ABSTRACT
Introduction
Liposomes have been used as carriers for vaccine adjuvants and antigens due to their inherent biocompatibility and versatility as delivery vehicles. Two vial admixture of protein antigens with liposome-formulated immunostimulatory adjuvants has become a broadly used clinical vaccine preparation approach. Compared to freely soluble antigens, liposome-associated forms can enhance antigen delivery to antigen-presenting cells and co-deliver antigens with adjuvants, leading to improved vaccine efficacy.
Areas covered
Several antigen-capture strategies for liposomal vaccines have been developed for proteins, peptides, and nucleic acids. Specific antigen delivery methodologies are discussed, including electrostatic adsorption, encapsulation inside the liposome aqueous core, and covalent and non-covalent antigen capture.
Expert opinion
Several commercial vaccines include active lipid components, highlighting an increasingly prominent role of liposomes and lipid nanoparticles in vaccine development. Utilizing liposomes to associate antigens offers potential advantages, including antigen and adjuvant dose-sparing, co-delivery of antigen and adjuvant to immune cells, and enhanced immunogenicity. Antigen capture by liposomes has demonstrated feasibility in clinical testing. New antigen-capture techniques have been developed and appear to be of interest for vaccine development.
1. Background
1.1. Liposomes
Liposomes are nanoscale spherical particles comprising a lipid bilayer that enclose an internal aqueous volume. They can be categorized according to particle size, lamellarity, surface charge, and composition. Liposomes are often formed from natural and biodegradable lipids and as such are generally considered nontoxic for clinical use [Citation1]. Lipids commonly used in liposome production are readily available in pharmaceutical-grade format at a reasonable cost. Liposome characteristics can be modified by altering their lipid composition or by changing production methodology. Disadvantages of the liposomal delivery systems include logistical challenges of production scale-up, batch-to-batch variability, the possibility of settling, aggregation, or degradation during storage, and limited loading capacity for antigens and adjuvants. In general, the use of a liposomal formulation can complicate the characterization a vaccine. For both simplicity of analytical quantification and for long-term storage, storing the antigens and liposomes separately is a common approach.
The size of liposomes can impact the immune response induced. In liposomal vaccine studies for leishmaniasis and for malaria, researchers found that smaller size liposome (around 100 nm) tended to activate Th2 cell-biased immune response, while larger size liposomal vaccines (400 nm or larger) induced more Th1 cell-biased cytokines and IFN-γ production [Citation2,Citation3]. The multilamellar structure of liposomes could increase liposome size, and researchers found that the increased size and multilamellar structure slightly increased liposomal vaccine immunogenicity when given orally [Citation4]. According to the desired immune response to be activated, liposome size could be tailored during the manufacturing process. However, since most vaccines are intended for parenteral usage, compatibility with 200 nm sterile filtration processes may complicate the use of large liposomes greater than that size.
Liposomes encapsulating the recombinant malaria circumsporozoite protein were tested as a human vaccine over 30 years ago, in 1992 [Citation5]. Three years later, PEGylated liposomal doxorubicin (DOXIL), the first approved liposomal product, was approved by the United States Food and Drug Administration (FDA) to treat Kaposi’s sarcoma as a cancer chemotherapy agent [Citation6]. Since then, liposomal formulations of numerous small-molecule active pharmaceutical ingredients, including cancer cytotoxic agents, antifungals, and pain relievers, have gained regulatory approvals [Citation7]. Monophosphoryl lipid A (MPLA) and aluminum phosphate-containing prophylactic hepatitis B infection vaccine (Fendrix) was approved in the European Union in 2005. In 2009, Cervarix which is adjuvanted with Adjuvant System 04 (AS04), containing MPLA adsorbed to aluminum hydroxide (Alum), was approved by the FDA as a prophylactic vaccine for cervical cancer [Citation8,Citation9]. In 2017, the FDA approved the recombinant zoster vaccine Shingrix, which includes the liposomal adjuvant system 01 (AS01). Shingrix provides long-term protection with low toxicity and is superior to Zostavax, an attenuated varicella-zoster virus vaccine (no longer available in the United States) [Citation10]. Most recently, AS01-adjuvanted Respiratory Syncytial Virus (RSV) pre-fusion F antigen vaccine, termed Arexvy, has gained approval in the United States for an RSV vaccine for the elderly. From a vaccine perspective, liposomes have been used to carry both antigens to induce antigen-specific immune responses and immunostimulatory adjuvants to enhance the immune response [Citation11]. While the association between liposomes and antigens is often not considered, various approaches exist to associate antigens with liposomes. This review focuses on this role and the design of liposomes as antigen carriers.
illustrates how antigen-associated immunogenic liposomes can exert several useful traits for vaccines. Firstly, liposomes provide a convenient delivery system for solubilizing immunostimulatory adjuvants, many of which cannot easily be directly dissolved in aqueous solution. Secondly, after liposomes are injected intramuscularly (IM), they form a depot at the injection site and slowly drain into the draining lymph node (dLN). Thirdly, antigen-presenting cells (APCs), which have long been known to avidly take up liposomes [Citation12], also take up liposome-associated antigens, leading to improved antigen uptake and presentation. Fourthly, by co-delivering adjuvants (together with antigens), APCs can upregulate expression co-stimulatory molecules (such as CD40 and CD28), which lead to improved engagement with T cells, which engage with processed antigen fragments displayed on the APC surface via a major histocompatibility complex (MHC) [Citation13,Citation14]. APCs present antigens to CD4+ T cells via MHC II complex and to CD8+ T cells via MHC I complex. Co-stimulation for B cells or T cells achieved through the CD40-CD40L pathway, and the CD80/86-CD28 pathway are important for effective immune responses [Citation15–17]. Fifthly, high density of antigens displayed on liposomes can also directly engage antigen with B cells bearing the appropriate B cell receptors (BCR), and induce BCR crosslinking, leading to improved immune response [Citation18]. Thus, taken together, there are multiple routes and mechanisms by which the use of liposome-associated antigens can enhance immunogenicity.
Figure 1. Roles and mechanisms for antigen delivery by immunogenic liposomes following intramuscular injection. liposomes can directly solubilize various adjuvants. Liposome-associated antigens are injected into the muscle and form a depot at the injection site. Antigen-presenting cells (APCs) uptake liposomes and present antigens on the APC surface via major histocompatibility complex (MHC) molecules. Co-delivered adjuvant helps to promote the co-stimulating of T cells. Followed by activated CD4+ and CD8+ T cells to initiate an effective immune response. High-density antigens also lead to B cell receptor crosslinking, followed by co-stimulation between CD4 T cells and B cells, promoting B cell expansion and differentiation.
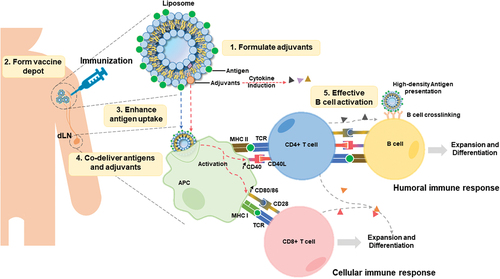
1.2. Vaccine adjuvant formulation using liposomes
Liposomes are an appealing choice for adjuvant formulation, given their biocompatibility and have a strong track record of use in humans for delivering vaccine adjuvants and other active pharmaceutical ingredients. Some representative adjuvants that can be formulated with liposomes bilayer are shown in .
Figure 2. Chemical structure of vaccine adjuvants that localize in lipid bilayers. Representative structures for QS21, MPLA, αGalCer, and 3M–052 are shown.
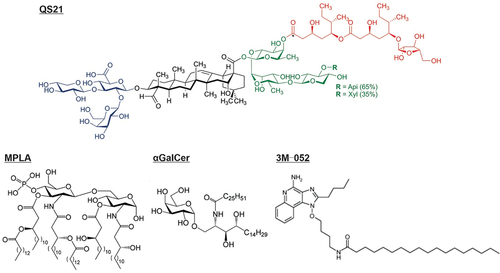
Saponin QS21 is a saponin extracted from the Chilean Soapbark tree (Quillaja saponaria). It has strong immune-stimulating efficacy with relatively low toxicity compared to other Quillaja saponin extract fractions [Citation19]. It is an important component of several adjuvant systems like AS01. Some of the toxicity of QS21 is ascribed to its interaction with cell membrane cholesterol that results in hemolytic activity and cell membrane permeabilization [Citation20]. Importantly, combining cholesterol with QS21 in liposome formulations can neutralize QS21 hemolytic activity [Citation21]. Since cholesterol is widely used in liposome formulations to provide a rigid and stable bilayer structure, liposomes represent a natural carrier for formulating QS21. As an immune response stimulator, QS21 formulated vaccines stimulate T helper type 1 (Th1) and T helper type 2 (Th2) cell-related immune responses [Citation22]. In a malaria liposomal vaccine study, QS21 enhanced vaccine activity by increasing the production of antigen-specific IFN-γ, activating CD4+ T cells, and elevating IgG2c levels, reflecting a Th1-biased immune response activation [Citation23]. In cancer vaccine studies, QS21-containing liposomal vaccines induced more antigen-specific CD8+ T cells and memory T cells than a liposomal vaccine without QS21 [Citation24].
Monophosphoryl lipid A (MPLA) is a modified lipid originating from the lipid A component of lipopolysaccharide (LPS). MPLA has diminished pyrogenicity (fever-inducing character) and, besides QS21, is the other immune-active component in the AS01 liposomal adjuvant. The army liposome formulation (ALF), and cationic adjuvant formulation (CAF) CAF09b also contain MPLA. There are multiple variants of MPLA, including numerous synthetic forms including PHAD [Citation25]. MPLA is widely used for liposomal vaccine development and activates the toll-like receptor (TLR) TLR4 signaling pathway at a safe inflammatory toxicity level [Citation26]. After MPLA targets the TLR4 receptor, the nuclear factor-kappaB (NF-κB) transcription factor is engaged and activates the TRAM-TRIF signaling pathway to induce Th1 cells [Citation27]. MPLA-formulated liposomes can activate the TLR4 signaling via the TRIF-dependent pathway to induce clathrin-mediated endocytosis by macrophages [Citation28]. ALF contains saturated phospholipids, cholesterol, and synthetic monophosphoryl 3-deacyl lipid A (3D-PHAD), which proved safe for enhancing vaccine immunogenicity in clinical trials [Citation29]. There are several formulations of ALF, such as ALF containing QS21 (ALFQ) and the ALF absorbed by aluminum hydroxide (ALFA). A malaria vaccine development study based on recombinant circumsporozoite protein (CSP) FMP013 antigen compared the adjuvanticity of ALF, ALA, and ALFQ formulations [Citation23]. ALFQ-immunized mice had the highest survival against a transgenic P. berghei sporozoite challenge with increased anti-antigen antibodies, Th1-biased IgG2c titer elevation, CSP-specific antibody-secreting cells, and antigen-specific IFN-γ production. The QS21 in the ALFQ formulation was also crucial for activating monocyte‑derived macrophages (MDM) [Citation30].
Unmethylated CpG oligodeoxynucleotides (CpG-ODN) sequences mimic bacterial DNA, which can stimulate immune response via activating TLR9 and activate T-helper cell-mediated immune response [Citation31]. A study to induce tissue-resident memory T cells (TRM cells) found the CpG-ODN to be the strong liver TRM cells inducer. Furthermore, it enhanced adjuvanticity when formulated in 1,2-dioleoyl-3-trimethylammonium-propane (DOTAP) cationic liposome [Citation32]. Another study reported that encapsulating CpG-ODN and protein antigens in liposomes was superior to soluble CpG-ODN mixed with protein-encapsulated liposomes in inducing IgG2a antibodies [Citation33]. In 2017, the 22-mer modified synthetic CpG-ODN, CpG 1018 adjuvanted prophylactic recombinant Hepatitis B Vaccine (HEPLISAV-B) was approved by FDA.
Resiquimod (R848) and telratolimod (3 M–052) are both dual agonists for TLR7 and TLR8. In tumor-related studies, TLR7/8 agonists reeducated M2 tumor-associated macrophages (TAMs) to the M1 phase TAMs to inhibit tumor growth [Citation34]. Loading R848 into nanoparticles can enhance the uptake of nanoparticles to TAMs. Liposomal R848 treated bone-marrow-derived macrophages (M0) showed a similar gene expression profile and morphology change to M1 type macrophages. Liposomal R848 accumulated in tumors and was uptake by TAMs [Citation35]. The synthetic imidazoquinolinone 3 M–052 is structurally similar to R848 but has a hydrophobic tail and is reported to induce higher antigen-specific IgG antibodies [Citation36]. A 3 M–052 formulated liposomal vaccine (GLA-3 M-052-PEG2000) admixed with recombinant antigen LecA enhanced antigen-specific IgA and IgG titer, increase intracellular IFN-γ levels and protect mice from the infection of intestinal [Citation37]. Co-delivery of TLR4 and TLR7/8 agonists increased production of IgG2a antibodies (Th1-related immune response) and the IL-12p70 cytokine [Citation38].
The glycolipid alpha-galactosylceramide (αGalCer) activates invariant natural killer (NK) T (iNKT) cells and proinflammatory cytokine production, which benefits immune responses against cancer, infection, and some auto-immune diseases [Citation39]. αGalCer can be used to bind proteins to form lipoprotein and be inserted into the lipid bilayer for antigen display on liposome [Citation40]. αGalCer as an effective adjuvant does not require covalently binding with antigen [Citation41]. 7DW8-5 is a derivative of αGalCer, reported to be a more potent activator for iNKT cells via binding to the CD1d protein [Citation42,Citation43].
The most used commonly adjuvant for vaccine development has been aluminum salts, and these have also been explored in combination with lipids and liposomes. The FDA has approved aluminum salt adjuvants for decades including aluminum hydroxide, aluminum phosphate, potassium aluminum sulfate, or mixed aluminum salts. Aluminum salts form a depot effect for prolonged release of admixed antigen and induce Th2-related immune response. Aluminum salt adjuvants are frequently compared with novel vaccine adjuvants as a baseline to test whether the testing adjuvant can effectively enhance immune response [Citation44,Citation45]. MPLA adsorbed to aluminum salts has been used for vaccine development studies including HIV, fentanyl, mite allergen, and malaria vaccine research [Citation46–50]. A clinical trial compared the antigen-alum and antigen-encapsulated MPLA-containing liposomes adsorbed by alum [Citation51]. Results showed that the liposome-formulated vaccine provided long-lasting protection and functional antibodies. Some studies compared the immunogenicity of antigen-adsorbed aluminum hydroxide (antigen-AH) with or without additional Army Liposome Formulation (ALF) adjuvant [Citation52]. Co-delivery of antigen-AH and liposomes increased the antigen-specific antibody titer and the percentage of the IgG2a antibody. Adding QS21 to the ALF formulation (ALFQ) and then adsorbing this to aluminum hydroxide (ALFQA) further improved the IgG antibody titer. Aluminum hydroxide is a Th2-biased adjuvant, while the MPLA and QS21 bring a more balanced Th1/Th2 immune response. The ALF adjuvant absorbed by aluminum hydroxide was used to investigate whether the pre-exposure to carrier protein would decrease the immunogenicity vaccine (TT-6-AmHap) [Citation53]. This vaccine utilized the opioid hapten (6-AmHap) conjugated with carrier tetanus toxoid (TT) protein and found that pre-exposure TT did not affect the induction of anti-6-AmHap antibodies.
2. Mixtures of antigens and liposomes
Admixture is a simple method for preparing liposomal vaccines on site before use. It often involves separately manufactured and vialed antigens and liposomes. Although this approach is complex, it simplifies the manufacturing, characterization, and access of each respective vial. Many vaccines using a two-vial approach with admixture comprise a vial of liquid liposomes and a vial of a lyophilized protein antigen, which also reduces protein degradation during storage. Admixture can be applied to antigens and liposomes that depends on electrostatic reactions or requires no association. Series of liposomes based on cationic formulated adjuvant (CAF) or Adjuvant system 01 (AS01) can use admixture method for vaccine preparation. Alternatively, liposomes and antigens can be pre-mixed prior to injection, and delivered in a single vial. This approach may be more convenient for vaccine distribution, however complexities could arise in vaccine characterization. Other complex lipid particles, such as bacterial membranes, virosomes, and virus-like lipid particles for the antigen delivery have been developed, but are not discussed in this review.
2.1. Electrostatic-based Mixtures
Liposomes can be neutral, cationic, and anionic based on the charges of the components. Through electrostatic reaction, charged liposomes can carry various components for antigen delivery and immune response stimulating purposes. Cationic liposomes formulated with 1,2-dioleoyl-sn-glycero-3-ethylphosphocholine (DOEPC) and the hydrophobic 3 M–052 adjuvant can adsorb the negatively charged recombinant glycoprotein B (gB) antigen [Citation54]. The rise of anti-gB IgG2C antibodies supported the idea that the 3 M–052 TLR7/8 agonist increases the Th1/Th2 T cell response ratio, enhances cytotoxic CD8+ T cell response, and downregulates T regulator cell, which suppresses the immune response [Citation55].
With respect to cellular interactions, cationic liposomes tend to become adsorbed on the negatively charged surfaces of cells, allowing rapid APC uptake of antigens [Citation56]. One caveat is that it can be difficult to distinguish antigen uptake into cells with antigen adsorption, since analysis techniques, such as flow cytometry cannot distinguish these [Citation57]. Since positively charged liposomes may also bind to normal cells instead of APCs, researchers assessed liposomal surface charge using intradermal injection. Dermal dendritic cells preferred anionic and neutral liposomes [Citation57]. It is possible to attach the bacterial surface layer protein (SLP) to cationic liposomes through an electrostatic reaction with carboxyl groups of SLP () [Citation58]. The SLP-decorated liposome can protect the enclosed antigen from stomach acid and survive different pH conditions, which makes it a good choice for oral vaccine development [Citation60]. One study used cationic liposome to adsorb negatively charged conjugate of the Group B Streptococcus (GBS) GBS67 protein and CpG-ODN, which was conjugated through a thiol-maleimide reaction. The liposome adsorbed the conjugate on surface, resulting in decreased CpG-ODN toxicity while preserving the intact antigen immunogenicity and adjuvanticity of CpG-ODN [Citation61]. Compared with non-conjugated mixture of CpG-ODN adjuvant, GBS67 protein-adsorbed cationic liposomes, the conjugate-adsorbed liposome increased both Th1 and Th2-related antibodies titer with no bias, elevated cytokines concentration, including IL-17A, IL-17F, IL-21, and IL-22. Furthermore, cationic liposomes formed a depot at the injection site, contributing to prolonged antigen retention for sustained immune system stimulation. It was difficult to conclude whether cationic liposomes were superior to anionic or neutral ones in delivering antigens and inducing immune responses. For example, when Ovalbumin (OVA) was admixed with cationic (1,2-Dioleoyl-3-trimethylammonium propane, DOTAP), anionic (3,4-dihydroxyphenylalanine, DOPA), or neutral (1,2-Dioleoyl-sn-glycero-3-phosphocholine, DOPC) liposomes, cationic liposomes showed increased particle size. Both cationic and anionic liposomes induced strong anti-OVA IgG1 responses without statistical difference [Citation62]. Another study reported that only anionic ID93 liposomes made of 1,2-Dipalmitoyl-sn-glycero-3-phosphocholine (DPPC) and 1,2-Dipalmitoyl-sn-glycero-3-phosphoglycerol (DPPG) induced a significantly higher number of Th1 cells in the presence of the MPLA-variant glucopyranosyl lipid, in contrast to cationic liposomes [Citation63]. When incorporating charge in liposomal vaccines, the formulation of the lipid, the type of antigen, and the route of administration should be considered.
Figure 3. Charged liposomes can capture proteins and mRNA through electrostatic reactions. a) SLP protein spontaneously loads on the cationic liposome surface through electrostatic force [Citation58]. b) mRNA electrostatically adsorbed on lipid-coated PBAE liposome [Citation59]. With permission from International journal of Nanomedicine 2019, originally published by and used with permission from Dove Medical Press Ltd, and American Chemical Society, copyright 2011.
![Figure 3. Charged liposomes can capture proteins and mRNA through electrostatic reactions. a) SLP protein spontaneously loads on the cationic liposome surface through electrostatic force [Citation58]. b) mRNA electrostatically adsorbed on lipid-coated PBAE liposome [Citation59]. With permission from International journal of Nanomedicine 2019, originally published by and used with permission from Dove Medical Press Ltd, and American Chemical Society, copyright 2011.](/cms/asset/960ccafc-e888-4532-8fe7-c1d2971e3fb5/ierv_a_2274479_f0003_oc.jpg)
2.1.1. Cationic adjuvant formulation (CAF)
The Cationic Adjuvant Formulation (CAF) includes the positively charged lipid dimethyl dioctadecylammonium (DDA) and is a liposomal adjuvant system that facilitates the co-delivery of antigens and immune stimulators to target immune cells. The first liposomal CAF adjuvant, CAF01, also contains the immunostimulatory glycolipid trehalose-6,6-dibehenate (TDB), which is a Mincle agonist reported to improve recognition and activation M1-phase macrophage [Citation64,Citation65]. The liposomal CAF04 adjuvant contains the immunostimulatory mycobacterial cell-wall lipid monomycoloyl glycerol (MMG), which is a potential immunostimulant and reported to induce an active Th1 immune response and induce the CD4+ T cell-mediated immune response [Citation66–68]. Based on the CAF04 formulation, the CAF09 adjuvant includes the TLR4 agonist MPLA to enhance immune response. CAF01 and CAF09 are two CAF-based liposomal adjuvants in human clinical trials.
CAF01 has been applied in clinical studies for a variety of diseases that require a strong and long-lasting immune response, including tuberculosis (NCT00922363), chlamydia (NCT02787109, NCT03926728), HIV (NCT01009762, NCT01141205), and malaria (PACTR201503001038304). A phase I clinical trial showed that CFA01 adjuvanted tuberculosis vaccines are safe, well tolerated, and able to induce the production of Th1-related cytokines, such as IFN-γ and TNF-α [Citation69]. Phase I clinical trials of a chlamydia vaccine consisting of the adjuvant CAF01 and recombinant subunit protein antigen CTH522 showed safety with increased IgG and neutralizing antibody titer and IFN-γ production after IM immunizations [Citation70].
The CAF09 formulation is an adjuvant nanoparticle synthesized with TLR3 agonist poly(I:C), DDA, MMG, and MLPA lipid. It has been advanced for cancer vaccines (NCT03412786), chlamydia vaccine (NCT03926728), malaria vaccine, HCV, and HIV [Citation71]. CAF09b adjuvanted vaccines can help cross-present exogenous antigens to MHC I molecule on APCs and induce robust CD4+ and CD8+ T cell response [Citation66,Citation72,Citation73]. For example, a pulmonary alveolar proteinosis (PAP) protein-derived MutPAP42mer peptide was admixed with CAF09b liposome as the vaccine for prostate cancer. CAF09 adjuvanted vaccine induced more PAP-specific CD8+ T cells, CD8 memory T cells, and higher cytotoxicity against PAP-expressing cells compared with MutPAP42mer peptide adjuvanted with CpG [Citation74].
The CAF08 liposomal formulation includes DDA, the 3 M–052 TLR7/8 agonist, and the Mincle agonist TDB [Citation75]. A single dose of CAF08 liposomes mixed with respiratory syncytial virus (RSV) perfusion antigen (RSV pre-F) vaccine protected newborn mice from infection. The co-stimulation to TLR7/8 receptors and Mincle increased the preference of Th1-related immune response, enhanced the RSV-specific CD8+ T cell response.
2.1.2. mRNA vaccines based on cationic lipids
Nucleic acid vaccines have been broadly explored, and often use cationic lipids for complexation [Citation76–78]. mRNA vaccines for COVID-19 brought this technology into the forefront of vaccine technology and has created great excitement about future mRNA vaccines [Citation79]. Generally, these vaccines use ionizable cationic lipids to form lipid nanoparticles (LNPs) [Citation80,Citation81]. The high immunogenicity and rapid degradation caused by mRNA limited vaccine application in the past. The entrapped mRNA is stable and immunogenic in LNPs, such as the Moderna (mRNA1273) and Pfizer (BNT162b1 and BNT162b2) vaccines. These entrapped mRNA remained stable during 2°C–8°C up to 30 days and proved to induce strong human antibody production and T cell response and keep safety in clinical trials [Citation82–85]. Other approaches for formulating negatively charged messenger RNA (mRNA) have been demonstrated such as protecting it by electrostatic adsorption on a cationic liposome surface, which contains a pH-sensitive poly(β-amino-ester) core that promotes liposome uptake by cells () [Citation59].
2.2. Adjuvant system 01 (AS01)
AS01 is a liposomal-based vaccine adjuvant system composed of cholesterol, unsaturated phospholipid, MPLA, and QS21. The AS01 system can activate the Toll-like receptor four pathway via MPLA and induce antigen-specific T cell responses [Citation86]. AS01-based vaccines can be prepared by admixing AS01 liposomes with lyophilized antigens. There are multiple approved vaccines based on this approach, including the Shingrix vaccine for shingles, the Mosquirix vaccine for malaria, and more recently, the Arexvy vaccine for RSV (NCT05059301, NCT04732871).
Shingrix is a recombinant Varicella Zoster virus vaccine that provides long-term protection for more than 90% of people from shingles and post-herpetic neuralgia (PHN) over 7 years after immunization [Citation55,Citation87]. The Shingrix vaccine comprises the adult formulation of AS01 (AS01B) liposome and varicella-zoster virus (VZV) glycoprotein E (gE), formed by two-vial admixing. Volunteers who received Shingrix showed a fast response rate and high geometric mean concentration (GMC) of anti-gE antibodies [Citation88]. According to a safety study that followed up with participants for a mean of 3.5 years, 9% reported adverse events from the Shingrix group and 8.9% from a placebo group without significant differences [Citation55]. Overall, the Shingrix vaccine is safe and has long-term effects for preventing shingles and PHN.
The RTS,S/AS01 malaria vaccine, also known as Mosquirix, contains the engineered recombinant protein antigen RTS,S, that is composed of protein targets for malaria parasite neutralization, and surface antigen of hepatitis B virus (HBsAg) to induce protein particle formation. The RTS,S antigen induced multifunctional antibodies in humans that neutralize malaria parasites [Citation89]. The AS01 formulated RTS,S vaccine protects humans from controlled malaria infection [Citation90]. The pediatric formulation RTS,S/AS01E, against P. falciparum malaria is recommended and prequalified by World Health Organization (WHO) [Citation91,Citation92]. Studies of other malaria-related vaccines that include AS01 are also ongoing, such as RH5.1/AS01 recombinant protein vaccine, targeting blood-stage parasite infection, which can produce functional antibodies and inhibit malaria parasites growth and duplicate in vivo [Citation93,Citation94].
In clinical trials evaluating the hepatitis B virus surface antigen (HBsAg) vaccine, the AS01 adjuvant demonstrated significant superiority over aluminum salts and non-liposomal formulations, such as AS03 (an oil-in-water emulsion containing α-tocopherol) and AS04 (MPLA adsorbed on aluminum salt), in terms of inducing HBsAg-specific CD4 T cells and IgG antibodies [Citation95]. Additionally, the AS01 formulation consistently and robustly induced innate cell-related responses, interferon-related responses, and NK cell responses in whole blood samples [Citation96]. Furthermore, AS01-adjuvanted HBsAg vaccines were found to elicit a more durable immune response compared to HBsAg vaccines adjuvanted with aluminum salts [Citation97].
3. Assessing antigen capture by Liposomes
Liposomal-based vaccines utilize different strategies to associate with antigens, especially for antigens displayed on liposome surfaces. shows ways in which antigens can be specifically captured by liposomes, especially the surface displaying strategy. In the generalized liposomal adjuvant approaches mentioned above, protein antigens may not stably associate with liposomes. Therefore, qualitative or quantitative analytical techniques must be developed to testify to antigen association with liposomes. For example, (non-liposomal) alum-adjuvanted vaccine formulations can easily be assessed for protein adsorption by separating the large alum microparticles from soluble proteins through centrifugation followed by evaluating protein concentration in the supernatant. Similar approaches are also possible with liposomes.
Figure 4. Modes by which antigens can be captured by liposome, including four types of surface display. Three antigen delivery routes include the admixture without association, encapsulation in an aqueous volume, and displayed on the surface. Under the surface display category, there are four strategies: electrostatic reaction, His-tag-to-metal linkage, lipid headgroup conjugation, and lipid tail anchoring.
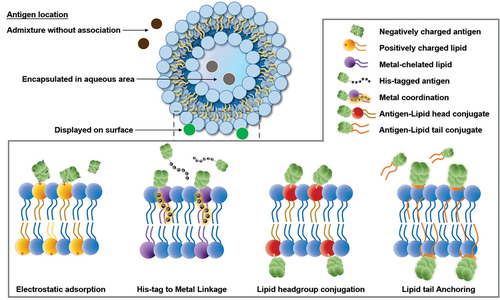
Free peptide or protein antigens in the supernatant can sometimes be separated from liposome-bound antigens in the pellet through centrifugation. Protein detection through standard assays can then quantify unbound antigen concentration in the soluble fraction [Citation98–100]. Antigens that cannot easily be quantified in that way can be labeled with fluorescent dyes to facilitate detection. For example, RNA can be stained with ribogreen dye or labeled with Cy3 dye to assess liposome loading efficiency after incubation and centrifugation to separate liposomes and free RNA [Citation54]. The fluorescence signal intensity of supernatants collected from RNA with or without co-incubation with liposomes is compared and calculated. A similar centrifugation approach used FITC-labeled lipopeptide pMPER to assess lipopeptide loading efficacy [Citation101]. The percentage of lipopeptide incorporated into liposome can also be calculated according to the absorbance of aromatic side chains at 280 nm [Citation102]. Calculating the antigen loading percentage by comparing supernatant and pellet is an alternative method. This is more complex because lipids in the liposome pellet fraction can necessitate a detergent addition or heating process to release the antigen from the liposome. For DNA entrapped within a liposome, DNase digests all unencapsulated DNA before extracting and precipitating encapsulated DNA from the liposome. The amount of loaded DNA by liposome can be quantified by subjecting the extraction product to electrophoresis [Citation103].
Depending on liposome size and composition, soluble unencapsulated antigen can be separated from liposome by centrifugation with centrifugation speeds as low as 12,000–15,000 g for 10 min [Citation48]. However, liposomes used in vaccines, such as AS01 tend be small and unilamellar with sub-200 nm diameter to both allow for sterile filtration and resist the propensity to settle during storage. In this case, their centrifugation can be challenging, depending on their composition and size. In some cases, ultracentrifugation at speeds of 150,000 g for extended periods has been used to separate free lipopeptide and liposome described above [Citation102]. Likewise, a similar high-speed centrifugation approach was used to assess positively charged antigens to anionic liposomes, followed by protein antigen quantification [Citation62].
Other techniques exist to assess antigen association. Fluorescently labeled antigens can be used in some cases if they change fluorescence properties upon binding to liposomes. For example, fluorescence resonance energy transfer (FRET) between the HiLyte 488-labeled antigen and the cobalt porphyrin-containing (CoPoP) liposome enables real-time monitoring of antigen capture by liposomes as well as assessing serum stability of the interaction [Citation104]. HIV-1 antigen CV2 lipopeptides were shown to be embedded in the liposome by visualizing Texas Red-labeled DHPE and green OG-conjugated palm-CV2 with fluorescence microscopy [Citation105].
According to liposome and antigen size, membrane filtration can separate liposome-loaded antigens and unbound soluble antigens [Citation106–108]. For example, the PepE-Bet v 1 protein coupling efficiency to CPK-liposomes can be determined by membrane filtration assay followed by quantification of PepE-Bet protein through RP-HPLC [Citation109]. The pepE-OVA323 association efficiency to CPK-liposome was determined by a filtration assay followed by RP-UPLC analysis [Citation110]. This membrane filtration method is unsuitable for some antigens exhibiting nonspecific binding to the filter membrane or antigens too large to pass through the membrane. In addition, lipopeptide aggregates cannot pass through the exclusion filter when not incorporated with liposome, which can be used to determine incorporation efficiency [Citation111]. Antigens bound with liposomes cannot enter the PAGE gel due to large particle size and causing no antigen bands to show on the gel. Compared to free antigen band intensity, antigen band intensity indicates antigen capture efficiency [Citation112].
Another approach to assess his-tagged protein binding to metal chelating liposomes involves using Nickel-NTA-coated beads. Exposing proteins and liposomes to these beads can separate free antigens and antigens associated with metal-chelated liposomes. The protein binds to metal-chelated liposomes and will no longer bind to the nickel beads. Both antigen concentrations in the supernatant or beads pellet can be measured by using sodium dodecyl sulfate – polyacrylamide gel electrophoresis (SDS-PAGE) [Citation113].
Circular dichroism (CD) is not a widely applicable method for accessing antigen association with liposome but has been used to observe secondary structure changes in peptides that occur upon liposome binding. The secondary structure changes indicate the association between antigen and liposomes. A lipopeptide consisting of palmitoylated β-amyloid peptide Aβ1–15 displayed a defined β-sheet conformation when incorporated onto the liposome surface, while the PEGylated Aβ1–16 showed a random secondary structure when incorporated into the liposome surface [Citation114]. The palmitoyl anchored palm-CV2 peptide, and palm-LV2 showed a mixture of α-helical and coil structure in 50% trifluoroethanol or 1% SDS condition but changed to exhibit completely random coil or completely β-sheet after incorporation onto ALF liposome separately [Citation105]. Antigens displayed on the surface of liposomes can be observed under negative staining electron microscopy (EM). This provides a direct method to visualize the density of antigens coupling on the liposomal surface [Citation115,Citation116]. To visualize smaller-size size antigens, gold-nanoparticle antibody labeling could be helpful [Citation117].
4. Entrapment of antigens within the liposome core
Liposomes can entrap hydrophilic molecules in their aqueous core. The passive entrapment of antigens during the lipid formation process does not require special modification of antigens, and it tends to be an ineffective method since only a small percent of the total antigens can be captured successfully inside the liposome. While various techniques, such as reverse-phase evaporation can greatly increase liposomal loading efficacy [Citation118], this also results in larger liposome diameters that are not suitable for sterile filtration and are prone to settling. Furthermore, following liposome formation, purification of the entrapped antigen should be separated from the external antigen. After purification, the antigen and liposome composition must be re-quantified, further complicating the approach. Despite these drawbacks, since no special conjugation linkage between antigen and liposome is required, this approach is frequently employed for vaccine studies.
Loading antigens inside liposomes can prevent rapid antigen degradation in the body, especially for nucleic acids or proteins prone to proteolytic degradation. As an effective prevention strategy against the SARS-CoV-2 virus, mRNA vaccines gained global attention as they can be developed and produced quickly and induce strong, robust humoral and cellular immune responses. A study investigating the RBD-mRNA encapsulated cationic liposomal (LPX/RBD-mRNA) vaccine against the SARS-CoV-2 virus showed that the injection route could impact immune response [Citation119]. The intramuscular way for mouse immunization showed decreased neutralizing antibodies compared with the intradermal route, while the intradermal route also shows a higher tendency to produce IgG2a antibodies rather than IgG1 antibodies.
This entrapment approach has also been extended to saRNA liposomal vaccine development [Citation120]. Self-amplifying RNA (saRNA) vaccines are a possible next-generation mRNA vaccine, which requires lower doses because saRNA encodes a viral enzyme for RNA replication. A study compared the encapsulation and surface electrostatic assembly loading strategies for saRNA liposomal vaccine [Citation121]. Cationic liposome protected saRNA from degradation. No difference between the delivery efficacy of encapsulated or surface displayed saRNA liposomal vaccines was observed. HIV-1 envelope glycoprotein gp140-included saRNA induced anti-HIV gp140 IgG antibody no matter which saRNA loading strategy was used. When the liposome surface is decorated for targeting or immune response enhancement purposes, encapsulating saRNA within liposomes emerges as the preferred choice to maintain unaffected saRNA loading efficacy. In a saRNA-encapsulated vaccine study, the surface display of mannose (achieved through the mannose interaction with cholesterol) demonstrated an increase in antigen-specific CD8 and CD4 T cell responses [Citation122]. However, it is important to note that in this study, a direct comparison between surface adsorption and encapsulation methods for saRNA loading efficacy was not conducted. Despite this, the encapsulation method holds promise as it ensures saRNA remains efficiently loaded, potentially enhancing the overall vaccine efficacy.
In 1988, a malaria vaccine study revealed that MPLA and aluminum hydroxide could boost the immunogenicity of the encapsulated cloned protein antigen (R32tet32), which was derived from the circumsporozoite (CS) protein of P. falciparum. In a rabbit study, the liposomes containing MPLA with encapsulated antigen R32tet32 outcompeted the soluble antigen, and the R32tet32-encapsulated liposome without MPLA in inducing dose-de Streptococcus pendent anti-R32tet32 IgG antibodies [Citation48]. Later, a clinical trial proved this antigen-encapsulated delivering strategy based on liposome is safe and can induce antigen-specific IgG titer for immunized humans to inhibit live sporozoite invasion [Citation5]. The adsorption of antigen and MPLA co-delivered liposome on aluminum hydroxide can further increase the anti-R32tet32 IgG antibodies in rabbit and rhesus monkey studies. For immune response induction, liposome surface display and liposome encapsulation were compared for the human MUC1 peptide antigen [Citation123]. The humoral immune response was more robust for the surface-displayed antigen, but the cellular immune response was similar for both strategies. This could be because antibody production is related to B cell interaction with liposome-displayed antigens, while the cellular immune response depends on antigen uptake and presentation on APCs. Another study found that the liposome-encapsulated antigen-induced immune response will not be affected by the liposome surface charge, but can be changed from a Th1-related immune response to a Th2-related immune response by incorporating immunostimulatory peptidoglycan monomer (PGM) [Citation124]. Entrapment of plasmid within liposome is an alternative strategy for inducing immune response for protein antigen that is difficult or unable to be produced by recombinant protein production. Such as in a malaria DNA vaccine study, encapsulated plasmids within liposomes provide a solution to induce functional antibodies [Citation103]. Plasmodium falciparum Rhoptry protein 5 (PfRH5) is a promising target for inducing immune responses against P. falciparum blood-stage infection. The small HBV antigen (HBsAg) was reported to enhance the immune response of some co-delivered antigens but failed to induce higher anti-HBs titer for many HBV vaccine developments. The PfRH5-HBsAg DNA-encoding plasmids encapsulated liposomal vaccine induced functional anti-PfRH5 antibody production though anti-HBsAg immune response remains low.
Liposomes displaying phosphatidylserine and encapsulating autoantigenic peptides can recover the immune system tolerance to self-antigens as a treatment for autoimmune disease. Such as encapsulating human insulin peptides can restore the tolerance to insulin-producing pancreatic β-cell for Type 1 diabetes [Citation98,Citation99]. Three liposome formulations were developed to encapsulate six melanoma helper peptides and TLR4 agonists [Citation125]. These six peptide-loaded liposomes were proven to be sustained at the secondary lymphoid organ for at least 6 days through subcutaneous injection, demonstrating that entrapping peptide in liposomes improved the in vivo retention. Protein antigens, such as ovalbumin (OVA) and derived peptides can be encapsulated into liposomes during the hydration process of liposome synthesis [Citation28]. By repeated freeze and thaw, OVA peptides were encapsulated by 1,2-Dioleoyl-sn-glycero-3-phosphoethanolamine (DOPE) structured liposome, which is then modified by lipid derivative of peptide KALA (STR-KALA) to form the liposomal vaccine KALA-OVA-LPs [Citation100]. Mice received a single injection of the KALA-OVA-LPs vaccine showed stronger CTL activity with retarded tumor growth in a prophylactic OVA-expressing tumor challenge. A study about encapsulating RBD protein antigen in DOPC liposome and covering liposome with immunostimulatory CpG to form a spherical nucleic acid (SNA) provided an efficient method for the co-delivery of antigen and adjuvant () [Citation126]. Compared with simply mixing antigen with adjuvants (Alum, AS01b, CpG DNA, AddaVax), the SNA vaccine induced 10 times higher functional antibodies titer than the admixed vaccine and protected immunized mice from the fatal viral infection.
Figure 5. Entrapment of antigens in the aqueous core of liposomes. a) RBD protein antigen encapsulated by DOPC liposome covered with CpG motif DNA [Citation126]. b) OVA protein encapsulated by GM3-αGC liposome [Citation127]. Used with permission from National Academy of Sciences, copyright 2022, and MDPI, copyright 2021.
![Figure 5. Entrapment of antigens in the aqueous core of liposomes. a) RBD protein antigen encapsulated by DOPC liposome covered with CpG motif DNA [Citation126]. b) OVA protein encapsulated by GM3-αGC liposome [Citation127]. Used with permission from National Academy of Sciences, copyright 2022, and MDPI, copyright 2021.](/cms/asset/1e3abbe7-63be-45e7-9688-5a68d7b138ff/ierv_a_2274479_f0005_oc.jpg)
Glycosphingolipid GM3 ganglioside is reported to target CD169-expressed cells such as CD169+ macrophages and pre-Dendritic cells, which proved to play an essential role in anti-bacteria, anti-virus, and anti-tumor immune response [Citation128]. Decorating liposomes with ganglioside, such as the GM3 liposomes, increased the encapsulated antigen uptake by human CD169+ APCs, induced antigen-specific CD8 T cells, and activated type I natural killer T cells [Citation129]. The WT1 and gP100 peptide antigen encapsulated in ganglioside-liposome all benefit from the improved targeting delivery to CD169+ APCs via cross-presentation of antigens to CD8+ T cells which resulted in 2 times higher production of IFN-γ than plain liposomal vaccine [Citation130]. Adding OVA protein antigen to lipid film during the hydration process, OVA was encapsulated within GM3-αGC-OVA liposome formulated with GM3 and αGalCer () [Citation127]. The GM3-containing liposomes induced a higher percentage of antigen-specific CD8 T cells in vivo. In addition, the GM3-αGC-OVA liposome targeted CD169+ macrophages, activated NK cells, caused the maturation of DCs, and increased the anti-tumor cytokine IL-12 release. In another study based on the GM3 liposome, co-delivery OVA peptide antigen with TLR4 ligand MPLA formulated GM3 liposome induced a more robust antigen-specific immune response than the GM3 liposome formulated with lipid-modified TLR7/8 ligand 3 M–052 [Citation131]. The weak interaction between the 3 M–052 and liposome may decrease the co-delivery efficacy of 3 M–052 with antigen which indicates that unstable binding of adjuvant could be the reason for inadequate immune response.
Archaeosomes are liposomes that contain distinct lipids derived from archaea, naturally extracted archaea lipids, or semi-synthetic archaea phosphoglycerolipids. Archaeosomes were reported to induce long-lasting functional antibodies production and perforin-dependent, T helper cell-independent cytotoxic T cells activities. For example, the OVA peptide entrapped within archaeosomes antigen-induced OVA-specific CD8+ T cell responses [Citation132,Citation133]. For tuberculosis vaccine development, the antigen Rv3619c-encapsulated archaeosomes induced 3 times higher IFN-γ, 5 times higher IL-12 than the free antigen vaccine in mice. It is also superior to the free antigen inducing a Th1-related immune response and can better protects the immunized mouse from the Mycobacterium tuberculosis infection challenge [Citation134]. An improved archaeosome formulation containing a single type of sulfated archaeal core lipid (SLA) provided a more accessible and lower-cost way for synthesizing archaeosomes as an adjuvant for encapsulated antigens [Citation135]. SLA and lactosyl-archaeol (LA) co-formulated archaeosomes are stable in structure and can enhance OVA-specific IgG titer and OVA-specific immune response more than the SLA archaeosomes. The SLA/LA archaeosomes showed comparable adjuvanticity with traditional archaeosomes. Using SLA archaeosomes with immune checkpoint inhibitors proved effective in inducing a robust cellular immune response and delaying the B16-OVA tumor progress in a mouse model [Citation136].
While most antigens assessed by liposome capture techniques are nucleic acids, proteins and peptides, other approaches have entrapped polysaccharide antigen molecules derived from two different serotypes of Streptococcus pneumoniae [Citation137]. These polysaccharides have traditionally served as the active ingredients of vaccines against pneumococcal disease, and they were encapsulated using a liposomal encapsulation of polysaccharides system (LEPS). The LEPS combined core-loaded polysaccharides with surface-displayed protein antigens and was an effective vaccine system to protect aged mice against S. pneumoniae infection [Citation138].
5. Antigen display on liposomes surface
Displaying antigens on the liposomal surface can mimic natural antigen display for recognition by immune cells. Displaying conformationally intact arrayed antigens on the surface enables recognition by B cell receptors, B cell crosslinking and antibody maturation. Displaying antigens on the surface of liposomes has been a widely used approach for liposome capture of antigens. While passive complexation may not achieve a stable display of antigens, efficacy can be modulated by varying variables such as incubation temperature during protein and liposome admixture. For example, a gentle and controlled thermal protein unfolding procedure has been shown to promote the interaction of recombinant factor VIII with phosphatidylserine-containing liposomes for immune tolerance induction [Citation139]. This section deals with approaches for antigen association, including lipid headgroup conjugation, metal chelation with His-tag coordination, and lipid tail anchoring.
5.1. Antigen conjugation to lipid headgroups
Conjugation of antigens with lipids allows the loading of antigen and adjuvant on the same particle and benefits the co-delivery of both to immune cells. The orientation of displayed antigen and exposed recognition site (related to secondary structure) for APCs impact vaccine immunogenicity. Lipid headgroup anchoring requires reaction between antigen and lipid head, using modified lipid chemistries such as the thiol groups of cysteine residues covalently coupled with maleimide lipid, or the conjugation of oxime-linked modified protein with modified αGalCer lipid as shown in . In a liposome-based SARS-CoV‑2 vaccine study, the receptor-binding domain (RBD) protein covalently bound with αGalCer by oxidizing the N-terminal amine of protein to ketone followed by oxime formation to form the αGalCer-RBD conjugate [Citation40]. The lipid tail of αGalCer inserts into the liposome bilayer and displays the RBD protein on both the inner and outer surface in a designed orientation. Compared with liposomes containing the unconjugated mix of RBD protein and αGalCer (RBD/αGalCer), the RBD-conjugated liposomal vaccine (αGalCer-RBD) injected mice induced 3 times higher expression of CD86 marker as an indication of activated DCs, increased anti-RBD IgG1, IgG2a, and IgG2b antibody titer, activated both CD4-mediated and CD8-mediated immune response. Liposomes containing the αGalCer-RBD conjugate proved better at inhibiting the binding between RBD and ACE2 in vitro. Since the immune-stimulating lipid αGalCer was included in all formulations, the surface-displaying antigen strategy proved to enhance the immunogenicity of the RBD liposomal vaccine.
Figure 6. Antigen conjugation with lipid headgroups for antigen display on liposome surfaces. a) an αGalCer-RBD conjugate anchors the lipid bilayer and displays RBD on liposomes [Citation40]. b) Aβ1–16 linked with PEGylated-lysine residues on both C- and N-terminal and anchored with DSPE lipid to display Aβ1–16 antigens [Citation114]. c) fusion peptide anchored on cholesterol (pepE-Bet v 1-CC-CPK-liposome) and displayed peptide on the surface [Citation109]. d) antigen modified with C-terminal cysteine covalently binds with maleimide-based liposome [Citation115]. Used with permission from the American Chemical Society, copyright 2022; National Academy of Sciences, copyright 2007; frontiers, copyright 2023; and American Society for Microbiology, copyright 2017.
![Figure 6. Antigen conjugation with lipid headgroups for antigen display on liposome surfaces. a) an αGalCer-RBD conjugate anchors the lipid bilayer and displays RBD on liposomes [Citation40]. b) Aβ1–16 linked with PEGylated-lysine residues on both C- and N-terminal and anchored with DSPE lipid to display Aβ1–16 antigens [Citation114]. c) fusion peptide anchored on cholesterol (pepE-Bet v 1-CC-CPK-liposome) and displayed peptide on the surface [Citation109]. d) antigen modified with C-terminal cysteine covalently binds with maleimide-based liposome [Citation115]. Used with permission from the American Chemical Society, copyright 2022; National Academy of Sciences, copyright 2007; frontiers, copyright 2023; and American Society for Microbiology, copyright 2017.](/cms/asset/76c4a970-e475-46d3-be30-17ca63664c05/ierv_a_2274479_f0006_oc.jpg)
In an Alzheimer’s Disease (AD) vaccine study, two types of antigen-displaying strategies were compared [Citation114]. The tetra-palmitoylated Aβ 1–15 liposomal vaccine-induced immune response was inclined to IgG antibody production. However, the Aβ 1–16 embedded into liposome by PEG (ACI-01) was more likely to produce IgM responses (). Analyzing the conformation of peptides within those liposomal anchors, researchers found the peptides between PEG spacers formed random coils and were more unstructured. In contrast, those tetra-palmitoylated peptides contained more stable β sheets. Besides serving as a linkage between antigen and liposome, the PEGylation is also used to prolong liposome half-life in the body, making it invisible to mononuclear phagocytic system cells. As the PEG length increases, the particle size of the nanoparticles will increase, and the degradation of the encapsulated antigen by DC will slow down. However, the PEG length should be designed to exhibit ideal-binding efficiency with nanoparticles to enhance nanoparticle uptake percentage, such as the middle-length PEG-3000 coated nanoparticle improved antibody-DC recognition and further facilitated T cell proliferation benefits the liposomal delivery system [Citation140].
The formation of a coiled-coil (CC) structure between two complementary α-helical peptides, the pepE, and the pepK, provided a new strategy for antigen capture and display on liposome surface [Citation110]. The pepK can be conjugated to liposome component cholesterol, while the pepE can covalently bind with antigen protein OVA323. The pepK and pepE interaction proved to increase antigen OVA323 loading efficacy on liposome and promote CD4+ T cell proliferation to induce more production of IFN-y and IL-10. Based on the same principle, a birch pollen allergy vaccine design study linked the pepE and antigen protein Bet v1 by pepE-Bet v 1 gene transfection of E. coli [Citation109]. The pepE-Bet v 1 protein interacted with cholesterol-conjugated pepK (), resulting in interfered IgE antibody binding and cross-linking and a more balanced Th1/Th2 immune response.
A thiol-maleimide reaction between maleimide and sulfhydryl groups of cysteine can achieve covalently binding between cysteine-modified antigens on maleimide lipids (). In a comparison study, this covalent binding proved to be superior to the two types of metal-His noncovalent binding in inducing stable antigen capture with increased germinal center marker GL-7 expression on B cells from 9% (Nickel), 12% (Cobalt) to 24% (Cys-linked) [Citation115]. However, a higher density of maleimide lipid was required to get the same amount of antigen loaded on liposomes compared to nickel- or cobalt-modified liposomes.
5.2. Metal-chelating lipids for binding his-tagged antigens
Another antigen-loading method is based on metal-chelating lipids. The imidazole group on the histidine side chain can coordinate with electrons in metal atoms, such as cobalt and nickel to form a stable complex. Since the His-tag (a stretch of 6–10 histidine residues) is commonly used in protein purification, this is a convenient approach for assessing antigen display on liposomes. As reported, the position changes of His-tag from N to C terminal of antigen epitope can influence the immune response due to steric hindrance rather than overall conformations [Citation117]. The His-tag linked antigen bound on the nickel metal-chelated liposome can be visualized under electron microscopy (EM) (). A study of vaccine design against HIV-1 envelope glycoprotein utilized the nickel-bearing liposomes and conserved CD4 binding site receptor (CD4bs) as antigen, which has a naturally masked N-glycan binding site for B cell recognition [Citation116]. To activate B cell response to the CD4bs, researchers designed a strategy to selectively activate B cells that can recognize and respond to CD4bs, followed by heterologous native flexibly linked (NFL) trimers boosting to elicit broadly neutralizing antibodies against HIV-1 envelope glycoprotein (Env). His-tagged Env NFL trimers bound on nickel-bearing liposome, as shown in .
Figure 7. Surface display of His-tagged antigen with metal chelating liposomes. electron micrographs of a a) plain nanoliposome, b) metal chelated liposome display the N-terminal linked His-tagged proteins (labeled with gold nanoparticles) on the liposome surface [Citation117]. c) His-tagged Env NFL trimer displayed on nickel-bearing liposomes [Citation116]. d) His-tag and cysteine-modified MD39 antigen captured by liposomes containing Ni-NTA and MPB [Citation141]. E) nickle- or cobalt-modified headgroup lipids containing liposome noncovalently capture His-tagged antigens [Citation115]. With permission from PLOS, copyright 2016; cell press, copyright 2019; Nature portfolio, copyright 2018; and American Society for Microbiology, copyright 2017.
![Figure 7. Surface display of His-tagged antigen with metal chelating liposomes. electron micrographs of a a) plain nanoliposome, b) metal chelated liposome display the N-terminal linked His-tagged proteins (labeled with gold nanoparticles) on the liposome surface [Citation117]. c) His-tagged Env NFL trimer displayed on nickel-bearing liposomes [Citation116]. d) His-tag and cysteine-modified MD39 antigen captured by liposomes containing Ni-NTA and MPB [Citation141]. E) nickle- or cobalt-modified headgroup lipids containing liposome noncovalently capture His-tagged antigens [Citation115]. With permission from PLOS, copyright 2016; cell press, copyright 2019; Nature portfolio, copyright 2018; and American Society for Microbiology, copyright 2017.](/cms/asset/5da2273b-e043-4f41-aa46-7512a4652239/ierv_a_2274479_f0007_oc.jpg)
3(nitrilotriacetic acid)-ditetradecylamine (NTA3-DTDA) contains three Ni2+ chelating moieties and can anchor His-tagged peptides to the surface through Ni-NTA and histidine coordination. Compared to the NTA3-DTDA, the NTA-DTDA contains only one NTA in the lipid head group showing weaker binding efficiency with histidine [Citation142]. A lipid approach in which OVA protein is encapsulated within the liposome, the Ni-NTA3-DTDA containing liposome captured and displayed His-tagged flagellin-related peptides 9Flg or 42Flg on the surface proved able to target the TLR5 expressed APC cells [Citation143]. The TLR5 ligand-decorated liposome increased liposome binding to TLR5-expressing cells HEK-293 and HepG2. Mice immunized with the 9Flg-OVA-liposome or 42Flg-OVA-liposome show increased OVA-specific IgG antibody titer and decreased tumor metastasis to lung tissue.
When the OVA peptide is modified with a His-tag, NTA3-DTDA containing stealth liposomes (SLs) can display the His-tagged OVA on both the outer hydrophilic surface and inner hydrophilic area by rehydration lipid film during the synthesis procedure [Citation144]. Based on the OVA-SLs, different His-tagged targeting molecules single chain full-length variable Ab fragments (ScFv) were incorporated with OVA-SLs suspension. Liposomes decorated with different dendritic cells targeting ScFv, the His-tagged DEC-205-ScFv, could target dendritic cells and inhibit tumor metastasis to lung tissue. In comparison, the vaccine without the decoration of DEC-205-ScFv failed to inhibit tumor metastasis to lung tissue.
In an HIV vaccine design, liposomes containing Ni-NTA headgroup lipid immobilized stabilized soluble SOSIP trimers, MD39 [Citation141]. The His-tag on the MD39 C-terminal coordinated with Ni-NTA containing liposome and displayed MD39 in a designed orientation mimicking the natural virus structure. To capture the MD39 more stable at 10% serum, the additional interaction between cysteine and maleimide-functionalized lipids (MPB) is important. MD39 antigen was synthesized with six His-tag followed by a cysteine on the C-terminal, coordinated with Ni-NTA and MPB separately, and displayed on the liposome surface (). Results showed an improved stable capture of the high density of MD39 by liposome after the additional introduction of MPB. In contrast, the high-density MD39 on liposome resulted in B cell activation, which showed benefit antigen-specific antibody production in mouse studies.
It has been demonstrated that in vivo, his-tagged antigens do not stably bind to multivalent Ni-NTA lipids, and improvements in chelator or ligand design are needed before metal chelation will be capable of retaining histidine-modified proteins on NTA liposomes after in-vivo-administration [Citation145]. A more stable capture of antigens on liposomes could bring a more robust antigen-specific immune response.
An alternative metal chelating strategy is to capture His-tagged antigens by cobalt-ion chelated liposomes (). The cobalt-incorporated liposomes showed a stable binding with His-tagged antigen in 20% serum at 37°C [Citation112,Citation115]. The cobalt-modified lipid head group liposome DGS-NTA(Co2+) showed an enhanced B cell activation in response to His-tagged BG505 NFL trimer antigen than the DGS-NTA(Ni2+) liposomes did [Citation115].
By modifying the lipid tail with cobalt (instead of the headgroup), CoPoP liposomes containing the cobalt-modified CoPoP lipid and the TLR4 ligand PHAD stably capture and display His-tagged antigens on the liposome surfaces (). In an infectious diarrhea vaccine study, the His-tagged recombinant enterotoxigenic Escherichia coli colonization factor subunit proteins CfaEB and CfaEad were displayed on CoPoP/PHAD liposome surface [Citation113]. The antigen in the form of nanoparticles resulted in an increased amount of antigen uptake by macrophages significantly and promoted the production of functional antibodies to neutralize live Escherichia coli in vitro. The small size antigen CfaEad displayed on CoPoP/PHAD liposome induced about 100 times higher serum IgG antibody titer compared to antigen incubated with liposome lacking cobalt (PoP/PHAD) [Citation113]. The CoPoP/PHAD liposome can carry different protein antigens exposed to the multistage life cycle of parasites as a multivalent vaccine [Citation107]. More than 80% of antigen was captured by CoPoP/PHAD liposomes compared to antigen binding below 10% for PoP/PHAD liposomes. The antigen-specific IgG titer induced by CoPoP/PHAD formulated vaccine was 1000 times higher than the IgG titer induced by the PoP/PHAD-formulated vaccine. Mice immunized with the multivalent CoPoP/PHAD liposomal vaccine, which displays His-Pfg27, His-Pfs25, His-Pfs230, and His-AMA-1 protein antigen all on the same nanoparticle, exanimated serum IgG titer remain the same level as monovalent vaccine and proved to be functional on recognizing life-stages covered parasite () [Citation107]. The CoPoP liposome containing MPLA with surface-displayed His-tag RBD vaccine (EuCorVac-19) proved to be safe and able to induce neutralizing antibodies in humans according to a recent clinical phase II study (NCT04783311) [Citation146].
Figure 8. Surface displaying of His-tagged peptide antigen with CoPoP/PHAD/QS21 liposomes as an anti-cancer vaccine. a) His-tagged polypeptides insert into lipid bilayer by binding with CoPoP [Citation104]. b) His-tagged peptide displayed on CoPoP/PHAD/QS21 liposome via coordination with CoPoP lipid [Citation108]. c) Nes2LR captured on liposome CoPoP/PHAD/QS21 induced Nes2LR specific CD8 T cells while Nes2LR adjuvanted with PolyIC is low in immunogenicity [Citation108]. d) schematic of liposomal malaria vaccine immunization based on His-tagged Psf25 protein antigen and CoPoP liposome [Citation107]. Used with permission from Nature, copyright 2015; BMJ, copyright 2021; Springer Nature, copyright 2018.
![Figure 8. Surface displaying of His-tagged peptide antigen with CoPoP/PHAD/QS21 liposomes as an anti-cancer vaccine. a) His-tagged polypeptides insert into lipid bilayer by binding with CoPoP [Citation104]. b) His-tagged peptide displayed on CoPoP/PHAD/QS21 liposome via coordination with CoPoP lipid [Citation108]. c) Nes2LR captured on liposome CoPoP/PHAD/QS21 induced Nes2LR specific CD8 T cells while Nes2LR adjuvanted with PolyIC is low in immunogenicity [Citation108]. d) schematic of liposomal malaria vaccine immunization based on His-tagged Psf25 protein antigen and CoPoP liposome [Citation107]. Used with permission from Nature, copyright 2015; BMJ, copyright 2021; Springer Nature, copyright 2018.](/cms/asset/9cadc986-bab5-454c-a621-e924a490d46d/ierv_a_2274479_f0008_oc.jpg)
The CoPoP/PHAD liposome formulated with saponin QS21 (CoPoP/PHAD/QS21 liposome) induced a greater frequency of antigen-specific CD8+ T cell compared with poly(I:C) adjuvanted peptide vaccine, even at 10,000 times lower peptide antigen dosing (). Over 80% of His-tagged peptides bound on cobalt contained liposomes while the liposome without cobalt had almost no binding affinity to his-tagged peptides [Citation106]. As a result, the cobalt-chelated liposomal vaccine induced more than 15% antigen-specific CD8 T cells and can prevent tumor growth, but the liposome lacking cobalt was not immunogenic and cannot inhibit tumor. The CoPoP/PHAD liposome is also a powerful tool for potential antigen screening. For example, a neoantigen Nes2LR was discovered from 20 predicted neoepitopes that were chosen from a total of 1014 candidates. The discovered neoepitope Nes2LR-displayed CoPoP/PHAD/QS21 liposomal vaccine proved to induce a high frequency of antigen-specific CD8+ T cells in the blood of immunized mice and inhibit Renca tumor growth [Citation108].
5.3. Lipid tail anchoring
Lipopeptides can be displayed on the liposome surface during the vaccine preparation and have been shown to be more immunogenic than soluble peptide antigens in the liposomal delivery system. Anchoring the lipid tail of lipopeptide into the lipid bilayer can also display antigen on the liposome surface in a designed orientation for immune response activation. For example, the E7-lipopeptide consists of the HPV16 protein-derivatives E749–57 peptide linked with peptide Lys-Ser-Ser (KSS), which is then attached with palmitic acid on Lys residue () [Citation111]. By evaporating the E7-lipopeptide with cationic lipid DOTAP and rehydrating the lipopeptide/lipid films followed by sonication and extrusion processes, lipopeptide can be incorporated with liposome. Lipopeptide was shown not to affect liposome size and charge but significantly increased antigen loading efficacy of DOTAP liposome from 25% (soluble E7 peptide and unlipidated KSS-E7 peptide) to 90% (E7 lipopeptide). Compared with soluble E7 peptide-loaded DOTAP liposomes, the DOTAP/E7-Lipopeptide proved to better inhibit TC-1 tumor growth in a therapeutic mouse model with a single injection. This could be explained by the increased antigen peptide exposed to APCs via MHC I molecule, which activates naïve CD8 T cells to proliferate and secrete cytotoxic cytokine IFN-γ. However, the total amount of lipopeptide that can be loaded is limited at a high lipopeptide molecular ratio.
Figure 9. Schematic of peptide antigens anchored on liposomes. a) E7 peptide linked with peptide Lys-Ser-Ser which attached with palmitic acid on the Lys residue to form lipopeptide and anchored on lipid bilayer of liposome [Citation111]. b) synthetic CV2 peptide with N-terminal palmitic acid (palm-CV2) anchored CV2 peptide to the lipid bilayer [Citation105]. c) palmitoylated β-amyloid peptide Aβ1–15 displayed on liposome surface [Citation114]. With permission American Chemical Society, copyright 2008; Springer Nature, copyright 2017; and National Academy of Sciences of the U.S.A., copyright 2007.
![Figure 9. Schematic of peptide antigens anchored on liposomes. a) E7 peptide linked with peptide Lys-Ser-Ser which attached with palmitic acid on the Lys residue to form lipopeptide and anchored on lipid bilayer of liposome [Citation111]. b) synthetic CV2 peptide with N-terminal palmitic acid (palm-CV2) anchored CV2 peptide to the lipid bilayer [Citation105]. c) palmitoylated β-amyloid peptide Aβ1–15 displayed on liposome surface [Citation114]. With permission American Chemical Society, copyright 2008; Springer Nature, copyright 2017; and National Academy of Sciences of the U.S.A., copyright 2007.](/cms/asset/8080078d-049e-41a8-8393-64194368b444/ierv_a_2274479_f0009_oc.jpg)
Lipopeptide liposomal vaccines against group A streptococcus (GAS) infection are of interest. The GAS M protein-derived J14 peptide and T-helper epitope P25 were lipidated and incorporated with liposomes [Citation147]. Following the intranasal immunizations, compared with lipopeptide-only immunized mice, the mice that received the liposome-incorporated lipopeptide vaccine showed a IgG2a titer increase which is preferred for an effective GAS infection treatment.
In a dual-use vaccine development study, the heroin antigen TT-MorHap (MorHap conjugated to tetanus toxoid) and HIV antigens V2 peptides (cyclic V2, CV2; linear V2, LV2) were co-delivered by ALF liposomes. The palmitic acid connected with HIV-1 envelope V2 peptide to form lipopeptide, which can anchor the bilayer of liposome () [Citation105]. The palm-CV2 peptide and palm-LV2 showed a mixture of α-helical and coil structure in 50% trifluoroethanol or 1% SDS condition but changed to exhibit completely random coil or completely β-sheet after incorporation onto ALF liposome separately. The structure difference exposes more binding sites for plamCV2 to be recognized by APCs cells, which could explain why palmCV2-induced anti-V2 antibodies have more binding targets than the plamLV2 did. Though the anti-CV2 antibody level dropped significantly with the co-delivery of TT-MorHap, the anti-gp70-V1V2, and anti-gp120 antibody titer level was kept the same. The result showed that the dual liposome vaccine did not affect the antibody titer induced by heroin hapten. This dual-vaccine system is promising to treat HIV and prevent heroin addiction simultaneously.
The structural changes of liposome displayed antigen could affect the vaccine immunogenicity, as reported in an AD vaccine study mentioned previously. The palmitoylated β-amyloid peptide Aβ1–15 (lipopeptide palmAβ1–15) anchored on the liposome surface (ACI-24 liposome, ) induced 2 times higher level of anti-Aβ1–42 IgG antibody than the lipid head conjugated liposomal vaccine ACI-01 did [Citation114]. Lipopeptide consisting of palmitoylated β-amyloid peptide Aβ1–15 displayed a defined β-sheet conformation when incorporated onto the liposome surface, while the PEGylated Aβ1–16 showed a random secondary structure when incorporated onto the liposome surface. The lipopeptide-formulated AD vaccine-immunized mice exhibited restored cognitive memory and decreased brain amyloid. The defined β-sheet conformation of ACI-24 displayed palmAβ1–15 may expose the critical binding site for the induction of functional antibodies. A clinical trial (NCT02738450) investigated the safety, tolerance, and immunogenicity of the ACI-24 vaccine with 16 adults with Down syndrome (people at high risk for Alzheimer’s Disease) [Citation148]. Two subcutaneous injections of the ACI-24 vaccine proved safe and well-tolerated. 4 of the 12 volunteers who received the ACI-24 vaccine showed increased anti-Aβ IgG antibody titer, and both plasma Aβ1–40 and Aβ1–42 levels were increased for ACI-24 groups as evidence of ACI-24 immunogenicity. Currently, a phase 1b/2 study (NCT05462106) aiming to investigate the ACI-24 vaccine on volunteers with prodromal Alzheimer’s disease or with Down syndrome only is ongoing. An estimated 140 volunteers will participate in this study to investigate vaccine safety, tolerance, immunogenicity, and pharmacodynamic effects.
Viral antigens likely benefit from being displayed on liposome surfaces to mimic the natural presentation of the viral antigen without losing its functional binding site for producing neutralizing antibodies. The viral membrane-proximal external region peptide (MPER) can lose the binding site for neutralizing antibodies after binding with lipid nanoparticles or membranes. In this circumstance, modifying the antigen to display it on the nanoparticle surface while maintaining its natural structure could be achieved by lipid conjugation strategy. An HIV vaccine development study compared the immunogenicity of the liposome-anchored viral envelope protein MPER vaccine and the oil-based emulsion (AddVax) adjuvanted, water-in-oil emulsion (Montanide) adjuvanted or the Alum adjuvanted MPER vaccines [Citation101]. The MPER synthetic with palmitoyl tail on the N-terminal (pMPER) was dried with different ratios of 1,2-dimyristoyl-sn-glycero-3-phosphocholine (DMPC), DOPC, and 1,2-Dioleoyl-sn-glycero-3-PG (DOPG) lipids and rehydrated to form liposomes. The TLR4 agonist MPLA was added to all formulations to enhance vaccine immunogenicity. Due to the low immunogenicity of MPER antigen, only the MPLA-assisted pMPER anchored liposome induced high anti-MPER IgG antibody titer in the mouse model. Without MPLA, the liposomal pMPER only induced low anti-MPER IgG titer.
Palmitic acid is not the only lipid anchor for peptide antigens. For example, compared to palmitic acid and phosphatidylcholine, cholesteryl hemisuccinate (CHEMS) conjugated to the C-terminal of the membrane proximal region (MPR) peptide of HIV-1 gp41 induced a stronger anti-peptide IgG titer in serum [Citation102]. In addition, the poly-hydrophobic amino acid tail of antigen peptides could also anchor the antigen into a liposome bilayer. Group A Streptococcus epitopes (GAS peptide) conjugated with polyleucine tail can self-assemble into a liposome bilayer during the hydration process to display antigens [Citation149]. Compared to unmodified antigens (76%), antigens anchored on the liposome by poly-leucine (95%) showed higher encapsulation efficiency. Also, only the liposomal vaccine with poly-leucine conjugated antigen showed opsonic ability against GAS bacteria.
6. Conclusion and outlook
Admixing antigens with adjuvant-loaded liposomes is a common method to prepare vaccines. Although in this case liposomes do not necessarily capture antigens, they can still deliver adjuvants to stimulate enhanced immune responses. Liposomal antigen capture strategies stand to further benefit vaccine development. Antigens can be captured in the aqueous core of liposomes. Charged liposomes can complex antigens through electrostatic forces, and the current mRNA LNP-based vaccines can be considered a special example of this. His-tagged antigens can coordinate with metal-chelated liposomes. Promising solutions to display antigens on liposome surfaces include using lipopeptide anchors or linking antigens with lipid headgroups.
Overall, liposomal vaccines are a clinically relevant platform for the delivery of antigens and adjuvants together. Liposomal vaccine composition, surface decoration, antigen loading strategies, immunization routes, and combinations of adjuvants are all worth investigating. Liposomal vaccine systems are poised for further improvements as new adjuvants, antigens and antigen capture strategies are developed.
Article highlights
Liposomes are broadly used as versatile vaccine adjuvant carriers
Coupling antigens with liposomes can enhance immunogenicity
Many liposomal approaches for antigen capture have been developed, some progressing to clinical testing
Declaration of interests
J.F Lovell holds an interest in POP Biotechnologies. Other authors declare no relevant competing interests. The authors have no other relevant affiliations or financial involvement with any organization or entity with a financial interest in or financial conflict with the subject matter or material discussed in the manuscript apart from those disclosed.
Reviewer disclosures
A reviewer on this manuscript is a current employee at GlaxoSmithKline. Peer reviewers on this manuscript have no other relevant financial or other relationships to disclose.
Author contributions
All authors contributed to the conception and design of the review article and interpretation of the relevant literature and were involved in writing the review article or revising it for intellectual content.
Additional information
Funding
References
- Liu P, Chen G, Zhang J. A review of liposomes as a Drug delivery system: Current status of approved products, regulatory environments, and future perspectives. Molecules. 2022;27(4):1372. doi:10.3390/molecules27041372
- Badiee A, Khamesipour A, Samiei A, et al. The role of liposome size on the type of immune response induced in BALB/c mice against leishmaniasis: rgp63 as a model antigen. Exp Parasitol. 2012;132(4):403–409. doi: 10.1016/j.exppara.2012.09.001
- Mann JF, Shakir E, Carter KC, et al. Lipid vesicle size of an oral influenza vaccine delivery vehicle influences the Th1/Th2 bias in the immune response and protection against infection. Vaccine. 2009;27(27):3643–3649. doi:10.1016/j.vaccine.2009.03.040
- Ogue S, Takahashi Y, Onishi H, et al. Preparation of double liposomes and their efficiency as an oral vaccine carrier. Biol Pharm Bull. 2006;29(6):1223–1228. doi:10.1248/bpb.29.1223
- Fries LF, Gordon DM, Richards RL, et al. Liposomal malaria vaccine in humans: a safe and potent adjuvant strategy. Proc Natl Acad Sci U S A. 1992;89(1):358–362. doi: 10.1073/pnas.89.1.358
- Barenholz Y. Doxil®–the first FDA-approved nano-drug: lessons learned. J Control Release. 2012;160(2):117–134. doi:10.1016/j.jconrel.2012.03.020
- Bulbake U, Doppalapudi S, Kommineni N, et al. Liposomal formulations in clinical use: an updated review. Pharmaceutics. 2017;9(4):12. doi: 10.3390/pharmaceutics9020012
- Monie A, Hung CF, Roden R, et al. Cervarix: a vaccine for the prevention of HPV 16, 18-associated cervical cancer. Biologics. 2008;2(1):97–105.
- Harper DM, DeMars LR. HPV vaccines - a review of the first decade. Gynecol Oncol. 2017;146(1):196–204. doi:10.1016/j.ygyno.2017.04.004
- Singh G, Song S, Choi E, et al. Recombinant zoster vaccine (Shingrix(®)): a new option for the prevention of herpes zoster and postherpetic neuralgia. Korean J Pain. 2020;33(3):201–207. doi:10.3344/kjp.2020.33.3.201
- Chatzikleanthous D, O’Hagan DT, Adamo R. Lipid-based nanoparticles for delivery of vaccine adjuvants and Antigens: toward multicomponent vaccines. Mol Pharmaceut. 2021;18(8):2867–2888. doi:10.1021/acs.molpharmaceut.1c00447
- Raz A, Bucana C, Fogler WE, et al. Biochemical, morphological, and ultrastructural studies on the uptake of liposomes by murine macrophages. Cancer Res. 1981;41(2):487–494.
- Hsu MJ, Juliano RL. Interactions of liposomes with the reticuloendothelial system: II. Nonspecific and receptor-mediated uptake of liposomes by mouse peritoneal macrophages. Biochim Biophys Acta, Mol Cell Res. 1982;720(4):411–419. doi:10.1016/0167-4889(82)90120-3
- Allen TM, Austin GA, Chonn A, et al. Uptake of liposomes by cultured mouse bone marrow macrophages: influence of liposome composition and size. Biochim Biophys Acta - Biomembr. 1991;1061(1):56–64. doi:10.1016/0005-2736(91)90268-D
- Goronzy JJ, Weyand CM. T-cell co-stimulatory pathways in autoimmunity. Arthritis Res Ther. 2008;10(1):S3. doi:10.1186/ar2414
- Lumsden JM, Williams JA, Hodes RJ. Differential requirements for expression of CD80/86 and CD40 on B cells for T-Dependent antibody responses in vivo. J Immunol. 2003;170(2):781–787. doi:10.4049/jimmunol.170.2.781
- Chen L, Flies DB. Molecular mechanisms of T cell co-stimulation and co-inhibition. Nat Rev Immunol. 2013;13(4):227–242. doi:10.1038/nri3405
- Cyster JG, Allen CDC. B cell responses: cell interaction dynamics and decisions. Cell. 2019;177(3):524–540. doi:10.1016/j.cell.2019.03.016
- Ragupathi G, Gardner JR, Livingston PO, et al. Natural and synthetic saponin adjuvant QS-21 for vaccines against cancer. Expert Rev Vaccines. 2011;10(4):463–470. doi:10.1586/erv.11.18
- Wang P. Natural and synthetic saponins as vaccine adjuvants. Vaccines. 2021;9(3):222. doi:10.3390/vaccines9030222
- Beck Z, Matyas GR, Alving CR. Detection of liposomal cholesterol and monophosphoryl lipid a by QS-21 saponin and limulus polyphemus amebocyte lysate. Biochim Biophys Acta. 2015;1848(3):775–780. doi:10.1016/j.bbamem.2014.12.005
- Lacaille-Dubois MA. Updated insights into the mechanism of action and clinical profile of the immunoadjuvant QS-21: a review. Phytomedicine. 2019;60:152905. doi:10.1016/j.phymed.2019.152905
- Genito CJ, Beck Z, Phares TW, et al. Liposomes containing monophosphoryl lipid a and QS-21 serve as an effective adjuvant for soluble circumsporozoite protein malaria vaccine FMP013. Vaccine. 2017;35(31):3865–3874. doi: 10.1016/j.vaccine.2017.05.070
- He X, Zhou S, Huang W-C, et al. A potent cancer vaccine adjuvant system for particleization of short, synthetic CD8+ T cell epitopes. ACS Nano. 2021;15(3):4357–4371. doi: 10.1021/acsnano.0c07680
- Alving CR, Rao M, Steers NJ, et al. Liposomes containing lipid A: an effective, safe, generic adjuvant system for synthetic vaccines. Expert Rev Vaccines. 2012;11(6):733–744. doi:10.1586/erv.12.35
- Wang YQ, Bazin-Lee H, Evans JT, et al. MPL adjuvant contains competitive antagonists of human TLR4. Front Immunol. 2020;11:577823. doi:10.3389/fimmu.2020.577823
- Schulke S, Flaczyk A, Vogel L, et al. MPLA shows attenuated pro-inflammatory properties and diminished capacity to activate mast cells in comparison with LPS. Allergy. 2015;70(10):1259–1268. doi: 10.1111/all.12675
- Watanabe S, Kumazawa Y, Inoue J, et al. Liposomal lipopolysaccharide initiates TRIF-Dependent signaling pathway Independent of CD14. PLoS One. 2013;8(4):e60078. doi:10.1371/journal.pone.0060078
- Alving CR, Peachman KK, Matyas GR, et al. Army liposome formulation (ALF) family of vaccine adjuvants. Expert Rev Vaccines. 2020;19(3):279–292. doi:10.1080/14760584.2020.1745636
- Jobe O, Kim J, Pinto DO, et al. Army liposome formulation containing QS-21 render human monocyte-derived macrophages less permissive to HIV-1 infection by upregulating APOBEC3A. Sci Rep. 2022;12(1):7570. doi: 10.1038/s41598-022-11230-8
- Bauer S, Kirschning CJ, Häcker H, et al. Human TLR9 confers responsiveness to bacterial DNA via species-specific CpG motif recognition. Proc Nat Acad Sci. 2001;98(16):9237–9242. doi: 10.1073/pnas.161293498
- Valencia-Hernandez AM, Zillinger T, Ge Z, et al. Complexing CpG adjuvants with cationic liposomes enhances vaccine-induced formation of liver T(RM) cells. Vaccine. 2023;41(5):1094–1107. doi: 10.1016/j.vaccine.2022.12.047
- Badiee A, Jaafari MR, Samiei A, et al. Coencapsulation of CpG oligodeoxynucleotides with recombinant leishmania major stress-inducible protein 1 in liposome enhances immune response and protection against leishmaniasis in immunized BALB/c mice. Clin Vaccine Immunol. 2008;15(4):668–674. doi:10.1128/CVI.00413-07
- Lin Y, Xu J, Lan H. Tumor-associated macrophages in tumor metastasis: biological roles and clinical therapeutic applications. J Hematol Oncol. 2019;12(1):76. doi:10.1186/s13045-019-0760-3
- Li H, Somiya M, Kuroda S. Enhancing antibody-dependent cellular phagocytosis by re-education of tumor-associated macrophages with resiquimod-encapsulated liposomes. Biomaterials. 2021;268:120601. doi:10.1016/j.biomaterials.2020.120601
- Kasturi SP, Rasheed MAU, Havenar-Daughton C, et al. 3M-052, a synthetic TLR-7/8 agonist, induces durable HIV-1 envelope-specific plasma cells and humoral immunity in nonhuman primates. Sci Immunol. 2020;5(48). doi: 10.1126/sciimmunol.abb1025
- Abhyankar MM, Orr MT, Lin S, et al. Adjuvant composition and delivery route shape immune response quality and protective efficacy of a recombinant vaccine for entamoeba histolytica. NPJ Vaccines. 2018;3(1):22. doi: 10.1038/s41541-018-0060-x
- Short KK, Miller SM, Walsh L, et al. Co-encapsulation of synthetic lipidated TLR4 and TLR7/8 agonists in the liposomal bilayer results in a rapid, synergistic enhancement of vaccine-mediated humoral immunity. J Control Release. 2019;315:186–196. doi: 10.1016/j.jconrel.2019.10.025
- King LA, Lameris R, de Gruijl TD, et al. CD1d-invariant natural killer T cell-based cancer immunotherapy: α-galactosylceramide and beyond. Front Immunol. 2018;9:1519. doi:10.3389/fimmu.2018.01519
- Wang J, Wen Y, Zhou SH, et al. Self-adjuvanting lipoprotein conjugate αGalCer-RBD induces potent immunity against SARS-CoV-2 and its variants of concern. J Med Chem. 2022;65(3):2558–2570. doi: 10.1021/acs.jmedchem.1c02000
- Yin X-G, Lu J, Wang J, et al. Synthesis and evaluation of liposomal anti-GM3 cancer vaccine candidates covalently and noncovalently adjuvanted by αGalCer. J Med Chem. 2021;64(4):1951–1965. doi: 10.1021/acs.jmedchem.0c01186
- Li X, Fujio M, Imamura M, et al. Design of a potent CD1d-binding NKT cell ligand as a vaccine adjuvant. Proc Nat Acad Sci. 2010;107(29):13010–13015. doi: 10.1073/pnas.1006662107
- Zhang F, Stephan SB, Ene CI, et al. Nanoparticles that reshape the tumor milieu create a therapeutic Window for effective T-cell therapy in solid malignancies. Cancer Res. 2018;78(13):3718–3730. doi:10.1158/0008-5472.CAN-18-0306
- Yankowski C, Wirblich C, Kurup D, et al. Inactivated rabies-vectored SARS-CoV-2 vaccine provides long-term immune response unaffected by vector immunity. NPJ Vaccines. 2022;7(1):110. doi:10.1038/s41541-022-00532-7
- Kirby DJ, Rosenkrands I, Agger EM, et al. Liposomes act as stronger sub-unit vaccine adjuvants when compared to microspheres. J Drug Target. 2008;16(7):543–554. doi:10.1080/10611860802228558
- Lu P, Guerin DJ, Lin S, et al. Immunoprofiling correlates of protection against SHIV infection in adjuvanted HIV-1 pox-protein vaccinated rhesus macaques. Front Immunol. 2021;12:625030. doi: 10.3389/fimmu.2021.625030
- Barrientos RC, Bow EW, Whalen C, et al. Novel vaccine that blunts fentanyl effects and sequesters ultrapotent fentanyl analogues. Mol Pharm. 2020;17(9):3447–3460. doi: 10.1021/acs.molpharmaceut.0c00497
- Richards RL, Hayre MD, Hockmeyer WT, et al. Liposomes, lipid A, and aluminum hydroxide enhance the immune response to a synthetic malaria sporozoite antigen. Infect Immun. 1988;56(3):682–686. doi:10.1128/iai.56.3.682-686.1988
- Om K, Paquin-Proulx D, Montero M, et al. Adjuvanted HIV-1 vaccine promotes antibody-dependent phagocytic responses and protects against heterologous SHIV challenge. PLOS Pathog. 2020;16(9):e1008764. doi: 10.1371/journal.ppat.1008764
- Calderón L, Facenda E, Machado L, et al. Modulation of the specific allergic response by mite allergens encapsulated into liposomes. Vaccine. 2006;24 Suppl 2:S2-38–39. doi: 10.1016/j.vaccine.2005.01.112
- Rao M, Onkar S, Peachman KK, et al. Liposome-encapsulated human immunodeficiency virus-1 gp120 induces potent V1V2-specific antibodies in humans. J Infect Dis. 2018;218(10):1541–1550. doi: 10.1093/infdis/jiy348
- Beck Z, Torres OB, Matyas GR. et al. Immune response to antigen adsorbed to aluminum hydroxide particles: effects of co-adsorption of ALF or ALFQ adjuvant to the aluminum-antigen complex. J Control Release. 2018;275:12–19. doi: 10.1016/j.jconrel.2018.02.006
- Komla E, Torres OB, Jalah R, et al. Effect of preexisting immunity to tetanus toxoid on the efficacy of tetanus toxoid-conjugated heroin vaccine in mice. Vaccines (Basel). 2021;9(6):573. doi: 10.3390/vaccines9060573
- Auderset F, Belnoue E, Mastelic-Gavillet B, et al. A TLR7/8 agonist-including DOEPC-Based cationic liposome formulation mediates its adjuvanticity through the sustained recruitment of highly activated monocytes in a type I IFN-Independent but NF-κB-Dependent manner. Front Immunol. 2020;11:580974. doi:10.3389/fimmu.2020.580974
- Lal H, Cunningham AL, Godeaux O, et al. Efficacy of an adjuvanted herpes zoster subunit vaccine in older adults. N Engl J Med. 2015;372(22):2087–2096. doi: 10.1056/NEJMoa1501184
- Tomori Y, Iijima N, Hinuma S, et al. Morphological analysis of Trafficking and Processing of anionic and cationic liposomes in cultured cells. Acta Histochem Cytochem. 2018;51(2):81–92. doi: 10.1267/ahc.17021
- Nagy NA, Castenmiller C, Vigario FL, et al. Uptake kinetics of liposomal formulations of differing charge influences development of in vivo dendritic cell immunotherapy. J Pharm Sci. 2022;111(4):1081–1091. doi: 10.1016/j.xphs.2022.01.022
- Luo G, Yang Q, Yao B, et al. Slp-coated liposomes for drug delivery and biomedical applications: potential and challenges. Int J Nanomedicine. 2019;14:1359–1383. doi: 10.2147/IJN.S189935
- Su X, Fricke J, Kavanagh DG, et al. In vitro and in vivo mRNA delivery using lipid-enveloped pH-responsive polymer nanoparticles. Mol Pharm. 2011;8(3):774–787. doi:10.1021/mp100390w
- Hollmann A, Delfederico L, Glikmann G, et al. Characterization of liposomes coated with S-layer proteins from lactobacilli. Biochim Biophys Acta - Biomembr. 2007;1768(3):393–400. doi:10.1016/j.bbamem.2006.09.009
- Chatzikleanthous D, Schmidt ST, Buffi G, et al. Design of a novel vaccine nanotechnology-based delivery system comprising CpGODN-protein conjugate anchored to liposomes. J Control Release. 2020;323:125–137. doi: 10.1016/j.jconrel.2020.04.001
- Yanasarn N, Sloat BR, Cui Z. Negatively charged liposomes show potent adjuvant activity when simply admixed with protein antigens. Mol Pharm. 2011;8(4):1174–1185. doi: 10.1021/mp200016d
- Orr MT, Fox CB, Baldwin SL, et al. Adjuvant formulation structure and composition are critical for the development of an effective vaccine against tuberculosis. J Control Release. 2013;172(1):190–200. doi: 10.1016/j.jconrel.2013.07.030
- Kodar K, Harper JL, McConnell MJ, et al. The mincle ligand trehalose dibehenate differentially modulates M1-like and M2-like macrophage phenotype and function via syk signaling. Immun Inflamm Dis. 2017;5(4):503–514. doi:10.1002/iid3.186
- Schoenen H, Bodendorfer B, Hitchens K, et al. Cutting edge: mincle is essential for recognition and adjuvanticity of the mycobacterial cord factor and its synthetic analog trehalose-dibehenate. J Immunol. 2010;184(6):2756–2760. doi: 10.4049/jimmunol.0904013
- Thakur A, Andrea A, Mikkelsen H, et al. Targeting the Mincle and TLR3 receptor using the dual agonist cationic adjuvant formulation 9 (CAF09) induces humoral and polyfunctional memory T cell responses in calves. PLoS One. 2018;13(7):e0201253. doi: 10.1371/journal.pone.0201253
- Andersen CA, Rosenkrands I, Olsen AW, et al. Novel generation mycobacterial adjuvant based on liposome-encapsulated monomycoloyl glycerol from mycobacterium bovis bacillus calmette-guérin. J Immunol. 2009;183(4):2294–2302. doi: 10.4049/jimmunol.0804091
- Pedersen GK, Andersen P, Christensen D. Immunocorrelates of CAF family adjuvants. Semin Immunol. 2018;39:4–13. doi:10.1016/j.smim.2018.10.003
- van Dissel JT, Joosten SA, Hoff ST, et al. A novel liposomal adjuvant system, CAF01, promotes long-lived mycobacterium tuberculosis-specific T-cell responses in human. Vaccine. 2014;32(52):7098–7107. doi: 10.1016/j.vaccine.2014.10.036
- Abraham S, Juel HB, Bang P, et al. Safety and immunogenicity of the chlamydia vaccine candidate CTH522 adjuvanted with CAF01 liposomes or aluminium hydroxide: a first-in-human, randomised, double-blind, placebo-controlled, phase 1 trial. Lancet Infect Dis. 2019;19(10):1091–1100. doi: 10.1016/S1473-3099(19)30279-8
- Espinosa DA, Christensen D, Muñoz C, et al. Robust antibody and CD8(+) T-cell responses induced by P. falciparum CSP adsorbed to cationic liposomal adjuvant CAF09 confer sterilizing immunity against experimental rodent malaria infection. NPJ Vaccines. 2017;2(1). doi: 10.1038/s41541-017-0011-y
- Filskov J, Andersen P, Agger EM, et al. HCV p7 as a novel vaccine-target inducing multifunctional CD4+ and CD8+ T-cells targeting liver cells expressing the viral antigen. Sci Rep. 2019;9(1):14085. doi:10.1038/s41598-019-50365-z
- Zimmermann J, Schmidt ST, Trebbien R, et al. A novel prophylaxis strategy using liposomal vaccine adjuvant CAF09b protects against influenza virus disease. Int J Mol Sci. 2022;23(3):1850. doi: 10.3390/ijms23031850
- Vu PL, Vadakekolathu J, Idri S, et al. A mutated prostatic acid phosphatase (PAP) peptide-based vaccine induces PAP-Specific CD8(+) T cells with ex vivo cytotoxic capacities in HHDII/DR1 transgenic mice. Cancers (Basel). 2022;14(8):1970. doi: 10.3390/cancers14081970
- van Haren SD, Pedersen GK, Kumar A, et al. CAF08 adjuvant enables single dose protection against respiratory syncytial virus infection in murine newborns. Nat Commun. 2022;13(1):4234. doi: 10.1038/s41467-022-31709-2
- Kranz LM, Diken M, Haas H, et al. Systemic RNA delivery to dendritic cells exploits antiviral defence for cancer immunotherapy. Nature. 2016;534(7607):396–401. doi: 10.1038/nature18300
- Lou G, Anderluzzi G, Schmidt ST, et al. Delivery of self-amplifying mRNA vaccines by cationic lipid nanoparticles: the impact of cationic lipid selection. JControlled Release. 2020;325:370–379. doi: 10.1016/j.jconrel.2020.06.027
- Melo M, Porter E, Zhang Y, et al. Immunogenicity of RNA replicons encoding HIV env immunogens designed for self-assembly into nanoparticles. Mol Ther. 2019;27(12):2080–2090. doi: 10.1016/j.ymthe.2019.08.007
- Hussain A, Yang H, Zhang M, et al. mRNA vaccines for COVID-19 and diverse diseases. J Control Release. 2022;345:314–333. doi: 10.1016/j.jconrel.2022.03.032
- Schoenmaker L, Witzigmann D, Kulkarni JA, et al. mRNA-lipid nanoparticle COVID-19 vaccines: structure and stability. Int J Pharmaceut. 2021;601:120586. doi: 10.1016/j.ijpharm.2021.120586
- Hou X, Zaks T, Langer R, et al. Lipid nanoparticles for mRNA delivery. Nature Rev Mater. 2021;6(12):1078–1094. doi:10.1038/s41578-021-00358-0
- Walsh EE, Frenck RW Jr., Falsey AR, et al. Safety and immunogenicity of two RNA-Based COVID-19 vaccine candidates. N Engl J Med. 2020;383(25):2439–2450. doi: 10.1056/NEJMoa2027906
- Sahin U, Muik A, Derhovanessian E, et al. COVID-19 vaccine BNT162b1 elicits human antibody and T(H)1 T cell responses. Nature. 2020;586(7830):594–599. doi: 10.1038/s41586-020-2814-7
- Crommelin DJA, Anchordoquy TJ, Volkin DB, et al. Addressing the cold reality of mRNA vaccine stability. J Pharm Sci. 2021;110(3):997–1001. doi:10.1016/j.xphs.2020.12.006
- Baden LR, El Sahly HM, Essink B, et al. Efficacy and safety of the mRNA-1273 SARS-CoV-2 vaccine. N Engl J Med. 2021;384(5):403–416. doi: 10.1056/NEJMoa2035389
- Didierlaurent AM, Laupèze B, Di Pasquale A, et al. Adjuvant system AS01: helping to overcome the challenges of modern vaccines. Expert Rev Vaccines. 2017;16(1):55–63. doi:10.1080/14760584.2016.1213632
- Cunningham AL, Lal H, Kovac M, et al. Efficacy of the herpes zoster subunit vaccine in adults 70 years of age or older. N Engl J Med. 2016;375(11):1019–1032. doi: 10.1056/NEJMoa1603800
- Cunningham AL, Heineman TC, Lal H, et al. Immune responses to a recombinant glycoprotein E herpes zoster vaccine in adults aged 50 years or older. J Infect Dis. 2018;217(11):1750–1760. doi: 10.1093/infdis/jiy095
- Kurtovic L, Atre T, Feng G, et al. Multifunctional antibodies are induced by the RTS,S malaria vaccine and associated with protection in a phase 1/2a trial. J Infect Dis. 2021;224(7):1128–1138. doi: 10.1093/infdis/jiaa144
- Moon JE, Ockenhouse C, Regules JA, et al. A phase IIa controlled human malaria infection and immunogenicity study of RTS,S/AS01E and RTS,S/AS01B delayed fractional dose regimens in malaria-naive adults. J Infect Dis. 2020;222(10):1681–1691. doi: 10.1093/infdis/jiaa421
- Laurens MB. RTS, S/AS01 vaccine (Mosquirix™): an overview. Human Vaccines & Immunotherapeutics. 2020;16(3):480–489. doi:10.1080/21645515.2019.1669415
- Regules JA, Cicatelli SB, Bennett JW, et al. Fractional third and fourth dose of RTS,S/AS01 malaria Candidate vaccine: a phase 2a controlled human malaria parasite infection and immunogenicity study. J Infect Dis. 2016;214(5):762–771. doi: 10.1093/infdis/jiw237
- Minassian AM, Silk SE, Barrett JR, et al. Reduced blood-stage malaria growth and immune correlates in humans following RH5 vaccination. Med (N Y). 2021;2(6):701–719.e719. doi: 10.1016/j.medj.2021.03.014
- Nielsen CM, Barrett JR, Davis C, et al. Delayed boosting improves human antigen-specific ig and B cell responses to the RH5.1/AS01B malaria vaccine. JCI Insight. 2023;8(2). doi: 10.1172/jci.insight.163859
- Burny W, Callegaro A, Bechtold V, et al. Different adjuvants induce common innate pathways that are associated with enhanced adaptive responses against a model antigen in humans. Front Immunol. 2017;8:943. doi: 10.3389/fimmu.2017.00943
- De Mot L, Bechtold V, Bol V, et al. Transcriptional profiles of adjuvanted hepatitis B vaccines display variable interindividual homogeneity but a shared core signature. Sci Transl Med. 2020;12(569). doi: 10.1126/scitranslmed.aay8618
- Budroni S, Buricchi F, Cavallone A, et al. Antibody avidity, persistence, and response to antigen recall: comparison of vaccine adjuvants. NPJ Vaccines. 2021;6(1):78. doi: 10.1038/s41541-021-00337-0
- Rodriguez-Fernandez S, Pujol-Autonell I, Brianso F, et al. Phosphatidylserine-liposomes promote tolerogenic features on dendritic cells in human type 1 diabetes by Apoptotic Mimicry. Front Immunol. 2018;9:253. doi: 10.3389/fimmu.2018.00253
- Pujol-Autonell I, Serracant-Prat A, Cano-Sarabia M, et al. Use of autoantigen-loaded phosphatidylserine-liposomes to arrest autoimmunity in type 1 diabetes. PLoS One. 2015;10(6):e0127057. doi: 10.1371/journal.pone.0127057
- Miura N, Akita H, Tateshita N, et al. Modifying antigen-encapsulating liposomes with KALA facilitates MHC class I antigen presentation and enhances anti-tumor effects. Mol Ther. 2017;25(4):1003–1013. doi:10.1016/j.ymthe.2017.01.020
- Hanson MC, Abraham W, Crespo MP, et al. Liposomal vaccines incorporating molecular adjuvants and intrastructural T-cell help promote the immunogenicity of HIV membrane-proximal external region peptides. Vaccine. 2015;33(7):861–868. doi: 10.1016/j.vaccine.2014.12.045
- Watson DS, Szoka FC Jr. Role of lipid structure in the humoral immune response in mice to covalent lipid-peptides from the membrane proximal region of HIV-1 gp41. Vaccine. 2009;27(34):4672–4683. doi:10.1016/j.vaccine.2009.05.059
- Fotoran WL, Santangelo R, de Miranda BNM, et al. DNA-Loaded cationic liposomes efficiently function as a vaccine against malarial proteins. Mol Ther Methods Clin Dev. 2017;7:1–10. doi:10.1016/j.omtm.2017.08.004
- Shao S, Geng J, Ah Yi H, et al. Functionalization of cobalt porphyrin–phospholipid bilayers with his-tagged ligands and antigens. Nature Chemistry. 2015;7(5):438–446. doi: 10.1038/nchem.2236
- Torres OB, Matyas GR, Rao M, et al. Heroin-HIV-1 (H2) vaccine: induction of dual immunologic effects with a heroin hapten-conjugate and an HIV-1 envelope V2 peptide with liposomal lipid a as an adjuvant. NPJ Vaccines. 2017;2(1):13. doi: 10.1038/s41541-017-0013-9
- He X, Zhou S, Quinn B, et al. An in vivo screen to identify short peptide mimotopes with enhanced antitumor immunogenicity. Cancer Immunol Res. 2022;10(3):314–326. doi: 10.1158/2326-6066.CIR-21-0332
- Huang W-C, Deng B, Lin C, et al. A malaria vaccine adjuvant based on recombinant antigen binding to liposomes. Nature Nanotechnol. 2018;13(12):1174–1181. doi: 10.1038/s41565-018-0271-3
- He X, Zhou S, Dolan M, et al. Immunization with short peptide particles reveals a functional CD8 + T-cell neoepitope in a murine renal carcinoma model. J Immunother Cancer. 2021;9(12):e003101. doi: 10.1136/jitc-2021-003101
- Warmenhoven H, Leboux R, Bethanis A, et al. Cationic liposomes bearing Bet v 1 by coiled coil-formation are hypo-allergenic and induce strong immunogenicity in mice. Front Allergy. 2022;3:1092262. doi: 10.3389/falgy.2022.1092262
- Leboux RJT, Benne N, van Os WL, et al. High-affinity antigen association to cationic liposomes via coiled coil-forming peptides induces a strong antigen-specific CD4(+) T-cell response. Eur J Pharm Biopharm. 2021;158:96–105. doi: 10.1016/j.ejpb.2020.11.005
- Chen W, Huang L. Induction of cytotoxic T-lymphocytes and antitumor activity by a liposomal lipopeptide vaccine. Mol Pharm. 2008;5(3):464–471. doi:10.1021/mp700126c
- Huang WC, Deng B, Seffouh A, et al. Antibody response of a particle-inducing, liposome vaccine adjuvant admixed with a Pfs230 fragment. NPJ Vaccines. 2020;5(1):23. doi: 10.1038/s41541-020-0173-x
- Zhou S, KOA Y, Mabrouk MT, et al. Antibody induction in mice by liposome-displayed recombinant enterotoxigenic Escherichia coli (ETEC) colonization antigens. Biomed J. 2023. doi:10.1016/j.bj.2023.03.001
- Muhs A, Hickman DT, Pihlgren M, et al. Liposomal vaccines with conformation-specific amyloid peptide antigens define immune response and efficacy in APP transgenic mice. Proc Natl Acad Sci U S A. 2007;104(23):9810–9815. doi: 10.1073/pnas.0703137104
- Bale S, Goebrecht G, Stano A, et al. Covalent linkage of HIV-1 trimers to synthetic liposomes elicits improved B cell and antibody responses. J Virol. 2017;91(16). doi: 10.1128/JVI.00443-17
- Dubrovskaya V, Tran K, Ozorowski G, et al. Vaccination with Glycan-modified HIV NFL envelope trimer-liposomes elicits broadly neutralizing antibodies to multiple sites of vulnerability. Immunity. 2019;51(5):915–929.e917. doi: 10.1016/j.immuni.2019.10.008
- Krupka M, Masek J, Barkocziova L, et al. The position of His-tag in recombinant OspC and application of various adjuvants affects the intensity and quality of specific antibody response after immunization of experimental mice. PLoS One. 2016;11(2):e0148497. doi: 10.1371/journal.pone.0148497
- Szoka F Jr., Papahadjopoulos D. Procedure for preparation of liposomes with large internal aqueous space and high capture by reverse-phase evaporation. Proc Natl Acad Sci U S A. 1978;75(9):4194–4198. doi:10.1073/pnas.75.9.4194
- Huang H, Zhang C, Yang S, et al. The investigation of mRNA vaccines formulated in liposomes administrated in multiple routes against SARS-CoV-2. J Control Release. 2021;335:449–456. doi:10.1016/j.jconrel.2021.05.024
- Goswami R, Chatzikleanthous D, Lou G, et al. Mannosylation of LNP results in improved potency for self-amplifying RNA (SAM) vaccines. ACS Infect Dis. 2019;5(9):1546–1558. doi: 10.1021/acsinfecdis.9b00084
- Blakney AK, McKay PF, Yus BI, et al. Inside out: optimization of lipid nanoparticle formulations for exterior complexation and in vivo delivery of saRNA. Gene Ther. 2019;26(9):363–372. doi:10.1038/s41434-019-0095-2
- Goswami R, O’Hagan DT, Adamo R, et al. Conjugation of mannans to enhance the potency of liposome nanoparticles for the delivery of RNA vaccines. Pharmaceutics. 2021;13(2):240. doi: 10.3390/pharmaceutics13020240
- Guan HH, Budzynski W, Koganty RR, et al. Liposomal formulations of synthetic MUC1 peptides: effects of encapsulation versus surface display of peptides on immune responses. Bioconjugate Chem. 1998;9(4):451–458. doi: 10.1021/bc970183n
- Brgles M, Habjanec L, Halassy B, et al. Liposome fusogenicity and entrapment efficiency of antigen determine the Th1/Th2 bias of antigen-specific immune response. Vaccine. 2009;27(40):5435–5442. doi:10.1016/j.vaccine.2009.07.012
- Salotto KE, Olson WC Jr., Pollack KE, et al. A nano-enhanced vaccine for metastatic melanoma immunotherapy. Cancer Drug Resist. 2022;5(3):829–845. doi: 10.20517/cdr.2021.132
- Teplensky MH, Distler ME, Kusmierz CD, et al. Spherical nucleic acids as an infectious disease vaccine platform. Proc Natl Acad Sci U S A. 2022;119(14):e2119093119. doi: 10.1073/pnas.2119093119
- Grabowska J, Stolk DA, Nijen Twilhaar MK, et al. Liposomal nanovaccine containing α-galactosylceramide and ganglioside GM3 Stimulates robust CD8+ T cell responses via CD169+ macrophages and cDC1. Vaccines (Basel). 2021;9(1):56. doi: 10.3390/vaccines9010056
- Grabowska J, Lopez-Venegas MA, Affandi AJ, et al. CD169(+) macrophages capture and dendritic cells instruct: the interplay of the gatekeeper and the General of the immune system. Front Immunol. 2018;9:2472. doi:10.3389/fimmu.2018.02472
- Asano K, Nabeyama A, Miyake Y, et al. CD169-positive macrophages dominate antitumor immunity by crosspresenting dead cell-associated antigens. Immunity. 2011;34(1):85–95. doi: 10.1016/j.immuni.2010.12.011
- Affandi AJ, Grabowska J, Olesek K, et al. Selective tumor antigen vaccine delivery to human CD169(+) antigen-presenting cells using ganglioside-liposomes. Proc Natl Acad Sci U S A. 2020;117(44):27528–27539. doi: 10.1073/pnas.2006186117
- Nijen Twilhaar MK, Czentner L, Bouma RG, et al. Incorporation of toll-like receptor ligands and inflammasome stimuli in GM3 liposomes to induce dendritic cell maturation and T cell responses. Front Immunol. 2022;13:842241. doi: 10.3389/fimmu.2022.842241
- Krishnan L, Sad S, Patel GB, et al. The potent adjuvant activity of archaeosomes correlates to the recruitment and activation of macrophages and dendritic cells in vivo. J Immunol. 2001;166(3):1885–1893. doi:10.4049/jimmunol.166.3.1885
- Krishnan L, Sad S, Patel GB, et al. Archaeosomes induce long-term CD8+ cytotoxic T cell response to entrapped soluble protein by the exogenous cytosolic pathway, in the absence of CD4+ T cell help. J Immunol. 2000;165(9):5177–5185. doi:10.4049/jimmunol.165.9.5177
- Ansari MA, Zubair S, Mahmood A, et al. RD antigen based nanovaccine imparts long term protection by inducing memory response against experimental murine tuberculosis. PLoS One. 2011;6(8):e22889. doi: 10.1371/journal.pone.0022889
- McCluskie MJ, Deschatelets L, Krishnan L. Sulfated archaeal glycolipid archaeosomes as a safe and effective vaccine adjuvant for induction of cell-mediated immunity. Hum Vaccin Immunother. 2017;13(12):2772–2779. doi:10.1080/21645515.2017.1316912
- Stark FC, Agbayani G, Sandhu JK, et al. Simplified admix archaeal glycolipid adjuvanted vaccine and checkpoint inhibitor therapy combination enhances protection from murine melanoma. Biomedicines. 2019;7(4):91. doi: 10.3390/biomedicines7040091
- Nayerhoda R, Park D, Jones C, et al. Extended polysaccharide analysis within the liposomal encapsulation of polysaccharides system. Materials. 2020;13(15):3320. doi: 10.3390/ma13153320
- Bhalla M, Nayerhoda R, Tchalla EYI, et al. Liposomal encapsulation of polysaccharides (LEPS) as an effective vaccine strategy to protect aged hosts against S. pneumoniae infection. Front Aging. 2021;2: doi: 10.3389/fragi.2021.798868
- Ramani K, Miclea RD, Purohit VS, et al. Phosphatidylserine containing liposomes reduce immunogenicity of recombinant human factor VIII (rFVIII) in a murine model of hemophilia A**Karthik Ramani and Razvan D. Miclea contributed equally to the manuscript. J Pharmaceut sci. 2008;97(4):1386–1398. doi:10.1002/jps.21102
- Cruz LJ, Tacken PJ, Fokkink R, et al. The influence of PEG chain length and targeting moiety on antibody-mediated delivery of nanoparticle vaccines to human dendritic cells. Biomaterials. 2011;32(28):6791–6803. doi:10.1016/j.biomaterials.2011.04.082
- Tokatlian T, Kulp DW, Mutafyan AA, et al. Enhancing humoral responses against HIV envelope trimers via nanoparticle delivery with stabilized synthetic liposomes. Sci Rep. 2018;8(1):16527. doi: 10.1038/s41598-018-34853-2
- van Broekhoven CL, Altin JG. The novel chelator lipid 3(nitrilotriacetic acid)-ditetradecylamine (NTA(3)-DTDA) promotes stable binding of His-tagged proteins to liposomal membranes: potent anti-tumor responses induced by simultaneously targeting antigen, cytokine and costimulatory signals to T cells. Biochim Biophys Acta. 2005;1716(2):104–116. doi:10.1016/j.bbamem.2005.09.003
- Faham A, Altin JG. Antigen-containing liposomes engrafted with flagellin-related peptides are effective vaccines that can induce potent antitumor immunity and immunotherapeutic effect. J Immunol. 2010;185(3):1744–1754. doi:10.4049/jimmunol.1000027
- van Broekhoven CL, Parish CR, Demangel C, et al. Targeting dendritic cells with antigen-containing liposomes: a highly effective procedure for induction of antitumor immunity and for tumor immunotherapy. Cancer Res. 2004;64(12):4357–4365. doi:10.1158/0008-5472.CAN-04-0138
- Platt V, Huang Z, Cao L, et al. Influence of multivalent nitrilotriacetic acid lipid−Ligand affinity on the circulation half-life in mice of a liposome-attached His6-protein. Bioconjugate Chem. 2010;21(5):892–902. doi:10.1021/bc900448f
- Lovell JF, Baik YO, Choi SK, et al. Interim analysis from a phase 2 randomized trial of EuCorVac-19: a recombinant protein SARS-CoV-2 RBD nanoliposome vaccine. BMC Med. 2022;20(1):462. doi: 10.1186/s12916-022-02661-1
- Ghaffar KA, Marasini N, Giddam AK, et al. Liposome-based intranasal delivery of lipopeptide vaccine candidates against group a streptococcus. Acta Biomater. 2016;41:161–168. doi: 10.1016/j.actbio.2016.04.012
- Rafii MS, Sol O, Mobley WC, et al. Safety, tolerability, and immunogenicity of the ACI-24 vaccine in adults with Down syndrome: a phase 1b randomized clinical trial. JAMA Neurol. 2022;79(6):565–574. doi: 10.1001/jamaneurol.2022.0983
- Azuar A, Madge HYR, Boer JC, et al. Poly(hydrophobic amino acids) and liposomes for delivery of vaccine against group a Streptococcus. Vaccines (Basel). 2022;10(8):1212. doi: 10.3390/vaccines10081212