371/journal.pmed.1001891
- Moore KA, Simpson JA, Scoullar MJL, et al. Quantification of the association between malaria in pregnancy and stillbirth: a systematic review and meta-analysis. Lancet Glob Health. 2017 Nov;5(11):e1101–e1112. doi: 10.1016/S2214-109X(17)30340-6
- World Health Organization. World malaria report 2022. Geneva: World Health Organization; 2022.
- Bundy DAP, Silva N, Horton S, et al. Child and adolescent health and development: Realizing neglected potential. Child and Adolescent Health and Development. 3rd edition. The International Bank for Reconstruction and Development/The World Bank; 2017p. 1–24. doi: 10.1596/978-1-4648-0423-6_ch1
- Brooker SJ, Clarke S, Fernando D, et al. Malaria in middle childhood and adolescence. Disease control priorities. Third Vol. 8. Child and Adolescent Health and Development; 2017p. 183–198. doi: 10.1596/978-1-4648-0423-6_ch14
- World Health Organization. Seasonal malaria chemoprevention with sulfadoxine–pyrimethamine plus amodiaquine in children: a field guide. Geneva: World Health Organization; 2023.
- Chandramohan D, Zongo I, Sagara I, et al. Seasonal malaria vaccination with or without seasonal malaria chemoprevention. N Engl J Med. 2021 Aug 25;385(11):1005–1017.
- Takarinda KP, Nyadundu S, Govha E, et al. Factors associated with a malaria outbreak at Tongogara refugee camp in Chipinge district, Zimbabwe, 2021: a case-control study. Malar J. 2022 Mar 19;21(1):94.
- World Health Organization. Malaria vaccines: preferred product characteristics and clinical development considerations. 2022.
- Dabira ED, Hachizovu S, Conteh B, et al. Efficacy, safety and tolerability of pyronaridine-artesunate in asymptomatic malaria-infected individuals: a randomized controlled trial. Clin Infect Dis. 2022 Jan 29;74(2):180–188.
- Duffy PE. Transmission-blocking vaccines: harnessing herd immunity for malaria elimination. Expert Rev Vaccines. 2021 Feb;20(2):185–198. doi: 10.1080/14760584.2021.1878028
- World Health Organization. Zeroing in on malaria elimination: final report of the E-2020 initiative. 2021.
- World Health Organaization. (2021, Oct 6). WHO Recommends Groundbreaking Malaria Vaccine For Children At Risk [News release]. Retrieved from https://www.who.int/news/item/06-10-2021-who-recommends-groundbreaking-malaria-vaccine-for-children-at-risk
- Datoo MS, Natama HM, Somé A, et al. Efficacy and immunogenicity of R21/Matrix-M vaccine against clinical malaria after 2 years’ follow-up in children in Burkina Faso: a phase 1/2b randomised controlled trial. Lancet Infect Dis. 2022 Dec;22(12):1728–1736. doi: 10.1016/S1473-3099(22)00442-X
- RTS,S Clinical Trials Partnership. Efficacy and safety of RTS,S/AS01 malaria vaccine with or without a booster dose in infants and children in Africa: final results of a phase 3, individually randomised, controlled trial. Lancet. 2015 Apr 23;386(9988): 31–45. doi: 10.1016/S0140-6736(15)60721-8
- Plowe CV, Alonso P, Hoffman SL. The potential role of vaccines in the elimination of falciparum malaria and the eventual eradication of malaria. J Infect Dis. 2009;200(11):1646–1649. doi: 10.1086/646613