ABSTRACT
Introduction
Influenza B viruses (IBV) cause a significant health and economic burden annually. Due to lower antigenic drift rate, less extensive antigenic diversity, and lack of animal reservoirs, the development of highly effective universal vaccines against IBV might be in reach. Current seasonal influenza vaccines are formulated to induce antibodies against the Hemagglutinin (HA) protein, but their effectiveness is reduced by mismatch between vaccine and circulating strains.
Areas covered
Given antibodies against the Neuraminidase (NA) have been associated with protection during influenza infection, there is considerable interest in the development of NA-based influenza vaccines. This review summarizes insights into the role of NA-based immunity against IBV and highlights knowledge gaps that should be addressed to inform the design of next-generation influenza B vaccines. We discuss how antibodies recognize broadly cross-reactive epitopes on the NA and the lack of understanding of IBV NA antigenic evolution which would benefit vaccine development in the future.
Expert opinion
Demonstrating NA antibodies as correlates of protection for IBV in humans would be paramount. Determining the extent of IBV NA antigenic evolution will be informative. Finally, it will be critical to determine optimal strategies for incorporating the appropriate NA antigens in existing clinically approved vaccine formulations.
1. Introduction
Influenza B viruses (IBV) co-circulate annually with Influenza A viruses (IAV) around the world, resulting in annual epidemics that cause significant health and social-economic burden [Citation1,Citation2,Citation3]. IBV accounted for 23% of influenza cases over 31 countries between 2000 and 2008, but the frequency of IBV cases can be much higher in some seasons and countries [Citation4]. For example, during the season 2015 in Australia the proportion of IBV among overall influenza cases was 60% [Citation5]. According to the FluNet database, IBV was detected globally at 9–40% during the period from 2017 to 2022 [Citation6]. Given this global burden, IBV is included in the World Health Organization (WHO)-recommended influenza vaccine, which is considered the most effective approach of preventing influenza infection.
In contrast to IAV, IBV has a restricted host range and primarily infects humans. Although IBV outbreaks have been reported in seals [Citation7,Citation8] and domestic pigs [Citation9] these are likely reverse zoonosis events from humans and these animals are not considered reservoirs of IBV. IBVs have been grouped into two antigenically distinct lineages, named B/Victoria/87-like and B/Yamagata/88-like strains. While the two lineages co-circulated between 2000 and 2019, no B/Yamagata viruses have been isolated since March 2020, and it is believed this lineage has become extinct [Citation10,Citation11]. Due to the disappearance of B/Yamagata and the overall slower rate of antigenic drift of IBV [Citation12], in combination with the lack of an animal reservoir, it is believed that it might be possible to eradicate IBV from humans. This, however, would require a wide distribution of potent and highly efficacious universal vaccines that broadly target antigenic variants of IBV.
Currently approved influenza vaccines are either trivalent influenza vaccines (TIV) or quadrivalent influenza vaccines (QIV), targeting A/H1N1, A/H3N2 and either one or both lineages of IBV. These vaccines are designed to induce neutralizing antibodies targeting the hemagglutinin (HA), the major surface protein of influenza virus. However, mutations in HA can result in immune escape causing antigenic drift. This can reduce vaccine effectiveness when the vaccine strain does not match the circulating strain [Citation13], creating the need of updating the influenza vaccine composition annually. However, as vaccine strains are selected based on influenza surveillance months prior to the influenza vaccine season, this increases the possibility of mismatches between selected strains and circulating strains [Citation13–16]. Given the limitations faced by current HA-based vaccines, the inclusion of additional protein antigens, like the neuraminidase (NA), could provide broader immunity and protection.
2. The critical role of NA in the influenza virus life cycle
The critical role of the NA in the influenza life cycle makes it a promising target for vaccines and therapeutics. NA is the second dominant glycoprotein on the surface of IAV and IBV. The amount of NA on virion is 5- to 10-fold less than HA [Citation17]. NA is a tetrameric membrane glycoprotein that consists of four identical monomers which include a catalytic head domain, a stalk domain, a transmembrane domain, and a cytoplastic tail. While HA allows viruses to attach and penetrate host cells, NA is crucial for releasing new virions from cell surfaces by cleaving sialic acids and allowing viruses to detach from host cells [Citation18–20]. In addition, NA contributes to virus motility by cleaving sialylated decoy receptors, which allows the virus to move through the mucus layer and to reach terminal sialic acids on the surface of target cells [Citation21]. NA also plays a significant role in preventing influenza virions from aggregation, therefore promoting virus spread [Citation22]. These important functions make NA a promising candidate for the development of vaccines and antivirals. Indeed, there are multiple commercial FDA-approved NA-inhibitor drugs that have been used in clinical settings such as Oseltamivir (Tamiflu), Zanamivir (Relenza), and Peramivir (Rapivab), which work by binding in the enzymatic active site of NA and blocking its activity [Citation23–26]. However, NA mutations lead to the development of resistance to these NA inhibitors [Citation27–29]. The clinical efficacy of NA inhibitors highlights the potential benefits of NA-based interventions, such as therapeutic monoclonal antibodies or NA-based vaccines, to contribute to broad and effective protection against influenza viruses, including IBV. However, the inclusion of NA in the vaccine requires a comprehensive understanding of antibody response to NAs and antigenic evolution of the NA. To that end, this review covers evidence on the protective potential and breadth of anti-IBV-NA antibodies and NA-based vaccines, as well as the evolution of the IBV NA and vaccination strategies to harness the protective potential of NA.
3. The protective role of anti-NA antibodies during influenza infection
Anti-NA antibodies, together with anti-HA antibodies, are established correlates of protection against influenza in humans. Specifically, an association between NA-specific antibodies and reduction of virus shedding and/or disease severity has been demonstrated in several studies. Human challenge studies [Citation8,Citation30,Citation31] provided evidence that the titer of serum anti-NA antibody is inversely correlated with influenza virus titer and duration of viral shedding. Furthermore, Neuraminidase inhibition (NAI) titers were identified as a strong correlate of reduced disease severity after challenge with influenza A/H1N1 virus [Citation32]. In A/H1N1 infected adults in community settings, pre-existing anti-NA antibodies were associated with shorter durations of virus shedding and illness [Citation33,Citation34]. In the context of anti-HA antibodies, which are an established correlate of protection [Citation35], NAI were identified as an independent correlate of protection [Citation32]. Similarly, a study on immune response to NA post-immunization with inactivated influenza vaccine (IIV) and live attenuated influenza vaccine (LAIV) found that NA-specific antibodies correlated with reduced frequency of PCR-confirmed influenza independently of HAI and microneutralization titers [Citation36]. Collectively, these studies emphasize the importance of NA-specific antibodies in reducing viral load and mitigating disease severity. It is important to note that these studies were performed in the context of IAV, and additional studies are needed to dissect the protective role of NA-specific antibodies against IBV infection in humans. Nonetheless, a protective role of NA-based immunity against IBV is supported by animal studies.
4. Broad cross-protection against IBV conferred by NA-specific immunity in animal models
The potential of NA-specific immunity to confer broad cross-protection against IBV in vivo has been demonstrated in a number of studies (). Importantly, several studies demonstrated the efficacy of NA-based vaccines in protecting mice from both homologous and heterologous challenge. Indeed, cross-protection was observed when vaccination with NA antigen from B/Yamagata/88 lineage conferred protection against heterologous challenge with different strains from the B/Victoria/87 lineage [Citation37,Citation38]. The effectiveness of NA-based vaccines has also been demonstrated in a guinea pig transmission model [Citation39], where vaccine-induced mucosal immunity against the IBV NA reduced the transmission of heterologous IBV strains. Although human studies are required to confirm these observations, these results suggest that NA-based vaccines may not only confer protection against IBV disease but also potentially prevent virus spread between individuals [Citation39]. Overall, NA-based vaccines show great potential in conferring broad protection against IBV in animal models. However, if the aim of including NA in influenza vaccines is to induce antibodies with greater breadth than those targeted to the HA, then it is critical to understand how mutations in the NA contribute to immune escape and the extent of antigenic drift that NA proteins undergo. To that end, we need a comprehensive understanding of (i) how broadly cross-reactive and strain-specific antibodies recognize the IBV NA, and (ii) the extent of NA antigenic evolution and how mutations impact antibody recognition.
Table 1. Animal studies demonstrating broadly cross-protection against IBV using NA-based vaccines.
5. Monoclonal antibodies targeting the IBV NA
Several studies have isolated broadly cross-reactive monoclonal antibodies against IBV NA (). These mAbs show varying degrees of cross-reactivity spanning both IBV lineages (pan-IBV) or both IAV and IBV (pan-influenza).
Table 2. Broadly cross-reactive anti-NA antibodies against influenza B virus.
5.1. Pan-IBV-NA specific monoclonal antibodies
Five anti-NA mAbs including 1F2, 1F4, 3G1, 4B2, and 4F11 were isolated from mice with broad cross-reactivity to ancestral, B/Yamagata, and B/Victoria lineages [Citation41]. Several mAbs against IBV NA have also been isolated by single-cell sorting of plasmablasts from humans either post-infection or post-vaccination [Citation42,Citation43]. Of note, two mAbs including (1G05 and 2E01) isolated from a single IBV-infected patient, displayed broad cross-reactivity in vitro by inhibiting NA activity against B/Yamagata/16/88-like viruses, B/Victoria/2/87-like viruses, and the ancestral B/Lee/1940 strain, and could protect mice against lethal IBV infection. These mAbs also exhibited neutralizing activity and Fc-receptor-mediated effector functions [Citation42]. However, since 1G05 and 2E01 were isolated from a single donor, it is unknown how prevalent such antibodies are in the population. Another panel of human mAbs against IBV NA was identified from two donors at 7 days post-vaccination (2014–2015 seasonal quadrivalent IIV). Particularly, 1092D4, 1086C12, 1086F8, and 1122C6 mAbs showed NA inhibition activity and cross-reactivity against both B/Yamagata/16/88-like and B/Victoria/2/87-like viruses. Additionally, these mAbs protected mice from challenge with B/Brisbane/60/2008 [Citation43]. These mAbs demonstrate the existence of highly conserved sites on the IBV NA that are susceptible to NA inhibition.
5.2. Human pan-influenza anti-NA monoclonal antibodies
Two pan anti-NA mAbs including 1E01, and 1G01 were firstly reported to broadly bind to both IAV NA (N1 and N2) and IBV NA (B/Yamagata/88 and B/Victoria/87). However, the in vitro and in vivo results showed that these mAbs only inhibit NA activity of one B/Victoria/87 strain and there was no NAI activity against two strains of B/Yamagata/88 [Citation44]. DA03E17 is another pan-anti-NA mAb isolated from A/H1N1pdm09 infected individuals. This mAb broadly binds to IAV N1, IAV N2, and IBV NA to a different epitope than 1G01, and based on microneutralization assays DA03E17 displays broader cross-reactivity than 1G01. In vivo DA03E17 protected mice from lethal infection with different subtypes of IAV and B/Yamagata viruses [Citation45]. More recently, a pan-anti-NA mAb (FNI9) was isolated showing potent NAI activity against all IAV N subtypes and IBV NA. FNI9 was protective against lethal IAV and IBV challenges in vivo [Citation46]. HCA-2, an antibody targeting a highly conserved epitope of IBV NA ‘ILRTQESEC’ was generated and showed a broad inhibition against nine subtypes of IAV NA as well as both B/Yamagata-88 and B/Victoria-87 NA. Notably, HCA-2 inhibited the activity of two drug-resistant IBV strains including those with the E117D and D197E mutations. However, whether this mAb provides broad protection in in vivo models is unknown at present [Citation47,Citation48].
5.3. Epitopes of broadly inhibiting antibodies: within and beyond the enzymatic active site
The development of NA-based vaccines requires an understanding of the molecular basis of antibody recognition of the IBV NA (, ). Structural characterization of pan-IBV mAbs 1G05 and 2E01 identified several contacts between the CDR-H3 loops and the IBV NA active site, which is highly conserved between strains. Additional binding sites proximal to the active site (R147, K435, and H134) that are conserved across IBV but not IAV likely determine the range of cross-reactivity for these mAbs [Citation42]. On the other hand, other pan-IBV binding mAbs (4F11 and 3G1) bind proximally to, but outside of, the enzyme active site. Interestingly, escape mutations for these mAbs can be found distally of the enzymatic active site including E338K, G385R, Q453R, and G346R [Citation41]. This highlights a need to further understand how mutations may impact escape from antibody recognition.
Figure 1. Residues on the IBV NA responsible for recognition and/or escape from mAbs. (a) footprint of pan-IBV NA mAbs. Residues on IBV-NA-specific that have been identified as contact residues for pan-IBV mAbs based on structural analyses or through escape mutants. The enzymatic active site if shown in red for reference. (b) footprint of pan-influenza NA mAbs. Residues on IBV-NA-specific that have been identified as contact residues for pan-NA mAbs based on structural analyses or through escape mutants. Most of key pan-influenza NA contact residues are located within conserved enzymatic active site of IAV and IBV NA. A few residues are found outside enzymatic site such as T437 (escape mutant from DA03E17) and L132, R154, W177 (binding sites of 1G01 mAb). Binding sites for these mAbs have been determined from structural or mutational analyses in the context of IAV NA. The equivalent positions have been identified and are shown here for BNA. The NA structure is based on PDB: 6V4N.
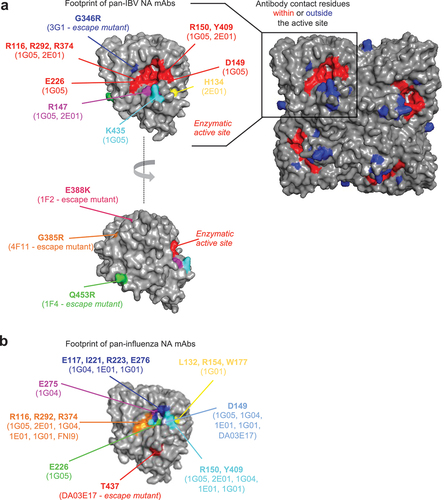
Table 3. Critical residues responsible for binding of NA to mAbs.
Epitopes of pan-NA mAbs encompass residues such as R116, E117, D149, R150, I221, R223, E275, E276, R292, R374, Y409, which are conserved between the active sites of group 1 IAV, group 2 IAV, and IBV NA. In addition, three residues responsible for 1G01 binding including L132, R154, W177 are found nearby the enzymatic active site. An emerging pattern from these analyses is that antibodies can inhibit NA activity by interacting with the highly conserved enzymatic active site in a manner similar to sialic acid receptors. This makes NA a promising target for universal vaccines.
6. Antigenic evolution of IBV NA – to what extent does IBV NA evolves to escape antibodies?
While it is well established that mutations in the IBV HA contribute to immune escape and antigenic drift, less is known about the antigenic evolution of the IBV NA. Immune escape of the IBV NA is supported by a limited number of studies on ancestral IBV isolates. Rabbit anti-sera specific for B/Lee/40, B/Taiwan/4/62 or B/Ann Arbor/1/66 showed variable degrees of cross-reactivity between the three NA antigens [Citation49]. Consistently, mAbs specific for the NA of B/Lee/40, B/HK/73 or B/Ore/80 showed variable degrees of cross-reactivity by NAI against several IBV isolates from 1940 to 1989 [Citation50]. These limited data suggest antigenic drift of the IBV NA, but additional studies are needed to determine the antigenic evolution of the IBV NA across the different lineages as well as the molecular basis of immune escape.
Sequence analysis also supports the possibility of the IBV NA undergoing antigenic drift as the NA sequences of both IAV and IBV are under diversifying selection [Citation51–56]. From the temporal phylogenies of the IBV NA genes for either B/Yamagata/88 and B/Victoria/87 lineage, mutations that become fixed in the virus population (trunk substitutions) and may have an important role in IBV evolution have been identified [Citation51]. Of these, mutations D342N, K373Q, and N342K have been previously shown to confer escape from mAbs [Citation50,Citation51]. However, the fixation of mutations in the phylogeny is not sufficient to conclude that they contribute to antigenic drift, as many of these substitutions may be antigenically neutral. Additionally, mutations in the NA may be compensating for substitutions in the HA without having effects on antibody recognition of the NA. Functional studies are therefore required to determine how mutations in the IBV NA contribute to antigenic evolution. Overall, little is known about the antigenic space of IBV NA and how mutations in the IBV NA contribute to immune escape and antigenic drift. Further studies combining serological and sequence analyses of IBV NA are needed to address this knowledge gap, which has important implication for the inclusion of NA in influenza vaccines.
7. Conclusion
To sum up, NA has been demonstrated to play a critical role in protection against both IAV and IBV. The broad cross-reaction of anti-NA antibodies suggests the potential of a next-generation NA-based vaccine. However, given the understanding about the antigenic characteristics of NA and cellular responses to NA remains limited, more studies are required to support develop a novel vaccine targeting NA.
8. Expert opinion
There is sufficient evidence that NA-based interventions can have a clinically relevant impact during influenza infections. Yet neither NA antigenic evolution nor immunogenicity are routinely considered. We highlight three key areas where additional research would benefit the development of influenza vaccines incorporating NA antigens. While these are not exclusive to IBV and will likely apply to human and possibly avian IAV, we believe IBV represents a straightforward target due to its lower rate of antigenic evolution and its, at least theoretical, potential for eradication.
First, further investigating NA antibodies as correlates of protection for IBV in humans would be paramount. This should include protective thresholds, ideally based on the assessment of different measurements of anti-NA antibodies, including binding antibodies determined by enzyme-linked immunosorbent assays (ELISA) and NA activity inhibition assays like the MUNANA/NA-Star assays and the enzyme-linked lectin assay (ELLA). Similarly, different outcomes of protection should be considered, including protection from acquisition of infection, protection from symptomatic and severe disease as well as effects on transmission. Such investigations will not only strengthen the case of incorporating NA antigens in influenza vaccines but will also aid the clinical development of vaccines including NA by defining target endpoints.
Second, further characterization of broadly conserved epitopes as well as the extent of IBV NA antigenic evolution will critically inform the design of optimal NA antigens conferring broad cross-protection. It will determine if IBV NA antigens would need to be updated, as is done for the HA, or if consensus rationally designed NA antigens could provide cross-protection. While current studies mainly investigate NA antigen targeting B cell responses, little is known about the contribution of NA-derived peptides to the CD8+ or CD4+ T cell response in humans and their protective role. Therefore, further understanding T cell responses to BNA could be a promising direction to improve the effectiveness of NA-based interventions.
Finally, and perhaps most critical, will be determining optimal strategies for incorporating the appropriate NA antigens in existing clinically approved vaccine formulations. Antibody responses to the NA following administration of current influenza vaccines are highly variable due to inconsistencies in the quantity of NA antigen in different vaccine batches [Citation57–59]. A comparison of six licensed trivalent influenza vaccines (inactivated or live-attenuated) further suggested that anti-NA antibodies were induced at different levels by each vaccine type [Citation60]. Standardizing the amount of NA in inactivated vaccines could resolve this issue. This could be achieved by the addition of recombinant NA (rNA) protein. Indeed, such rNA antigens can be used to supplement either inactivated split-virion vaccines or rHA-based vaccines (e.g. Flublok) to induced robust antibodies responses to NA without compromising HA immunogenicity [Citation61,Citation62]. An important consideration is that as the stability of NA protein affects its immunogenicity, rNA needs to be correctly folded and remain intact during manufacturing process. The inclusion of multiple tetramerization domains such as VASP, GCN4, and Tetrabrachion in NA construct have been reported to produce immunogenic recombinant NA antigens [Citation63–65]. Lipid nanoparticle-encapsulated nucleoside-modified mRNA (mRNA-LNP)-based influenza vaccines are also in development. In animal models, mRNA-LNP vaccines expressing multiple IBV antigens including HA, NA, NP, and M2 conferred broad cross-reactivity in vitro and cross-protection against different IBV strains in vivo [Citation66]. An advantage of mRNA-LNP-based vaccines is the potential to co-administer mRNA-encoding different antigens. This could facilitate easier co-administration of the HA and NA, or of multiple NA antigens if that is required to increase antigenic coverage. Success in incorporating NA antigens in influenza vaccines and the overall development of universal IBV vaccines would not only mitigate the clinical and socio-economic impact of IBV but can also pave the way for the development of broadly cross-protective vaccines for more antigenically challenging IAVs from humans and animals.
Article highlights
NA-specific antibodies are a correlate of protection from influenza disease.
NA-based vaccines are broadly protective against IBV in animal models.
Broadly cross-reactive monoclonal antibodies against the NA define universal epitopes at the enzymatic active site of NA.
Antigenic evolution of IBV NA is poorly characterized.
Various strategies exist to induce NA-specific antibodies by vaccination.
Declaration of interests
M. Koutsakos has acted as a consultant for Sanofi group of companies. The other authors have no relevant affiliations or financial involvement with any organization or entity with a financial interest in or financial conflict with the subject matter or materials discussed in the manuscript. This includes employment, consultancies, honoraria, stock ownership or options, expert testimony, grants, or patents received or pending, or royalties.
Reviewer disclosures
Peer reviewers on this manuscript have no relevant financial or other relationships to disclose.
Author contributions
THTD and MK drafted the manuscript. AKW and SK revised the manuscript.
Additional information
Funding
References
- Kosik I, Yewdell JW. Influenza a virus hemagglutinin specific antibodies interfere with virion neuraminidase activity via two distinct mechanisms. Virology. 2017;500:178–183. doi: 10.1016/j.virol.2016.10.024
- Hensen L, Kedzierska K, Koutsakos M. Innate and adaptive immunity toward influenza B viruses. Future Microbiol. 2020;15(11):1045–1058. doi: 10.2217/fmb-2019-0340
- Koutsakos M, Nguyen TH, Barclay WS, et al. Knowns and unknowns of influenza B viruses. Future Microbiol. 2016;11(1):119–135. doi: 10.2217/fmb.15.120
- Caini S, Kusznierz G, Garate VV, et al. The epidemiological signature of influenza B virus and its B/Victoria and B/Yamagata lineages in the 21st century. PLoS One. 2019;14(9):e0222381. doi: 10.1371/journal.pone.0222381
- Barr IG, Vijaykrishna D, Sullivan SG Differential age susceptibility to influenza B/Victoria lineage viruses in the 2015 Australian influenza season. Eurosurveillance. 2016;21(4):30118. doi: 10.2807/1560-7917.ES.2016.21.4.30118
- Paget J, Caini S, Del Riccio M, et al. Has influenza B/Yamagata become extinct and what implications might this have for quadrivalent influenza vaccines? Eurosurveillance. 2022;27(39):2200753. doi: 10.2807/1560-7917.ES.2022.27.39.2200753
- Osterhaus A, Rimmelzwaan G, Martina B, et al. Influenza B virus in seals. Science. 2000;288(5468):1051–1053. doi: 10.1126/science.288.5468.1051
- Ohishi K, Ninomiya A, Kida H, et al. Serological evidence of transmission of human influenza a and B viruses to Caspian seals (phoca caspica). Microbiol Immunol. 2002;46(9):639–644. doi: 10.1111/j.1348-0421.2002.tb02746.x
- Ran Z, Shen H, Lang Y, et al. Domestic pigs are susceptible to infection with influenza B viruses. J Virol. 2015;89(9):4818–4826. doi: 10.1128/JVI.00059-15
- Koutsakos M, Wheatley AK, Laurie K, et al. Influenza lineage extinction during the COVID-19 pandemic? Nature Rev Microbiol. 2021;19(12):741–742. doi: 10.1038/s41579-021-00642-4
- Dhanasekaran V, Sullivan S, Edwards KM, et al. Human seasonal influenza under COVID-19 and the potential consequences of influenza lineage elimination. Nat Commun. 2022;13(1):1721. doi: 10.1038/s41467-022-29402-5
- Bedford T, Suchard MA, Lemey P, et al. Integrating influenza antigenic dynamics with molecular evolution. Elife. 2014;3:e01914. doi:10.7554/eLife.01914
- de Jong JC, Beyer Walter E.P., Palache WE, et al. Mismatch between the 1997/1998 influenza vaccine and the major epidemic a (H3N2) virus strain as the cause of an inadequate vaccine‐induced antibody response to this strain in the elderly. J Med Virol. 2000;61(1):94–99. doi: 10.1002/(SICI)1096-9071(200005)61:1<94:AID-JMV15>3.0.CO;2-C
- Krammer F, Palese P Advances in the development of influenza virus vaccines. Nat Rev Drug Discov. 2015;14(3):167–182. doi: 10.1038/nrd4529
- JE S, PC S. Importance of antigenic composition of influenza virus vaccine in protecting against the natural disease; observations during the winter of 1947-1948. Am J Public Health Nations Health. 1949;39(3):345–355. doi: 10.2105/AJPH.39.3.345
- Payne A-M. The influenza programme of WHO. Bullet World Health Organ. 1953;8(5–6):755.
- Hutchinson EC, Charles PD, Hester SS, et al. Conserved and host-specific features of influenza virion architecture. Nat Commun. 2014;5(1):1–11. doi: 10.1038/ncomms5816
- Wang Q, Tian X, Chen X, et al. Structural basis for receptor specificity of influenza B virus hemagglutinin. Proceedings of the National Academy of Sciences. 2007;104 (43):p. 16874–16879.
- Burmeister W, Ruigrok R, Cusack S The 2.2 a resolution crystal structure of influenza B neuraminidase and its complex with sialic acid. EMBO J. 1992;11(1):49–56. doi: 10.1002/j.1460-2075.1992.tb05026.x
- Du W, de Vries E, van Kuppeveld FJ, et al. Second sialic acid‐binding site of influenza A virus neuraminidase: binding receptors for efficient release. FEBS J. 2021;288(19):5598–5612. doi: 10.1111/febs.15668
- Cohen M, Zhang X-Q, Senaati HP, et al. Influenza a penetrates host mucus by cleaving sialic acids with neuraminidase. Virol J. 2013;10(1):1–13. doi: 10.1186/1743-422X-10-321
- Rajendran M, Krammer F, McMahon M The human antibody response to the influenza virus neuraminidase following infection or vaccination. Vaccines. 2021;9(8):846. doi: 10.3390/vaccines9080846
- Tao J, Wang H, Wang W, et al. Binding mechanism of oseltamivir and influenza neuraminidase suggests perspectives for the design of new anti-influenza drugs. PLoS Comput Biol. 2022;18(7):e1010343. doi: 10.1371/journal.pcbi.1010343
- Andrews DM, Cherry PC, Humber DC, et al. Synthesis and influenza virus sialidase inhibitory activity of analogues of 4-guanidino-Neu5Ac2en (zanamivir) modified in the glycerol side-chain. Eur J Med Chem. 1999;34(7–8):563–574. doi: 10.1016/S0223-5234(00)80026-4
- Sarukhanyan E, Shanmugam TA, Dandekar T. In silico studies reveal peramivir and zanamivir as an optimal drug treatment even if H7N9 avian type influenza virus acquires further resistance. Molecules. 2022;27(18):5920. doi: 10.3390/molecules27185920
- Caceres CJ, Seibert B, Cargnin Faccin F, et al. Influenza antivirals and animal models. FEBS Open Bio. 2022;12(6):1142–1165. doi: 10.1002/2211-5463.13416
- Trebbien R, Pedersen SS, Vorborg K, et al. Development of oseltamivir and zanamivir resistance in influenza A (H1N1) pdm09 virus, Denmark, 2014. Eurosurveillance. 2017;22(3):30445. doi: 10.2807/1560-7917.ES.2017.22.3.30445
- Orozovic G, Orozovic K, Järhult JD, Tripp, R., et al. Study of oseltamivir and zanamivir resistance-related mutations in influenza viruses isolated from wild mallards in Sweden. PLoS One. 2014;9(2):e89306. doi: 10.1371/journal.pone.0089306
- Moscona A Oseltamivir resistance—disabling our influenza defenses. N Engl J Med. 2005;353(25):2633–2636. doi: 10.1056/NEJMp058291
- Couch RB, Kasel JA, Gerin JL, et al. Induction of partial immunity to influenza by a neuraminidase-specific vaccine. J Infect Dis. 1974;129(4):411–420. doi: 10.1093/infdis/129.4.411
- Murphy BR, Kasel JA, Chanock RM Association of serum anti-neuraminidase antibody with resistance to influenza in man. N Engl J Med. 1972;286(25):1329–1332. doi: 10.1056/NEJM197206222862502
- Memoli MJ, Shaw PA, Han A, Moscona, A., et al. Evaluation of antihemagglutinin and antineuraminidase antibodies as correlates of protection in an influenza A/H1N1 virus healthy human challenge model. MBio. 2016;7(2):10.1128/mBio.00417-16. doi: 10.1128/mBio.00417-16
- Maier HE, Nachbagauer R, Kuan G, et al. Pre-existing antineuraminidase antibodies are associated with shortened duration of influenza a (H1N1) pdm virus shedding and illness in naturally infected adults. Clinl Infect Dis. 2020;70(11):2290–2297. doi: 10.1093/cid/ciz639
- Couch RB, Atmar RL, Franco LM, et al. Antibody correlates and predictors of immunity to naturally occurring influenza in humans and the importance of antibody to the neuraminidase. J Infect Dis. 2013;207(6):974–981. doi: 10.1093/infdis/jis935
- Hobson D, Curry R, Beare A, et al. The role of serum haemagglutination-inhibiting antibody in protection against challenge infection with influenza A2 and B viruses. Epidemiol Infect. 1972;70(4):767–777. doi: 10.1017/S0022172400022610
- Monto AS, Petrie JG, Cross RT, et al. Antibody to influenza virus neuraminidase: an independent correlate of protection. J Infect Dis. 2015;212(8):1191–1199. doi: 10.1093/infdis/jiv195
- Johansson BE, Brett IC. Recombinant influenza B virus HA and NA antigens administered in equivalent amounts are immunogenically equivalent and induce equivalent homotypic and broader heterovariant protection in mice than conventional and live influenza vaccines. Hum Vaccines. 2008;4(6):420–424. doi: 10.4161/hv.4.6.6201
- Wohlbold TJ, Nachbagauer R, Xu H, Griffin, D E., et al. Vaccination with adjuvanted recombinant neuraminidase induces broad heterologous, but not heterosubtypic, cross-protection against influenza virus infection in mice. MBio. 2015;6(2):e02556–14. doi: 10.1128/mBio.02556-14
- McMahon M, Kirkpatrick E, Stadlbauer D, Schultz-Cherry, S., et al. Mucosal immunity against neuraminidase prevents influenza B virus transmission in Guinea Pigs. MBio. 2019;10(3):e00560–19. doi: 10.1128/mBio.00560-19
- Kim K-H, Li Z, Bhatnagar N, et al. Universal protection against influenza viruses by multi-subtype neuraminidase and M2 ectodomain virus-like particle. PLOS Pathogens. 2022;18(8):e1010755. doi: 10.1371/journal.ppat.1010755
- Wohlbold TJ, Podolsky KA, Chromikova V, et al. Broadly protective murine monoclonal antibodies against influenza B virus target highly conserved neuraminidase epitopes. Nat Microbiol. 2017;2(10):1415–1424. doi: 10.1038/s41564-017-0011-8
- Madsen A, Dai Y-N, McMahon M, et al. Human antibodies targeting influenza B virus neuraminidase active site are broadly protective. Immunity. 2020;53(4):852–863. e7. doi: 10.1016/j.immuni.2020.08.015
- Piepenbrink MS, Nogales A, Basu M, Moscona, A., et al. Broad and protective influenza B virus neuraminidase antibodies in humans after vaccination and their clonal persistence as plasma cells. MBio. 2019;10(2):e00066–19. doi: 10.1128/mBio.00066-19
- Stadlbauer D, Zhu X, McMahon M, et al. Broadly protective human antibodies that target the active site of influenza virus neuraminidase. Science. 2019;366(6464):499–504. doi: 10.1126/science.aay0678
- Yasuhara A, Yamayoshi S, Kiso M, et al. A broadly protective human monoclonal antibody targeting the sialidase activity of influenza a and B virus neuraminidases. Nat Commun. 2022;13(1):6602. doi: 10.1038/s41467-022-34521-0
- Momont C, Dang HV, Zatta F, et al. A pan-influenza antibody inhibiting neuraminidase via receptor mimicry. Nature. 2023;1–8.
- Gravel C, Li C, Wang J, et al. Qualitative and quantitative analyses of virtually all subtypes of influenza a and B viral neuraminidases using antibodies targeting the universally conserved sequences. Vaccine. 2010;28(36):5774–5784. doi: 10.1016/j.vaccine.2010.06.075
- Doyle TM, Li C, Bucher DJ, et al. A monoclonal antibody targeting a highly conserved epitope in influenza B neuraminidase provides protection against drug resistant strains. Biochem Biophys Res Commun. 2013;441(1):226–229. doi: 10.1016/j.bbrc.2013.10.041
- Curry R, Brown J, Baker F, et al. Serological studies with purified neuraminidase antigens of influenza B viruses. Epidemiol Infect. 1974;72(2):197–204. doi: 10.1017/S0022172400023408
- Air GM, Laver WG, Luo M, et al. Antigenic, sequence, and crystal variation in influenza B neuraminidase. Virology. 1990;177(2):578–587. doi: 10.1016/0042-6822(90)90523-T
- Virk RK, Jayakumar J, Mendenhall IH, et al. Divergent evolutionary trajectories of influenza B viruses underlie their contemporaneous epidemic activity. Proceedings of the National Academy of Sciences. 2020;117 (1):p. 619–628.
- Vijaykrishna D, Holmes EC, Joseph U, et al. The contrasting phylodynamics of human influenza B viruses. Elife. 2015;4:e05055. doi: 10.7554/eLife.05055
- Kilbourne ED, Johansson BE, Grajower B. Independent and disparate evolution in nature of influenza A virus hemagglutinin and neuraminidase glycoproteins. Proceedings of the National Academy of Sciences. 1990;87 (2):p. 786–790.
- Sandbulte MR, Westgeest KB, Gao J, et al. Discordant antigenic drift of neuraminidase and hemagglutinin in H1N1 and H3N2 influenza viruses. Proceedings of the National Academy of Sciences. 2011;108 (51):p. 20748–20753.
- Laver W, Air G, Webster R, et al. Amino acid sequence changes in antigenic variants of type a influenza virus N2 neuraminidase. Virology. 1982;122(2):450–460. doi: 10.1016/0042-6822(82)90244-6
- Westgeest KB, de Graaf M, Fourment M, et al. Genetic evolution of the neuraminidase of influenza a (H3N2) viruses from 1968 to 2009 and its correspondence to haemagglutinin evolution. J Gen Virol. 2012;93(Pt 9):1996. doi: 10.1099/vir.0.043059-0
- Sultana I, Yang K, Getie-Kebtie M, et al. Stability of neuraminidase in inactivated influenza vaccines. Vaccine. 2014;32(19):2225–2230. doi: 10.1016/j.vaccine.2014.01.078
- Getie‐Kebtie M, Sultana I, Eichelberger M, et al. Label‐free mass spectrometry‐based quantification of hemagglutinin and neuraminidase in influenza virus preparations and vaccines. Influenza Other Respir Viruses. 2013;7(4):521–530. doi: 10.1111/irv.12001
- Ito H, Nishimura H, Kisu T, Cao, Y., et al. Low response in eliciting neuraminidase inhibition activity of sera among recipients of a split, monovalent pandemic influenza vaccine during the 2009 pandemic. PLoS One. 2020;15(5):e0233001. doi: 10.1371/journal.pone.0233001
- Couch RB, Atmar RL, Keitel WA, et al. Randomized comparative study of the serum antihemagglutinin and antineuraminidase antibody responses to six licensed trivalent influenza vaccines. Vaccine. 2012;31(1):190–195. doi: 10.1016/j.vaccine.2012.10.065
- Johansson BE, Matthews JT, Kilbourne ED. Supplementation of conventional influenza a vaccine with purified viral neuraminidase results in a balanced and broadened immune response. Vaccine. 1998;16(9–10):1009–1015. doi: 10.1016/S0264-410X(97)00279-X
- Johansson BE, Pokorny BA, Tiso VA Supplementation of conventional trivalent influenza vaccine with purified viral N1 and N2 neuraminidases induces a balanced immune response without antigenic competition. Vaccine. 2002;20(11–12):1670–1674. doi: 10.1016/S0264-410X(01)00490-X
- Bosch BJ, Bodewes R, de Vries RP, et al. Recombinant soluble, multimeric HA and NA exhibit distinctive types of protection against pandemic swine-origin 2009 a (H1N1) influenza virus infection in ferrets. J Virol. 2010;84(19):10366–10374. doi: 10.1128/JVI.01035-10
- Schotsaert M, Ysenbaert T, Smet A, et al. Long-lasting cross-protection against influenza A by neuraminidase and M2e-based immunization strategies. Sci Rep. 2016;6(1):24402. doi: 10.1038/srep24402
- Liu W-C, Lin C-Y, Tsou Y-T, et al. Cross-reactive neuraminidase-inhibiting antibodies elicited by immunization with recombinant neuraminidase proteins of H5N1 and pandemic H1N1 influenza a viruses. J Virol. 2015;89(14):7224–7234. doi: 10.1128/JVI.00585-15
- Pardi N, Carreño JM, O’Dell G, et al. Development of a pentavalent broadly protective nucleoside-modified mRNA vaccine against influenza B viruses. Nat Commun. 2022;13(1):4677. doi: 10.1038/s41467-022-32149-8