ABSTRACT
Introduction
Malaria represents a public health challenge in tropical and subtropical regions, and currently deployed control strategies are likely insufficient to drive elimination of malaria. Development and improvement of malaria vaccines might be key to reduce disease burden. Vaccines targeting asexual blood stages of the parasite have shown limited efficacy when studied in human trials conducted over the past decades.
Areas covered
Vaccine candidates based on the merozoite surface protein 1 (MSP1) were initially envisioned as one of the most promising approaches to provide immune protection against asexual blood-stage malaria. Successful immunization studies in monkey involved the use of the full-length MSP1 (MSP1FL) as vaccine construct. Vaccines using MSP1FL for immunization have the potential benefit of including numerous conserved B-cell and T-cell epitopes. This could result in improved parasite strain-transcending, protective immunity in the field. We review outcomes of clinical trials that utilized a variety of MSP1 constructs and formulations, including MSP1FL, either alone or in combination with other antigens, in both animal models and humans.
Expert opinion
Novel approaches to analyze breadth and magnitude of effector functions of MSP1-targeting antibodies in volunteers undergoing experimental vaccination and controlled human malaria infection will help to define correlates of protective immunity.
1. Introduction
The malaria disease burden batters particularly impoverished tropical and subtropical regions of the world [Citation1]. The latest estimated number of 247 million annual malaria cases, which resulted in 619,000 deaths globally [Citation2], suggests that the control strategies implemented in the last decades [Citation3], which led to reduction in morbidity and mortality [Citation4], are insufficient for malaria elimination. This holds true especially when facing unexpected challenges, such as the recent SARS-CoV-2 pandemic [Citation5] or outbreaks of armed conflict haunting areas endemic to malaria [Citation6].
Vaccines are considered a potentially impactful, additional tool toward reducing and controlling the malaria disease burden [Citation7], yet the history of malaria vaccine development has been long and tedious. In 1967, Ruth Nussenzweig published an important breakthrough toward a first concept of a pre-erythrocytic vaccine aimed at protecting from malaria infection. She immunized mice with the rodent malaria parasite Plasmodium berghei by exposing them to sporozoites that had been attenuated by X-ray irradiation [Citation8]. This approach conferred significant sterile protection in mice against the parasite when later challenged with fully infectious P. berghei sporozoites delivered by mosquito bites. Since then, progress toward developing an effective malaria vaccine, and especially against pre-erythrocytic stages (commonly known as sporozoite and/or liver-stage vaccines), has faced many challenges [Citation9]. This is based on a number of factors including the complex biology of Plasmodium species and their well-adapted interactions with the mosquito vector and the human host [Citation10,Citation11]. Despite these obstacles, arduous efforts resulted in the development of the first generation pre-erythrocytic malaria vaccine against P. falciparum called RTS,S/AS01 [Citation9]. RTS,S/AS01 is the first licensed malaria vaccine (MosquirixTM) and is currently in the implementation phase in three African countries [Citation12]. The WHO-specified malaria vaccine efficacy goal of 75% against malaria disease in African children [Citation13] has recently been reported in a phase II clinical trial evaluating the subunit malaria vaccine candidate R21 [Citation14,Citation15]. Moreover, sterile protection against P. falciparum malaria infection has also been accomplished by attenuated, purified sporozoite vaccine approaches (PfSPZ Vaccine) [Citation16,Citation17].
In contrast to pre-erythrocytic stage vaccines, blood-stage malaria vaccines are aimed at reducing or blocking asexual reproduction of the malaria parasite in red blood cells (RBCs). Several clinical trials based on blood-stage parasite antigens of P. falciparum have been conducted but, unfortunately, as to date, no vaccine has shown strong protective efficacy in phase II field trials [Citation18,Citation19]. Thus, current efforts are focused on refining liver-stage vaccines [Citation20], while less attention is given to the development of new blood-stage candidates or to the refinement of previously tested antigens [Citation21]. Pre-erythrocytic vaccines targeting the sporozoites and/or liver stage offer the advantage that they act from the moment the limited numbers of parasites transmitted by an infected Anopheline mosquito bite enter the host until they exit the liver as merozoites and reach the blood stage. However, if these vaccines are not extremely efficient, they will not prevent potential progression of liver-stage merozoites to asexual blood-stage parasites resulting in malaria disease and potentially death. Life-threatening effects associated with P. falciparum malaria, such as severe anemia and cerebral malaria, are caused by asexual blood stages [Citation22].
We provide insight into the potential of the merozoite surface protein 1 (MSP1) to become a component of a multistage, multicomponent next-generation malaria vaccine. Development of a multistage malaria vaccine representing a combination of pre-erythrocytic subunit vaccines such as RTS’S/AS01 or R21 and asexual blood-stage proteins like MSP1 might have the potential to further enhance vaccine-induced protection against clinical disease in the field. The biology, genetic diversity, and preclinical studies of MSP1 in animal models are presented. Clinical trials in humans that have employed full length or parts of MSP1 alone or in combination with other malaria antigens and formulations are also reviewed.
2. Biology of the MSP1
In 1981, a novel P. falciparum schizont-derived protein with relative molecular mass of around 195 kDa was reported by Anthony Holder [Citation23]. Later, the gene structure and specific fragments resulting from the processing of the encoded protein, what was then called P195 protein, were described [Citation24]. This P195 protein is the most abundant protein on the merozoite surface and is today known as MSP1. MSP1 is synthesized from the onset of schizogony as a 195 kDa precursor that undergoes a series of proteolytic cleavages [Citation25,Citation26]. The first proteolytic processing step is performed by the P. falciparum subtilisin-like protease PfSUB1 at the time of merozoite egress from the host cell to yield four fragments, namely the p83, p30, p38, and p42 polypeptides [Citation27]. The multipartite MSP1 complex found on the surface of the free merozoite is shed at the time of RBC invasion in a secondary processing step performed by the subtilisin-like protease PfSUB2 [Citation28]. This step mediates the proteolytic cleavage of p42 into two further fragments, p33 and p19 (). The cleavage releases the complex containing p33 as soluble fragment, while the C-terminal, GPI-anchored p19 fragment remains on the parasite surface as the parasite enters the RBC [Citation29,Citation30]. The p19 fragment, comprised of two epidermal growth factor-like domains, is then transferred to the developing food vacuole [Citation31]. MSP1 is thought to play a key role upon the first attachment of the free merozoites to RBCs, although other components of the macromolecular complex anchored to MSP1 such as MSP6 [Citation32], MSP7 [Citation33,Citation34], MSPDBL1, and MSPDBL2 [Citation35] are also functionally involved.
Figure 1. Consecutive enzymatic processing steps of MSP1 protein. MSP1 is produced at the end of schizogony as a precursor protein of around 196 kDa. Once the merozoite reaches maturity, PfSUB1 cleaves MSP1 at specific cleavage sites (shown here in gray squares), leading to formation of four fragments named p83 (MSP1-83), p30 (MSP1-30), p38 (MSP1-38), and p42 (MSP1-42). A second cleavage event of the p42 fragment by PfSUB2 leads to the formation of fragments p33 (MSP1-33) and p19 (MSP1-19). MSP1 is divided in 17 domains [Citation37] based on sequence polymorphisms, whereby 7 domains are highly polymorphic, 5 are semi-conserved, and 5 are highly conserved which are color-marked in red, orange, and pink, respectively. MSP1 has been historically characterized by two prototypic sequences: the MSP1-D from the P. falciparum MAD20 strain and the MSP1-F from the WELLCOME strain. These two forms are found in African parasite populations, with distinct distributions observed between East and West Africa [Citation36,Citation148].
![Figure 1. Consecutive enzymatic processing steps of MSP1 protein. MSP1 is produced at the end of schizogony as a precursor protein of around 196 kDa. Once the merozoite reaches maturity, PfSUB1 cleaves MSP1 at specific cleavage sites (shown here in gray squares), leading to formation of four fragments named p83 (MSP1-83), p30 (MSP1-30), p38 (MSP1-38), and p42 (MSP1-42). A second cleavage event of the p42 fragment by PfSUB2 leads to the formation of fragments p33 (MSP1-33) and p19 (MSP1-19). MSP1 is divided in 17 domains [Citation37] based on sequence polymorphisms, whereby 7 domains are highly polymorphic, 5 are semi-conserved, and 5 are highly conserved which are color-marked in red, orange, and pink, respectively. MSP1 has been historically characterized by two prototypic sequences: the MSP1-D from the P. falciparum MAD20 strain and the MSP1-F from the WELLCOME strain. These two forms are found in African parasite populations, with distinct distributions observed between East and West Africa [Citation36,Citation148].](/cms/asset/b7f68b54-b46f-4473-b5bd-0a63e8b650a4/ierv_a_2295430_f0001_oc.jpg)
The gene msp1 (PF3D7_0930300) is located on chromosome 9 of the P. falciparum 3D7 reference genome, between the genomic positions 1,201,305–1,207,576. It is a single-copy gene with no introns within its coding region (coordinates 1,201,812–1,206,974) and is divided into 17 blocks with varying levels of genetic diversity, where 7 blocks are highly polymorphic, 5 blocks are semi-conserved, and 5 blocks are highly conserved [Citation37–40]. Evident from its name, msp1 expression is highest during the asexual blood stage of the parasite (). msp1 block 2 undergoes frequent reorganization from slipped strand mispairing [Citation41,Citation42] and possibly intragenic recombination [Citation38,Citation40,Citation43]. Differences in fragment size and the presence of unique peptide repeats [Citation44] delineate three major block 2 families: RO33, K1, and MAD20. Among these families, K1 and MAD20 alleles are the most diverse since they have varying numbers of unique tri-peptide repeats. RO33 alleles lack these tri-peptide repeats and are monomorphic. K1 and MAD20 alleles are observed at greater frequency globally than RO33 [Citation45–49]. Due to its variable genomic sequence [Citation50], genotyping of P. falciparum infections by molecular characterization of msp1 can differentiate between re-infection and recrudescent infections [Citation51,Citation52] and illuminate genetic diversity and sub-structure in parasite populations [Citation45–47,Citation49,Citation53]. However, also due to regions of high variability in sequence and the presence of insertions/deletions (indels), comprehensive locus genotyping by read mapping of whole-genome sequencing data against a reference genome becomes challenging.
Figure 2. Genetic diversity of MSP1 field isolates. (A) UMAP single cell expression profile of the merozoite surface protein gene PF3D7_0930300; https://www.sanger.ac.uk/tool/mca/mca/. Each dot represents a cell. The four quadrants represent the transcriptomes of sporozoites (salivary glands, top left quadrant), sporozoites (hemolymph, lower left quadrant), and ookinetes (lower right quadrant). The top right quadrant, containing the cells in which MSP1 is most highly expressed, is asexual blood-stage parasites. (B) Geographic origin of P. falciparum strains from which MSP1 sequences were obtained and frequency of representative alleles based on block 2 classification. Lower rows (in gray) are stratified African samples according to WHO regional delineations. (C) Principal component analysis (PCA) of msp1 sequences aligned with MUSCLE in MegaX v10.0.5. PCA values were calculated using multi-sequence alignment with Rv.4.1.3 library adegenet.
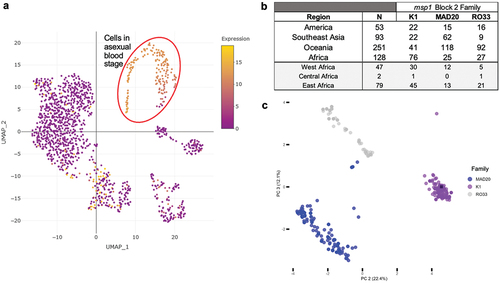
To overcome the challenge of genotyping by read mapping, 37 publicly available or reported P. falciparum whole-genome assemblies built from long read sequencing data [Citation54,Citation55] for strains collected from different continents (South East Asia (SEA) = 13; America = 3; Africa = 21) were used to obtain the msp1 coding region (CDS) and conduct a preliminary analysis of CDS sequence diversity. The average CDS length across all strains was 5092 bp (range: 4884–5166 bp) and nucleotide diversity across the entire. Further, 488 publically available, full-length, msp1 sequences from P. falciparum were downloaded from National Center for Biotechnology Information (NCBI) [Citation39,Citation56–59]. Among all sequences analyzed (n = 525), the average CDS length was 5095 bp (range: 4884–5220 bp), and nucleotide diversity across the entire CDS was relatively low (πnucleotide per 100 sites = 1.7%; 95% CI 1.5–1.9) despite only 80% of the nucleotide positions being conserved (nconserved_sites = 4168). The proportion of each msp1 block 2 family was consistent with global results obtained from Polymerase Chain Reaction (PCR)-generated allele fragments [Citation45–49], where most strains were classified as K1 (n = 161) or MAD20 (n = 220) ( and Supp. Table S1). Across block 2 family types, K1 and MAD20 were observed to have longer sequences on average (K1: 5121 average bp length, range = 4884–5220 bp; MAD20: 5094 average bp length, range = 4484–5172 bp), and RO33 had the shortest average sequence length (RO33: 5068 average bp length, range = 5043–5085 bp). A principal component analysis of the msp1 sequences shows them clustered according to sequence length and block 2 family designation, illustrating that sequence variance in the dataset is driven in large part by block 2 ( and Supp. Fig 1 and 2). PC1 (not shown) accounted for 40% of variance in the sample set, driven by a small subset (n = 10) of the shortest K1 and MAD20 sequences. Variation among all sequences is clearer in PC2 and PC3, where those shorter sequences are still observed as separate from their respective, more frequent, block 2 family clusters. The MAD20 sequences separated from their larger cluster have a larger number of SNPs in downstream msp1 blocks (Supp. Fig XX). Previous analyses of msp1 sequence diversity have shown sequence length polymorphism in block 2 as key to msp1 variation among P. falciparum strains, consistent with our results [Citation42,Citation60,Citation61]. Interestingly, most African strains encode K1 variants, while Southeast Asian and Oceania strains encode primarily MAD20 variants. This geographical differentiation is tentative due to the temporal and study differences among analyzed strains.
3. Naturally acquired humoral immune responses against MSP1
Immuno-epidemiological studies have shown that residents from malaria-endemic areas, after repeated exposure to P. falciparum infections, are able to attain strain-transcending immunity, allowing for partial protection against clinical disease [Citation64]. One of the key mediators of this acquired immunity seems to be the presence of antibodies targeting asexual blood-stage parasites [Citation65]. Antibodies against MSP1 have been often, but not always, correlated with protection against clinical malaria [Citation66–71]. Contradicting findings might be partially attributed to the organization of the protein, consisting of an alternation between conserved and more polymorphic regions (). The early focus of research was set on understanding antibody responses against different subunits of MSP172. MSP1-specific antibody responses in Mali were found to be predominantly directed against the dimorphic parts of MSP1 [Citation72].
One important aspect to clarify was whether antibodies against specific regions, and especially against dimorphic regions of MSP1, have a preponderant role in naturally acquired protection. Using sera from individuals residing in malaria-endemic regions of West Africa, as well as sera from German patients with acute malaria, a correlation between antibodies targeting specific MSP1 regions and a protective immune response against P. falciparum when stratified according to age and parasitemia levels was observed [Citation73]. For example, serum samples from adults were more reactive against the dimorphic F5 region of the K1 strain (), which is located near the enzymatic processing site between p83 and p30, several months after recovering from an infection when compared to children [Citation73]. Another study in Mali led to more ambiguous results in that no correlation between MSP1 antibody titers and protection against infection was observed in children. Yet, in adolescents, the presence of antibodies to dimorphic fragment M6, pertaining to the N-terminal p83 subunit of MSP1 (MSP1-83) (from MAD20 isolate) correlated with a 50% reduced risk of P. falciparum infection and increased ability to control parasitemia [Citation74]. In contrast, in adults living in the same region, the humoral immune response to some of the MSP1 polymorphic regions was associated with an increased risk of infection [Citation74].
Figure 3. Overview of MSP1-based vaccine constructs evaluated in preclinical and early phase clinical development studies. Arrows point at the MSP1 subunit or epitopes within the subunit contained in the vaccine candidate. Highlighted: block 2, dimorphic fragments M6 (amino acids 671–833 from the K1 strain in yellow) and F5 (amino acids 384–595 from the MAD20 strain in orange).
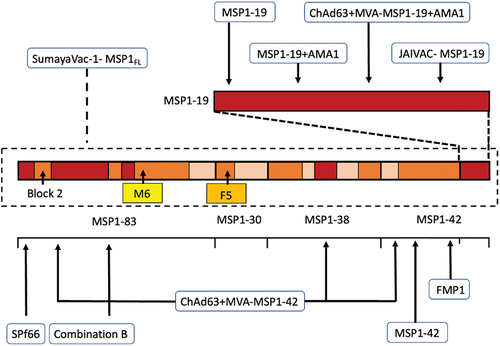
In Papua New Guinea, positive correlations of antibody titers against the partially dimorphic C-terminal MSP1-42 (including the conserved MSP1-19) with protection against clinical malaria and severe parasitemia in children were observed [Citation66]. Interestingly, responses to conserved regions such as the C-terminal MSP1-19 subunit only [Citation67] were also shown to correlate with protection when analyzing serum antibodies from Gambia and Sierra Leone in an enzyme-linked immunosorbent assay (ELISA). A systematic review with meta-analysis on the relationship between anti-merozoite antibodies and incidence of P. falciparum malaria corroborated that one of the largest protective effects came from IgG against the conserved MSP1-19 [Citation75]. These findings supported the research shift from focusing mainly on dimorphic regions to also considering conserved regions of the protein.
In The Gambia, the reactivity of antibodies from children aged 3–7 years against the MSP1 block 2 (harbored within the N-terminal MSP1-83 subunit) was tested [Citation76]. MSP1 block 2 is the locus with the lowest inter-population variance in allele frequencies in different regions in Africa and Southeast Asia, indicating immune selection in this part of MSP1 [Citation77]. Antibodies to MSP1 block 2 were strongly associated with protection from P. falciparum malaria [Citation76]. Similar results were found when analyzing children from Ghana, with antibodies to MSP1 block 2 being significantly associated with a reduced risk of subsequent clinical malaria [Citation78]. Following data corroborated that the MSP1-83 subunit in the N-terminal end of the protein is a major target of opsonizing antibodies acquired during natural exposure to malaria [Citation79]. In summary, several immune-epidemiological studies suggested that antibodies against C-terminal MSP1-42 subunit, but also to the N-terminal MSP1-83 subunit of MSP1 (both harboring dimorphic and conserved regions), may be relevant to mount a protective humoral response. Yet, despite some initial interest [Citation80–83], the N-terminal part of the protein has not been the focus as a component of an immunization strategy in humans, until recently [Citation84]. Recent findings suggest that MSP1-specific humoral immunity undergoes a progressive maturation process from infants to adults characterized by an augmentation in both quantities but also functional potency as individuals age. This is particularly pronounced in the context of blood-stage antibody-dependent neutrophil functionality [Citation85], which underscores specific antibody Fc-effector profiles correlating with the ability to control malaria.
A common, shared high-throughput immunomonitoring platform using well-defined reagents and read-outs might help in future to better understand the association between anti-MSP1 antibodies in serum samples tested and clinical malaria in different age groups residing in a range of malaria-endemic countries. The availability of ever increasing data on the parasite genotypes circulating in different regions of sub-Saharan Africa will help to improve and adapt these immunomonitoring platforms further by using reagents reflecting the parasite populations better than prime MSP1-specific immunity to be measured.
4. Experimental vaccine studies in non-human primates
Initial experiments using animal models in 1984 consisted in the immunization of Saimiri sciureus monkeys with the parasite-purified full-length MSP1 (MSP1FL) protein followed by a subsequent intravenous challenge with infected red blood cells (iRBCs) [Citation86]. All immunized monkeys self-resolved the infection following a peak parasitemia of ~4%. However, results were not fully conclusive as two out of four monkeys from the control group spontaneously cleared the infection as well (although their peak parasitemia was much higher at ~11%) [Citation86]. Shortly after, a side-by-side comparison on the protective effect of an immunization with MSP1FL versus rhoptry proteins was performed [Citation87]. Immunization with MSP1FL completely protected Aotus lemurinus griseimembra monkeys against P. falciparum malaria. Monkeys were immunized either with MSP1FL and subunits or with rhoptry proteins (n = 3) from the Uganda Palo Alto Knob + strain (FUP) versus controls and later challenged with a lethal infection of homologous P. falciparum FUP strain 3 weeks after last immunization. No patent parasitemia was detected in all monkeys immunized with MSP1, but only one out of three monkeys immunized with rhoptry proteins was partially protected [Citation87].
Supported by immune-epidemiological data pointing at conserved regions as potentially protection-mediating portions of MSP1, a recombinant vaccine construct was created [Citation88]. It contained two conserved regions of MSP1 involving the N-terminal amino acids 146–312 from MSP1-83, together with amino acids 1059–1196 from the MSP1-38 subunit, covering ~20% of the total length of MSP1. The vaccine was used to immunize Aotus trivirgatus griseimembra monkeys resulting in two out of five monkeys being protected against iRBC challenge using the highly virulent P. falciparum Vietnam Oak-Knoll (FVO) strain. Control animals immunized with recombinant P. falciparum aldolase were not protected. Yet, intriguingly, B-cell and T-cell responses were reported to be comparable in protected versus non-protected animals [Citation88].
To further proof the capacity of conserved regions of MSP1 to induce protective immunity in the monkey model, Etlinger et al. [Citation89] used the Saimiri non-human primate model. Monkeys were immunized either with native MSP1FL protein or with the so-called ‘recombinant protein 1’ of MSP1 (representing the N-terminal amino acids 147–321 from the MSP1-83 subunit) belonging to the K1 strain. The subsequent intravenous challenge was performed with iRBC from the Palo Alto strain expressing the MAD20 sequence (heterologous challenge). Whilst all control monkeys required drug treatment, half of the monkeys immunized with ‘recombinant protein 1’ were protected. Of interest, two out of three Saimiri animals immunized with native MSP1FL cleared the heterologous infection [Citation89]. The third monkey was removed from the study 8 days after challenge and, although speculative, could likely be protected as well. All control monkeys required malaria drug therapy 12 days after challenge. These data suggested that the conserved regions from the N-terminal end of MSP1 may be important for heterologous protection in the Saimiri monkey model and that the best results in terms of protection were obtained by using MSPFL protein for immunization.
The development of the synthetic peptide-based vaccine named SPf66 by Manuel Patarroyo involved immunizing wild-caught Aotus trivirgatus monkeys with merozoite-derived proteins, followed by challenging the monkeys with the asexual blood stages of the P. falciparum FVO strain [Citation90,Citation91]. The proteins that provided some levels of protection were partially sequenced, and synthetic peptides were created from these sequences to immunize additional monkeys [Citation92]. The most promising peptides were merged, and the most potent combination was synthesized as a single hybrid polypeptide which was reported to incorporate amino acids from 35 kDa, 55 kDa, and 83 kDa proteins linked by an amino acid sequence reproducing one repeat region of the Circumsporozoite Protein (CSP). The amino acid sequence of the 83 kDa peptide included in the SPf66 vaccine was demonstrated to be derived from the N-terminal end of MSP1 (MSP1-83). Among the group of six monkeys immunized with the three peptides comprising SPf66, three monkeys did not exhibit complete protection and experienced mild parasitemia reaching a maximum of 5%. Parasitemia peaked 10–15 days later compared to the control group, but the monkeys eventually underwent spontaneous recovery. Conversely, the remaining three monkeys in the same group demonstrated no signs of disease, and no parasites were detected in their blood smear samples up to 180 days of follow-up [Citation90]. Later, SPf66 from two different sources of production (Colombia versus USA) was tested in Aotus nancymai, and results underlined that the 83 kDa peptide was one of the less immunogenic of the components of the vaccine [Citation93]. Two separate research teams were later unable to replicate these findings [Citation93,Citation94], but the vaccine proceeded further with human efficacy trials in Latin America, sub-Saharan Africa, and Asia [Citation95–105].
Following experiments in monkeys focused on protein subunits, especially on the C-terminal fragment of MSP1. This was based on immune-epidemiological studies pointing at MSP1-42 and MSP1-19 as immunodominant subunits associated with reduced clinical malaria incidence. Directing the induced immune responses to a specified, immunodominant region would enhance protective responses and might avoid immune evasion mechanisms of the parasite. Moreover, the expression of full-length Plasmodium proteins poses technical challenges due to their intricate structural conformations and substantial size. Thus, by shifting from MSP1FL to subunits of MSP1, the large-scale antigen production under good manufacturing practice became feasible. Immunization of Aotus nancymai and Aotus vociferans monkeys with either recombinant MSP1-42 or MSP1-19 led to distinct protection outcomes [Citation106]. Monkeys immunized with the partially dimorphic MSP1-42 using Freund’s adjuvant and later challenged with lethal FVO strain of P. falciparum required treatment similarly to the control monkeys. In contrast, monkeys immunized with the conserved MSP1-19 subunit self-resolved an otherwise lethal infection [Citation106]. Unexpectedly, sera from the protected animals had no effect on in vitro invasion of P. falciparum into RBCs, questioning the dogma considering invasion-blocking antibodies as the main contributors to protection [Citation106]. Yet, opposite results, this time in favor of the role of invasion blocking mechanism in protection, were reported a decade later when Aotus nancymai monkeys immunized with recombinant MSP1-42 were protected against the P. falciparum FVO strain. Protection strongly correlated with the anti-MSP1-42 antibody titer and with in vitro growth inhibition activity (GIA) [Citation107,Citation108]. These findings definitively promoted the combination of ELISA and GIA as key immune-monitoring assays to predict protective immunity induced by blood-stage vaccines in human clinical trials. More recently, a chimeric recombinant PfMSP1/8 vaccine fusing MSP1-19 together with the N-terminus of P. falciparum merozoite surface protein 8 (MSP8), lacking its low-complexity Asn/Asp-rich domain, was explored [Citation109,Citation110]. As found in previous mice experimentation [Citation111,Citation112], the vaccine elicited antibody responses to both, MSP8 and the conserved MSP1-19 domains in Aotus nancymai. The antibodies generated showed significant cross-reactivity between the FVO and 3D7 alleles of MSP1 and exhibited strong in vitro parasite growth inhibitory activity [Citation109,Citation110].
Immunization of Rhesus macaques with the polymorphic MSP1 block 2 construct (the MSP1 hybrid antigen) elicited antibodies against epitopes shared between all three block two serotypes, namely K1, MAD20 and RO33 types, but IgG was found to be non-inhibitory in the GIA [Citation113]. Subsequent immunization of Aotus lemurinus griseimembra with the same construct, followed by a challenge with P. falciparum FVO strain, resulted in 50% of the immunized monkeys protected. Protected animals had four times higher parasite-specific serum antibody titers and different antibody epitope specificities compared to unprotected monkeys [Citation114]. In summary, experimental immunization experiments in monkey animal models suggested that combining conserved domains of both the N- and the C-terminal regions of MSP1, or alternatively using the entire MSP1FL for immunization, promoted higher protection levels against asexual blood-stage challenge. These findings essentially corroborated results obtained from immune-epidemiological studies mentioned above.
5. MSP1-based vaccination studies in human populations
MSP1 has undergone a series of clinical trials in different populations, age groups, dosing, and formulations (, Supp Table 2). An overview of the MSP1 fragments tested in these clinical trials is provided in .
Table 1. Subunit and viral vectored MSP1 vaccine constructs evaluated in clinical trials.
The SPf66 vaccine, which includes amino acid sequences from a conserved region of MSP1-83, was the first malaria vaccine to be evaluated in a phase III study. SPf66 was evaluated extensively for safety, immunogenicity, and efficacy in Colombia [Citation95–97], Ecuador [Citation98], Venezuela [Citation99], Tanzania [Citation100–102], The Gambia [Citation103], Thailand [Citation104], and Brazil [Citation105]. Despite a reported estimated vaccine efficacy of 31% (with a 95% confidence interval of 0–52%) [Citation101], overall conclusions of these trials suggested that SPf66 vaccine-induced immunity did not mediate malaria protection [Citation115]. Of interest for this manuscript, knowledge of the detailed immune responses that were induced against the MSP1-83 vaccine component in SPf66-vaccinated individuals remained unclear. Amador et al. [Citation95] reported that sera from vaccines with high antibody titers recognized the 83 kDa protein. However, immune responses against the different components of this synthetic construct have not been described in greater detail [Citation78–88,Citation116,Citation117].
A phase I vaccine trial investigated the safety and immunogenicity of a vaccine candidate called Combination B [Citation80]. This vaccine combined an N-terminal block 3 conserved fragment of MSP1 (190LCS.T3), the full-length MSP2 protein, and a part of the ring-infected erythrocyte surface antigen (RESA) formulated in Montanide ISA720 adjuvant and was tested first in Australian malaria-naïve volunteers. The results of the trial showed a strong T-cell response triggered by the MSP1 fragment and RESA, but generally, the antibody responses were weak. No protection against the P. falciparum 3D7 iRBC challenge 4 weeks after immunization was observed [Citation82]. The same vaccine was later explored in a malaria pre-exposed population from Papua New Guinea with similar results [Citation83]. The study suggested that MSP1 was not the component accounting for the observed 62% reduction in parasitemia triggered by Combination B vaccination in the phase I–IIb trial [Citation83].
Another phase I trial evaluated an alum-adsorbed vaccine containing MSP1-19 subunit from either the P. falciparum 3D7 or FVO strain fused to tetanus toxoid T-helper epitopes P30 and P2 in healthy adults [Citation118]. Both vaccines were immunogenic with serum antibody responses increasing with the dose administered. Yet, modifications to the formulation were suggested to be necessary to improve safety and immunogenicity profiles. The work of the MSP1 Malaria Vaccine Working Group focussed on the development of a MSP1 vaccine called ‘falciparum malaria protein 1’ (FMP1) derived from the MSP1-42 fragment (3D7 strain) using AS02A as adjuvant [Citation119]. A phase I trial demonstrated the vaccine to be safe and immunogenic when tested in malaria pre-exposed residents from western Kenya. The elicited antibodies showed GIA activity in vitro. Cellular immunity was induced as shown by T-cell proliferation and Enzyme-Linked Immunosorbent Spot (ELISPOT) responses [Citation120]. Thus, this vaccine moved ahead for testing in children aged 12–47 months [Citation121] showing comparable outcomes to adults and with a statistically significant effect of dosage level on immune responses. The FMP1 was further tested under a seasonal malaria setting by the Mali FMPI Working Group. FMP1 was also found to be highly immunogenic in Malian adults (n = 40) exposed to intense seasonal malaria transmission, with elicited immune responses binding to genetically diverse parasite clones [Citation122]. The levels of anti-MSP1-42 antibodies exhibited a seasonal pattern that was significantly enhanced and prolonged by the vaccine. Yet, despite being one of the MSP1 candidates with a longer history in human malaria clinical vaccine testing, the vaccine did not progress further [Citation123]. The reason was that in a phase IIb trial in Kenya including children that received three immunizations, and fully completing the follow-up period, the overall vaccine efficacy was 5.1% (95% CI: −26% to +28%), and thus, further development was halted [Citation123] .
To further assess safety and immunogenicity of two additional MSP1-based vaccine candidates encompassing the C-terminal MSP1-42 subunit (FVO vs 3D7 strains) and formulated with Alhydrogel, a phase I clinical trial enrolling malaria-naïve volunteers from Kansas (USA) was conducted [Citation124]. Both vaccines were regarded as safe and well tolerated, but they did not generate a significant functional humoral immune response in vitro when tested by GIA. To improve the vaccine effectiveness, the addition of other immunostimulants to the formulation was proposed. Ellis et al. [Citation125] conducted a phase I study with a vaccine formulation of Alhydrogel complemented with the adjuvant CPG 7909. Following the third round of immunization, an in vitro GIA was performed. The level of inhibition observed was dependent on the antibody titer, with slightly higher inhibition (14%, range 3–32%) in the CPG 7909 group compared to the non-CPG 7909 group [Citation125].
Other vaccine delivery approaches were developed over time to induce stronger cellular immune responses. A prime-boost approach was followed using the chimpanzee adenovirus 63 (ChAd63) and Modified Vaccinia Ankara (MVA) as delivery vectors including all conserved regions of MSP1 plus the MSP1-42 subunit from the 3D7 and WELLCOME strain [Citation126]. Sixteen malaria-naïve volunteers were immunized, and a strong CD4+ and CD8+ T-cell response was induced [Citation126]. MSP1-specific antibody responses were induced binding as well to native parasites. Antibodies could not inhibit P. falciparum growth in vitro, and it was speculated that antibody titers were not high enough to mediate growth inhibition, although other antibody functionalities were not tested. Possibly, with the intention of strengthening the ability to block RBC invasion by vaccine-elicited antibodies, a recombinant fusion of the domain III of P. falciparum Apical Membrane Antigen 1 (AMA1) together with MSP1-19 was produced, combined with adjuvant Montanide ISA 720 and tested in a phase Ia trial [Citation127]. The vaccine called PfCP2.9/Montanide ISA 720 was evaluated in healthy Chinese malaria-naïve volunteers. The high antibody titers measured to the PfCP2.9 immunogen did not result in in vitro inhibition of parasite growth, and there was limited recognition of cultivated asexual blood-stage parasites in an immunofluorescence assay [Citation127]. Later, the same antigenic combination MSP1-AMA1, although not including the conserved regions (as in [Citation126]), and delivered by ChAd63 and MVA in a prime-boost approach, was tested in phase Ia trial involving malaria-naïve volunteers [Citation128,Citation129] and phase IIa trial involving naturally immune Kenyan adults [Citation128,Citation129]. As immunological read out ELISA antibody titers, GIA, IgG avidity, and isotypes were measured. The moderate in vitro GIA observed was mainly attributed to responses binding to AMA1 (>20% GIA activity). The phase IIa trial called VAC039 showed limited protection against a natural mosquito bite challenge [Citation128]. Only one out of 38 volunteers showed protection, although some non-protected volunteers presented a longer parasite pre-patent period. Induction of cellular immunity against MSP1 and AMA1 did not appear to impact parasite growth rates in vivo [Citation128].
Safety and protective efficacy of the vaccine candidate JAIVAC-1 were also assessed in healthy Indian males aged 18–45 years in a phase Ia trial [Citation130]. JAIVAC-1 is composed of two recombinant proteins formulated with adjuvant Montanide ISA720, namely MSP1-19 and PfF2, representing the amino-terminal, conserved, cysteine-rich region of EBA-175 (with receptor-binding sites for glycophorin A in RBCs). All subjects seroconverted for PfF2, but the immune response to MSP1-19 was poor. A dose–response relationship was observed between the vaccine dose of PfF2 and antibody responses, but the vaccine failed to elicit significant antibody responses against MSP1-19130.
The latest phase I clinical study evaluating MSP1 took place between 2017 and 2018 in malaria-naïve volunteers. For the first time in humans, the safety and immunogenicity of MSP1FL formulated together with the potent Toll-Like Receptor-4 (TLR4) agonist glucopyranosyl lipid A in an oil-in-water nano-emulsion (GLA-SE) as adjuvant, named SumayaVac-1 was tested [Citation84]. The main difference of the MSP1FL protein included in SumayaVac-1 compared to the MSP1FL tested in monkeys is that while Saimiri or Aotus monkeys were immunized with MSP1FL isolated from parasites [Citation86,Citation87,Citation89,Citation131], SumayaVac-1 is based on MSP1FL produced recombinantly in E. coli by a codon optimization of the msp-1 gene to reduce the AT content. SumayaVac-1 uses pZE expression plasmids [Citation132] to separately express two halves of the MSP1 protein (p83/30 and p38/42) that are refolded together at a later step to reconstitute the full-length protein, structurally resembling the native MSP1 protein [Citation133,Citation134]. In this dose escalation, phase Ia clinical trial, the vaccine was safe and all malaria-naïve vaccinees sero-converted independent of the vaccine dose applied. High MSP1-specific antibody titers of IgG and IgM isotype peaked at 4 weeks after last vaccination, exceeding the antibody level seen in semi-immune populations from Kenya [Citation84].
6. Humoral immunity against MSP1 and antibody effector function
Antibody-mediated effector functions against merozoites have proven to be highly correlating with protection against clinical disease [Citation135–141]. Fc receptor-dependent functionality is the result of interaction of antibody-opsonized merozoites with the complement system [Citation136,Citation137], as well as with a variety of circulating immune effector cells, namely, neutrophils [Citation135,Citation142,Citation143], monocytes [Citation138,Citation139], and Natural Killer (NK) cells [Citation141]. In a controlled human malaria infection study in Kenya, the breadth of Fc-mediated effector functions against merozoites, but not GIA, delineated grades of clinical manifestations and parasite replication rates [Citation144]. Importantly, effector functions against MSP1FL recapitulated the results achieved when these effector mechanisms were tested against purified merozoites, indicating that MSP1FL is indeed a key target of naturally acquired opsonizing antibodies [Citation145]. To the best of our knowledge, serum samples collected neither in MSP1 trials in monkeys nor in humans have tested for Fc-mediated functionality except recently [Citation84,Citation146]. In the SumayaVac-1 trial (EudraCT 2016–002463-33), antibodies from malaria-naïve vaccinees promoted activation of the classical complement pathway, opsonic phagocytosis by both neutrophils and monocytes, as well as the production of IFNγ and degranulation of NK cells. These multi-functional antibodies were sustained above baseline level months after immunization [Citation146]. Interestingly, antibodies against both, the C-terminal MSP1-42 and the N-terminal MSP1-83, displayed the highest levels of opsonization-mediated functions. The use of MSP1FL could aid in the design of future MSP1-based vaccine constructs through systematic identification of epitopes that are targets of functional, protective antibodies when assessed in Controlled Human Malaria Infection (CHMI) studies in malaria pre-exposed volunteers. However, MSP1FL could divert the immune response leading to insufficient achievement of functional antibody titers, a possibility that will be explored in upcoming studies. MSP1-83 harbors the highly conserved MSP1 blocks 1, 3, and 5, of great importance for strain-transcending immunity [Citation147]. The two prototypic MSP1 sequences, namely MSP1-D and MSP1-F, are recognized by antibodies produced after immunization with MSP1FL and antibody-mediated effector functions showed cross-strain variability [Citation84]. This is of paramount interest for trials in African populations exposed to a variety of parasite strains [Citation84,Citation148].
7. Conclusion
For MSP1, as for other malaria blood-stage vaccine candidates, the key mechanism mediating immune protection has been attributed to elucidation of high titers of antibodies capable of inhibiting in a Fab-mediated manner merozoite invasion into RBCs [Citation67,Citation149]. Thus, the first in vitro surrogate of blood-stage vaccine efficacy has been testing of serum antibodies for mediation of GIA. This is the case for the most advanced blood-stage vaccine candidate PfRH5 [Citation150] that leads to induction of high levels of parasite neutralizing serum antibodies in the GIA [Citation151–153], although no sterile protection was found in a recently conducted phase I trial followed by CHMI [Citation154].
Immune-epidemiological data regarding MSP1 immunity suggest that both the N- and C-terminal ends of MSP1 play an important role in protection. The technical possibility of Good Manufacturing Practices (GMP) production of MSP1FL preserving the natural protein conformation of both the N- and C-terminal end will be essential for generating protective humoral immune responses based on growth inhibitory antibodies or Fc-mediated effector functions [Citation146]. The MSP1 structure of the Sumaya-Vac1 has recently been re-solved [Citation133], highlighting that MSP1 forms dimers in a concentration-dependent manner and that dimerization is affected by the presence of the erythrocyte cytoskeleton protein spectrin [Citation133].
Conserved regions of MSP1-19 and MSP1-83 were included in an MSPFL vaccine construct, which was proven to be highly protective in monkey trials. Recently, antibodies elicited by MSPFL vaccination in malaria-naïve humans were shown to promote a wide range of antibody Fc-dependent effector function in vitro. The availability of a well-characterized CHMI model in adult African populations will allow as next step to dissect the correlation between the breath of antibody effector functions induced by MSP1FL vaccination, their interaction with preexisting MSP1-specific immunity, and impact on asexual blood-stage parasite growth rates and pre-patent period under highly controlled conditions.
Testing of MSP1FL in humans has also the potential to significantly improve the development of future potentially preventive or even therapeutic anti-MSP1 monoclonal antibodies (mAbs) isolated from vaccinated and CHMI protected volunteers similar to already established antibodies against CSP [Citation155,Citation156]. Some well-described mAbs against MSP1 are known to react with epitopes on the MSP1-42 and MSP1-19 C-terminal processing fragments and to recognize the first growth factor-like domain of MSP1 [Citation157,Citation158]. Yet, not all mAbs have been characterized as inhibitory and some of them deploy an interfering or blocking activity [Citation159,Citation160]. Of interest, some mAbs able to inhibit P. falciparum growth in vitro reacted with epitopes on the MSP1-83 N-terminal processing fragment [Citation161] included in MSP1FL vaccines [Citation84].
8. Expert opinion
Our main existing deployed tools for malaria control, including drugs and insecticides, lose their activity to increasingly resistant parasites and mosquitoes and raise the challenge of further improving other tools to avoid an increase in morbidity and mortality after decades of successful efforts. The CSP-based pre-erythrocytic RTS,S/AS01 malaria vaccine or its R21 biosimilar has shown that vaccines targeting the major surface protein of P. falciparum sporozoites do significantly reduce clinical malaria especially in children living in sub-Saharan Africa. Building also on evidence obtained from naturally acquired protective humoral immunity, further improvement of the protection level of malaria vaccines might depend on the development of a multistage, multicomponent vaccine targeting both, sporozoites and asexual blood-stage parasites, as these latest stages are the responsible for the clinical manifestations, and tackling the blood stage contributes to the reduction of the reservoir of transmissible sexual stages.
Until a multi-stage vaccine against malaria is generated, the addition of an anti-merozoite blood-stage vaccine based on the recombinantly expressed MSP1FL to vaccination strategies already in place using pre-erythrocytic vaccines is a valuable way to go. MSP1FL delivered with safe adjuvants like GLA-SE has proven to be safe and highly immunogenic in a recently conducted first in human phase 1a clinical trial in naïve volunteers. The capacity of MSP1FL to elicit functional and strain-transcending antibodies is worth exploring in future clinical studies in combination with pre-erythrocytic vaccines such as RTS,S/AS01 or R21.
Antibodies play a central role in protection against malaria infection and clinical malaria. The dissection of antibody Fc-mediated effector functions has shed more light on the range of effector functions mediated by parasite-binding antibodies and their interaction with immune players such as neutrophils, monocytes, NK-cells, and the complement system. These Fc-mediated effector mechanisms are dependent on opsonization in order to trigger inhibition of parasite invasion, antibody-dependent cellular cytotoxicity, respiratory burst of neutrophils, complement fixation, deposition, and membrane attack complex formation, as well as antibody-mediated phagocytosis by neutrophils and monocytes.
Malaria pre-exposure levels impact on the quality, quantity, and duration of malaria vaccine-induced immunity and protection. The availability of clinical research facilities able to perform CHMI studies in malaria pre-exposed populations based on the application of defined dosages of live, non-attenuated purified, cryopreserved P. falciparum sporozoites or iRBC has opened the opportunity to evaluate under highly controlled conditions the protective potential of novel vaccine combinations in the relevant target population. The deployment of system serology studies as immune-monitoring tool in serum samples collected from these clinical trial participants with known protection status against CHMI will help to unravel the functionally most relevant antibody-binding sites on their target antigens and the effector functions mediated. Dissection of vaccine-induced antibody fine specificity and their association to preexisting antibody levels and fine specificity will shed much needed insight on the impact of levels of malaria pre-exposure on vaccine-induced immunity and the resulting immune protection in the different age groups. This knowledge will help to understand how to improve already existing malaria vaccines for different malaria pre-exposed populations.
Article highlights
The critical and life-threatening consequences linked to Plasmodium falciparum malaria are directly attributable to the presence of asexual blood-stage parasites.
Blood-stage malaria vaccines seek to diminish/impede the asexual reproduction of malaria parasites. Various clinical trials centered around P. falciparum blood-stage candidate vaccines have been performed but, regrettably, none has demonstrated robust protective effectiveness.
Ongoing endeavors are concentrated on enhancing liver-stage vaccines which, in the event of not exhibiting sterile immunity, cannot safeguard against the potential advancement of liver-stage merozoites into asexual blood-stage parasites.
The creation of a multi-stage malaria vaccine, combining liver-stage vaccines with an effective blood-stage vaccine, holds the promise of augmenting vaccine-driven protection against clinical disease.
The merozoite surface protein 1 (MSP1) of P. falciparum is a major target of protective immunity and was initially considered an ideal malaria vaccine candidate.
In monkey models, employing the complete MSP1 protein (MSP1FL) for immunization, synergizing conserved domains from both the N- and C-terminal regions of MSP1, resulted in elevated levels of protection against live parasite challenge.
MSP1 human trials have employed subunits or composite formulations comprising diverse smaller domains, but never the MSP1FL, and exhibited poor performance.
An MSP1FL vaccine adjuvanted with GLA-SE has recently been tested for safety and immunogenicity in a first in human phase Ia trial involving malaria-naive volunteers.
Antibodies generated through MSP1FL vaccination demonstrated the ability to stimulate a diverse array of Fc-dependent effector functions in vitro.
The logical progression entails delving into the connection between the spectrum of antibody multi-functionality and their effects on asexual blood-stage parasites in a CHMI model available for use in adult African populations.
Declaration of interests
R Thomson Luque is employee of Sumaya-Biotech GmbH & Co. KG. The authors have no other relevant affiliations or financial involvement with any organization or entity with a financial interest in or financial conflict with the subject matter or materials discussed in the manuscript apart from those disclosed.
Reviewer disclosures
Peer reviewers on this manuscript have no relevant financial or other relationships to disclose.
Author contributions
All authors have contributed to the conception and design of the review article and interpreting the relevant literature and have been involved in writing the review article or revised it for intellectual content.
Dedication
This review is dedicated to Prof. Herman Bujard, an inspiring scientist who, as a force of nature, drew everyone who interacted with him to join his passion to develop an MSP1-based malaria vaccine to protect vulnerable people living in malaria-endemic countries.
Disclaimer
The findings and conclusions in this report are those of the authors and do not necessarily represent the official position of other entities.
Supplemental Material
Download Zip (605.3 KB)Acknowledgments
The authors extend their heartfelt gratitude to the dedicated researchers, institutions, and study volunteers participating in malaria vaccine studies who have relentlessly pursued the formidable objective of jointly creating a malaria vaccine candidate centered around the merozoite surface antigen 1. The collective endeavors of the malaria research community have profoundly driven scientific advancements presented in this manuscript.
Data availability statement
The Plasmodium falciparum whole-genome assemblies used for this work are available from the NCBI (https://www.ncbi.nlm.nih.gov/gene/).
Supplementary Material
Supplemental data for this article can be accessed online at https://doi.org/10.1080/14760584.2023.2295430
Additional information
Funding
References
- World Health Organization. Malaria vaccine: WHO position paper - January 2016. Weekly Epidemiol Rec. 2016;91:33–52.
- Organization, W.H. World malaria report 2021. (2021).
- Hemingway J, Ranson H, Magill A, et al. Averting a malaria disaster: will insecticide resistance derail malaria control? Lancet. 2016;387:1785–1788.
- Organization, W.H. World malaria report 2019. (2019).
- Achan J, Serwanga A, Wanzira H, et al. Current malaria infection, previous malaria exposure, and clinical profiles and outcomes of COVID-19 in a setting of high malaria transmission: an exploratory cohort study in Uganda. Lancet Microbe. 2022;3:e62–e71.
- Cervellati M, Esposito E, Sunde U, et al. Malaria risk and civil violence. Munich, Germany: CESifo; 2017.
- Penny MA, Camponovo F, Chitnis N, et al. Future use-cases of vaccines in malaria control and elimination. Parasite Epidemiol Control. 2020;10:e00145.
- Nussenzweig RS, Vanderberg J, Most H, et al. Protective immunity produced by the injection of x-irradiated sporozoites of plasmodium berghei. Nature. 1967;216:160–162.
- Keating C. The history of the RTS,S/AS01 malaria vaccine trial. Lancet. 2020;395:1336–1337.
- Crompton PD, Moebius J, Portugal S, et al. Malaria immunity in man and mosquito: insights into unsolved mysteries of a deadly infectious disease. Annu Rev Immunol. 2014;32:157–187.
- Beeson JG, Kurtovic L, Valim C, et al. The RTS,S malaria vaccine: current impact and foundation for the future. Sci Transl Med. 2022;14:eabo6646.
- Adepoju P. RTS,S malaria vaccine pilots in three African countries. Lancet. 2019;393:1685.
- World Health Organization. World malaria report 2018. Geneva: World Health Organization; 2018.
- Datoo MS, Natama MH, Somé A, et al. Efficacy of a low-dose candidate malaria vaccine, R21 in adjuvant matrix-M, with seasonal administration to children in Burkina Faso: a randomised controlled trial. Lancet. 2021;397:1809–1818.
- Datoo MS, Natama HM, Somé A, et al. Efficacy and immunogenicity of R21/Matrix-M vaccine against clinical malaria after 2 years’ follow-up in children in Burkina Faso: a phase 1/2b randomised controlled trial. Lancet Infect Dis. 2022;22. doi: 10.1016/S1473-3099(22)00442-X
- Epstein JE, Paolino KM, Richie TL, et al. Protection against Plasmodium falciparum malaria by PfSPZ vaccine. JCI Insight. 2017;2:e89154.
- Richie TL, Church LWP, Murshedkar T, et al. Sporozoite immunization: innovative translational science to support the fight against malaria. Expert Rev Vaccines. 2023;22:964–1007.
- Thera MA, Doumbo OK, Coulibaly D, et al. A field trial to assess a blood-stage malaria vaccine. N Engl J Med. 2011;365:1004–1013.
- Genton B, Reed ZH. Asexual blood-stage malaria vaccine development: facing the challenges. Curr Opin Infect Dis. 2007;20:467–475.
- Duffy PE, Patrick Gorres J. Malaria vaccines since 2000: progress, priorities, products. NPJ Vaccines. 2020;5:48.
- Cai J, Chen S, Zhu F, et al. Whole-killed blood-stage vaccine: is it worthwhile to further develop it to control malaria? Front Microbiol. 2021;12:670775.
- Dunachie SJ, Berthoud T, Keating SM, et al. MIG and the regulatory cytokines IL-10 and TGF-β1 correlate with malaria vaccine immunogenicity and efficacy. PLoS One. 2010;5:e12557.
- Holder AA, Freeman RR. Immunization against blood-stage rodent malaria using purified parasite antigens. Nature. 1981;294:361–364.
- Holder AA, Lockyer MJ, Odink KG, et al. Primary structure of the precursor to the three major surface antigens of Plasmodium falciparum merozoites. Nature. 1985;317:270–273.
- Holder AA. The precursor to major merozoite surface antigens: structure and role in immunity. Prog Allergy. 1988;41:72–97.
- Holder AA, Blackman MJ, Burghaus PA, et al. A malaria merozoite surface protein (MSP1)-structure, processing and function. Mem Inst Oswaldo Cruz. 1992;87 Suppl 3:37–42.
- Koussis K, Withers-Martinez C, Yeoh S, et al. A multifunctional serine protease primes the malaria parasite for red blood cell invasion. EMBO J. 2009;28:725–735.
- Harris PK, Yeoh S, Dluzewski AR, et al. Molecular identification of a malaria merozoite surface sheddase. PLoS Pathog. 2005;1:241–251.
- Blackman MJ, Holder AA. Secondary processing of the Plasmodium falciparum merozoite surface protein-1 (MSP1) by a calcium-dependent membrane-bound serine protease: shedding of MSP133 as a noncovalently associated complex with other fragments of the MSP1. Mol Biochem Parasitol. 1992;50:307–315.
- Child MA, Epp C, Bujard H, et al. Regulated maturation of malaria merozoite surface protein-1 is essential for parasite growth. Mol Microbiol. 2010;78:187–202.
- Dluzewski AR, Ling IT, Hopkins JM, et al. Formation of the food vacuole in Plasmodium falciparum: a potential role for the 19 kDa fragment of merozoite surface protein 1 (MSP1(19)). PLoS One. 2008;3:e3085.
- Trucco C, Fernandez-Reyes D, Howell S, et al. The merozoite surface protein 6 gene codes for a 36 kDa protein associated with the Plasmodium falciparum merozoite surface protein-1 complex. Mol Biochem Parasitol. 2001;112:91–101.
- Stafford WHL, Günder B, Harris A, et al. A 22 kDa protein associated with the Plasmodium falciparum merozoite surface protein-1 complex. Mol Biochem Parasitol. 1996;80:159–169.
- Pachebat JA, Ling IT, Grainger M, et al. The 22 kDa component of the protein complex on the surface of Plasmodium falciparum merozoites is derived from a larger precursor, merozoite surface protein 7. Mol Biochem Parasitol. 2001;117:83–89.
- Chiu CY, Hodder AN, Lin CS, et al. Antibodies to the Plasmodium falciparum proteins MSPDBL1 and MSPDBL2 opsonize merozoites, inhibit parasite growth, and predict protection from clinical malaria. J Infect Dis. 2015;212:406–415.
- Babiker HA, Creasey AM, Fenton B, et al. Genetic diversity of Plasmodium falciparum in a village in eastern Sudan. 1. Diversity of enzymes, 2D-PAGE proteins and antigens. Trans R Soc Trop Med Hyg. 1991;85:572–577.
- Tanabe K, Mackay M, Goman M, et al. Allelic dimorphism in a surface antigen gene of the malaria parasite Plasmodium falciparum. J Mol Biol. 1987;195:273–287.
- Takala S, Branch O, Escalante AA, et al. Evidence for intragenic recombination in Plasmodium falciparum: identification of a novel allele family in block 2 of merozoite surface protein-1: Asembo Bay Area Cohort Project XIV. Mol Biochem Parasitol. 2002;125:163–171.
- Tanabe K, Sakihama N, Walliker D, et al. Allelic dimorphism-associated restriction of recombination in Plasmodium falciparum msp1. Gene. 2007;397:153–160.
- Kiwanuka GN. Genetic diversity in Plasmodium falciparum merozoite surface protein 1 and 2 coding genes and its implications in malaria epidemiology: a review of published studies from 1997-2007. J Vector Borne Dis. 2009;46:1–12.
- Levinson G, Gutman GA. Slipped-strand mispairing: a major mechanism for DNA sequence evolution. Mol Biol Evol. 1987;4:203–221.
- Ferreira MU, Ribeiro WL, Tonon AP, et al. Sequence diversity and evolution of the malaria vaccine candidate merozoite surface protein-1 (MSP-1) of Plasmodium falciparum. Gene. 2003;304:65–75.
- Gentz R, Certa U, Takacs B, et al. Major surface antigen p190 of Plasmodium falciparum: detection of common epitopes present in a variety of plasmodia isolates. Embo J. 1988;7:225–230.
- Jongwutiwes S, Tanabe K, Nakazawa S, et al. Sequence variation in the tripeptide repeats and T cell epitopes in P190 (MSA-1) of Plasmodium falciparum from field isolates. Mol Biochem Parasitol. 1992;51:81–89.
- Agonhossou R, Akoton R, Lagnika H, et al. P. falciparum msp1 and msp2 genetic diversity in P. falciparum single and mixed infection with P. malariae among the asymptomatic population in Southern Benin. Parasitol Int. 2022;89:102590.
- Mwingira F, Nkwengulila G, Schoepflin S, et al. Plasmodium falciparum msp1, msp2 and glurp allele frequency and diversity in sub-Saharan Africa. Malar J. 2011;10:79.
- Chen J-T, Li J, Zha G-C, et al. Genetic diversity and allele frequencies of Plasmodium falciparum msp1 and msp2 in parasite isolates from Bioko Island, Equatorial Guinea. Malar J. 2018;17:458.
- Apinjoh TO, Tata RB, Anchang-Kimbi JK, et al. Plasmodium falciparum merozoite surface protein 1 block 2 gene polymorphism in field isolates along the slope of mount Cameroon: a cross – sectional study. BMC Infect Dis. 2015;15:309.
- Somé AF, Bazié T, Zongo I, et al. Plasmodium falciparum msp1 and msp2 genetic diversity and allele frequencies in parasites isolated from symptomatic malaria patients in Bobo-Dioulasso, Burkina Faso. Parasit Vectors. 2018;11:323.
- Miller LH, Roberts T, Shahabuddin M, et al. Analysis of sequence diversity in the Plasmodium falciparum merozoite surface protein-1 (MSP-1). Mol Biochem Parasitol. 1993;59:1–14.
- Snounou G, Beck HP. The use of PCR genotyping in the assessment of recrudescence or reinfection after antimalarial drug treatment. Parasitol Today. 1998;14:462–467.
- Cattamanchi A, Kyabayinze D, Hubbard A, et al. Distinguishing recrudescence from reinfection in a longitudinal antimalarial drug efficacy study: comparison of results based on genotyping of msp-1, msp-2, and glurp. Am J Trop Med Hyg. 2003;68:133–139.
- Mohammed H, Mindaye T, Belayneh M, et al. Genetic diversity of Plasmodium falciparum isolates based on MSP-1 and MSP-2 genes from Kolla-Shele area, Arbaminch Zuria District, southwest Ethiopia. Malar J. 2015;14:73.
- Otto TD, Böhme U, Sanders MJ, et al. Long read assemblies of geographically dispersed Plasmodium falciparum isolates reveal highly structured subtelomeres. Wellcome Open Res. 2018;3(52). doi: 10.12688/wellcomeopenres.14571.1
- Moser KA, Dwivedi A, Stucke EM, et al. New Plasmodium falciparum genome assemblies from diverse endemic regions enables the comprehensive genomic and genetic characterization of clinical isolates. Am J Trop Med Hyg. 2017;97:511–511. AMER SOC TROP MED & HYGIENE 8000 WESTPARK DR, STE 130, MCLEAN, VA 22101 USA
- Tanabe K, Zollner G, Vaughan JA, et al. Plasmodium falciparum: genetic diversity and complexity of infections in an isolated village in western thailand. Parasitol Int. 2015;64:260–266.
- Tanabe K, Mita T, Palacpac NMQ, et al. Within-population genetic diversity of Plasmodium falciparum vaccine candidate antigens reveals geographic distance from a Central sub-Saharan African origin. Vaccine. 2013;31:1334–1339.
- White WL. Erratum to: why I hate the index finger. Hand (N Y). 2011;6:233.
- Tanabe K, Sakihama N, Kaneko A. Stable SNPs in malaria antigen genes in isolated populations. Science. 2004;303:493.
- Takala SL, Escalante AA, Branch OH, et al. Genetic diversity in the Block 2 region of the merozoite surface protein 1 (MSP-1) of Plasmodium falciparum: additional complexity and selection and convergence in fragment size polymorphism. Infect Genet Evol. 2006;6:417–424.
- Rich SM, Ayala FJ. Population structure and recent evolution of Plasmodium falciparum. Proc Natl Acad Sci U S A. 2000;97:6994–7001.
- Aponte JJ, Menendez C, Schellenberg D, et al. Age interactions in the development of naturally acquired immunity to Plasmodium falciparum and its clinical presentation. PLoS Med. 2007;4:e242.
- Ellis RD, Wu Y, Martin LB, et al. Phase 1 study in malaria naïve adults of BSAM2/Alhydrogel®+CPG 7909, a blood stage vaccine against P. falciparum malaria. PLoS One. 2012;7:e46094.
- Cohen S, Mc GI, Carrington S. Gamma-globulin and acquired immunity to human malaria. Nature. 1961;192:733–737.
- Hviid L, Lopez-Perez M, Larsen MD, et al. No sweet deal: the antibody-mediated immune response to malaria. Trends Parasitol. 2022;38:428–434.
- al-Yaman F, Genton B, Kramer KJ, et al. Assessment of the role of naturally acquired antibody levels to Plasmodium falciparum merozoite surface protein-1 in protecting Papua New Guinean children from malaria morbidity. Am J Trop Med Hyg. 1996;54:443–448.
- Egan AF, Morris J, Barnish G, et al. Clinical immunity to Plasmodium falciparum malaria is associated with serum antibodies to the 19-kDa C-terminal fragment of the merozoite surface antigen, PfMSP-1. J Infect Dis. 1996;173:765–769.
- Dodoo D, Theander TG, Kurtzhals JAL, et al. Levels of antibody to conserved parts of Plasmodium falciparum merozoite surface protein 1 in Ghanaian children are not associated with protection from clinical malaria. Infect Immun. 1999;67:2131–2137.
- Nebie I, Diarra A, Ouedraogo A, et al. Humoral responses to Plasmodium falciparum blood-stage antigens and association with incidence of clinical malaria in children living in an area of seasonal malaria transmission in Burkina Faso, West Africa. Infect Immun. 2008;76:759–766.
- Osier FH, Fegan G, Polley SD, et al. Breadth and magnitude of antibody responses to multiple Plasmodium falciparum merozoite antigens are associated with protection from clinical malaria. Infect Immun. 2008;76:2240–2248.
- Bowman NM, Moormann AM, Bowman NM, et al. Longevity of genotype-specific immune responses to Plasmodium falciparum merozoite surface protein 1 in Kenyan children from regions of different malaria transmission intensity. Am J Trop Med Hyg. 2016;95:580–587.
- Früh K, Doumbo O, Müller HM, et al. Human antibody response to the major merozoite surface antigen of Plasmodium falciparum is strain specific and short-lived. Infect Immun. 1991;59:1319–1324.
- Muller HM, Früh K, von Brunn A, et al. Development of the human immune response against the major surface protein (gp190) of Plasmodium falciparum. Infect Immun. 1989;57:3765–3769.
- Tolle R, Früh K, Doumbo O, et al. A prospective study of the association between the human humoral immune response to Plasmodium falciparum blood stage antigen gp190 and control of malarial infections. Infect Immun. 1993;61:40–47.
- Fowkes FJ, Richards JS, Simpson JA, et al. The relationship between anti-merozoite antibodies and incidence of Plasmodium falciparum malaria: a systematic review and meta-analysis. PLoS Med. 2010;7:e1000218.
- Conway DJ, Cavanagh DR, Tanabe K, et al. A principal target of human immunity to malaria identified by molecular population genetic and immunological analyses. Nat Med. 2000;6:689–692.
- Noranate N, Prugnolle F, Jouin H, et al. Population diversity and antibody selective pressure to Plasmodium falciparum MSP1 block2 locus in an African malaria-endemic setting. BMC Microbiol. 2009;9:219.
- Cavanagh DR, Dodoo D, Hviid L, et al. Antibodies to the N-terminal block 2 of Plasmodium falciparum merozoite surface protein 1 are associated with protection against clinical malaria. Infect Immun. 2004;72:6492–6502.
- Jaschke A, Coulibaly B, Remarque EJ, et al. Merozoite surface protein 1 from Plasmodium falciparum is a major target of opsonizing antibodies in individuals with acquired immunity against malaria. Clin Vaccine Immunol. 2017;24. DOI:10.1128/CVI.00155-17
- Saul A, Lawrence G, Smillie A, et al. Human phase I vaccine trials of 3 recombinant asexual stage malaria antigens with Montanide ISA720 adjuvant. Vaccine. 1999;17:3145–3159.
- Genton B, Betuela I, Felger I, et al. A recombinant blood-stage malaria vaccine reduces Plasmodium falciparum density and exerts selective pressure on parasite populations in a phase 1–2b trial in Papua New Guinea. J Infect Dis. 2002;185:820–827.
- Lawrence G, Cheng Q, Reed C, et al. Effect of vaccination with 3 recombinant asexual-stage malaria antigens on initial growth rates of Plasmodium falciparum in non-immune volunteers. Vaccine. 2000;18:1925–1931.
- Genton B, Al-Yaman F, Anders R, et al. Safety and immunogenicity of a three-component blood-stage malaria vaccine in adults living in an endemic area of Papua New Guinea. Vaccine. 2000;18:2504–2511.
- Blank A, Fürle K, Jäschke A, et al. Immunization with full-length Plasmodium falciparum merozoite surface protein 1 is safe and elicits functional cytophilic antibodies in a randomized first-in-human trial. NPJ Vaccines. 2020;5:10.
- Nziza N, Tran TM, DeRiso EA, et al. Accumulation of neutrophil phagocytic antibody features tracks with naturally acquired immunity against malaria in children. J Infect Dis. 2023;228:759–768.
- Perrin LH, Merkli B, Loche M, et al. Antimalarial immunity in Saimiri monkeys. Immunization with surface components of asexual blood stages. J Exp Med. 1984;160:441–451.
- Siddiqui WA, Tam LQ, Kramer KJ, et al. Merozoite surface coat precursor protein completely protects Aotus monkeys against Plasmodium falciparum malaria. Proc Natl Acad Sci U S A. 1987;84:3014–3018.
- Herrera S, Herrera MA, Perlaza BL, et al. Immunization of Aotus monkeys with Plasmodium falciparum blood-stage recombinant proteins. Proc Natl Acad Sci U S A. 1990;87:4017–4021.
- Etlinger HM, Caspers P, Matile H, et al. Ability of recombinant or native proteins to protect monkeys against heterologous challenge with Plasmodium falciparum. Infect Immun. 1991;59:3498–3503.
- Patarroyo ME, Romero P, Torres ML, et al. Induction of protective immunity against experimental infection with malaria using synthetic peptides. Nature. 1987;328:629–632.
- Rodriguez R, Moreno A, Guzman F, et al. Studies in owl monkeys leading to the development of a synthetic vaccine against the asexual blood stages of Plasmodium falciparum. Am J Trop Med Hyg. 1990;43:339–354.
- Moreno A, Patarroyo ME. Development of an asexual blood stage malaria vaccine. Blood. 1989;74:537–546.
- Ruebush TKN, Campbell GH, Moreno A, et al. Immunization of owl monkeys with a combination of Plasmodium falciparum asexual blood-stage synthetic peptide antigens. Am J Trop Med Hyg. 1990;43:355–366.
- Herrera S, Guerrero R, Clavijo C, et al. Failure of a synthetic vaccine to protect Aotus lemurinus against asexual blood stages of Plasmodium falciparum. Am J Trop Med Hyg. 1992;47:682–690.
- Amador R, Moreno A, Murillo LA, et al. Safety and immunogenicity of the synthetic malaria vaccine SPf66 in a large field trial. J Infect Dis. 1992;166:139–144.
- Amador R, Moreno A, Valero V, et al. The first field trials of the chemically synthesized malaria vaccine SPf66: safety, immunogenicity and protectivity. Vaccine. 1992;10:179–184.
- Valero MV, Amador LR, Galindo C, et al. Vaccination with SPf66, a chemically synthesised vaccine, against Plasmodium falciparum malaria in Colombia. Lancet. 1993;341:705–710.
- Sempértegui F, Estrella B, Moscoso J, et al. Safety, immunogenicity and protective effect of the SPf66 malaria synthetic vaccine against Plasmodium falciparum infection in a randomized double-blind placebo-controlled field trial in an endemic area of Ecuador. Vaccine. 1994;12:337–342.
- Noya O, Berti YG, Noya BAD, et al. A population-based clinical trial with the SPf66 synthetic Plasmodium falciparum malaria vaccine in Venezuela. J Infect Dis. 1994;170:396–402.
- Teuscher T, Armstrong Schellenberg JRM, Bastos de Azevedo I, et al. SPf66, a chemically synthesized subunit malaria vaccine, is safe and immunogenic in Tanzanians exposed to intense malaria transmission. Vaccine. 1994;12:328–336.
- Alonso P, Smith T, Armstrong Schellenberg JRM, et al. Randomised trial of efficacy of SPf66 vaccine against Plasmodium falciparum malaria in children in southern Tanzania. Lancet. 1994;344:1175–1181.
- Acosta C, Galindo CM, Schellenberg D, et al. Evaluation of the SPf66 vaccine for malaria control when delivered through the EPI scheme in Tanzania. Trop Med Int Health. 1999;4:368–376.
- D’alessandro U, Leach A, Olaleye BO, et al. Efficacy trial of malaria vaccine SPf66 in Gambian infants. Lancet. 1995;346:462–467.
- Nosten F, Luxemburger C, Kyle DE, et al. Randomised double-blind placebo-controlled trial of SPf66 malaria vaccine in children in northwestern Thailand. Lancet. 1996;348:701–707.
- Urdaneta M, Struchiner CJ, Boulos M, et al. Evaluation of SPf66 malaria vaccine efficacy in Brazil. The American Journal of Tropical Medicine and Hygiene. 1998;58:378–385.
- Kumar S, Yadava A, Keister DB, et al. Immunogenicity and in vivo efficacy of recombinant Plasmodium falciparum merozoite surface protein-1 in Aotus monkeys. Mol Med. 1995;1:325–332.
- Darko CA, Angov E, Collins WE, et al. The clinical-grade 42-kilodalton fragment of merozoite surface protein 1 of Plasmodium falciparum strain FVO expressed in Escherichia coli protects Aotus nancymai against challenge with homologous erythrocytic-stage parasites. Infect Immun. 2005;73:287–297.
- Singh S, Miura K, Zhou H, et al. Immunity to recombinant Plasmodium falciparum merozoite surface protein 1 (MSP1): protection in Aotus nancymai monkeys strongly correlates with anti-MSP1 antibody titer and in vitro parasite-inhibitory activity. Infect Immun. 2006;74:4573–4580.
- Alaro JR, Partridge A, Miura K, et al. A chimeric Plasmodium falciparum merozoite surface protein vaccine induces high titers of parasite growth inhibitory antibodies. Infect Immun. 2013;81:3843–3854.
- Burns JM Jr, Miura K, Sullivan J, et al. Immunogenicity of a chimeric Plasmodium falciparum merozoite surface protein vaccine in Aotus monkeys. Malar J. 2016;15:159.
- Shi Q, Lynch MM, Romero M, et al. Enhanced protection against malaria by a chimeric merozoite surface protein vaccine. Infect Immun. 2007;75:1349–1358.
- Alaro JR, Lynch MM, Burns JM Jr. Protective immune responses elicited by immunization with a chimeric blood-stage malaria vaccine persist but are not boosted by Plasmodium yoelii challenge infection. Vaccine. 2010;28:6876–6884.
- Cowan GJ, Creasey AM, Dhansarnsombut K, et al. A malaria vaccine based on the polymorphic block 2 region of MSP-1 that elicits a broad serotype-spanning immune response. PLoS One. 2011;6:e26616.
- Cavanagh DR, Kocken CHM, White JH, et al. Antibody responses to a novel Plasmodium falciparum merozoite surface protein vaccine correlate with protection against experimental malaria infection in Aotus monkeys. PLoS One. 2014;9:e83704.
- Graves P, Gelband H. Vaccines for preventing malaria (SPf66). Cochrane Database Syst Rev. 2006;2006:Cd005966.
- Daubenberger CA, Nickel B, Ciatto C, et al. Amino acid dimorphism and parasite immune evasion: cellular immune responses to a promiscuous epitope of Plasmodium falciparum merozoite surface protein 1 displaying dimorphic amino acid polymorphism are highly constrained. Eur J Immunol. 2002;32:3667–3677.
- Pöltl-Frank F, Zurbriggen R, Helg A, et al. Use of reconstituted influenza virus virosomes as an immunopotentiating delivery system for a peptide-based vaccine. Clin Exp Immunol. 1999;117:496–503.
- Keitel WA, Kester KE, Atmar RL, et al. Phase I trial of two recombinant vaccines containing the 19kd carboxy terminal fragment of Plasmodium falciparum merozoite surface protein 1 (msp-1(19)) and T helper epitopes of tetanus toxoid. Vaccine. 1999;18:531–539.
- Stoute JA, Gombe J, Withers MR, et al. Phase 1 randomized double-blind safety and immunogenicity trial of Plasmodium falciparum malaria merozoite surface protein FMP1 vaccine, adjuvanted with AS02A, in adults in western Kenya. Vaccine. 2007;25:176–184.
- Ockenhouse CF, Angov E, Kester KE, et al. Phase I safety and immunogenicity trial of FMP1/AS02A, a Plasmodium falciparum MSP-1 asexual blood stage vaccine. Vaccine. 2006;24:3009–3017.
- Withers MR, McKinney D, Ogutu BR, et al. Safety and reactogenicity of an MSP-1 malaria vaccine candidate: a randomized phase Ib dose-escalation trial in Kenyan children. PLoS Clin Trials. 2006;1:e32.
- Thera MA, Doumbo OK, Coulibaly D, et al. Safety and allele-specific immunogenicity of a malaria vaccine in Malian adults: results of a phase I randomized trial. PLoS Clin Trials. 2006;1:e34.
- Ogutu BR, Apollo OJ, McKinney D, et al. Blood stage malaria vaccine eliciting high antigen-specific antibody concentrations confers no protection to young children in Western Kenya. PLoS One. 2009;4:e4708.
- Malkin E, Long CA, Stowers AW, et al. Phase 1 study of two merozoite surface protein 1 (MSP1(42)) vaccines for Plasmodium falciparum malaria. PLoS Clin Trials. 2007;2:e12.
- Ellis RD, Martin LB, Shaffer D, et al. Phase 1 trial of the Plasmodium falciparum blood stage vaccine MSP1(42)-C1/Alhydrogel with and without CPG 7909 in malaria naive adults. PLoS One. 2010;5:e8787.
- Sheehy SH, Duncan CJ, Elias SC, et al. Phase Ia clinical evaluation of the Plasmodium falciparum blood-stage antigen MSP1 in ChAd63 and MVA vaccine vectors. Mol Ther. 2011;19:2269–2276.
- Malkin E, Hu J, Li Z, et al. A phase 1 trial of PfCP2.9: an AMA1/MSP1 chimeric recombinant protein vaccine for Plasmodium falciparum malaria. Vaccine. 2008;26:6864–6873.
- Sheehy SH, Duncan CJ, Elias SC, et al. ChAd63-MVA-vectored blood-stage malaria vaccines targeting MSP1 and AMA1: assessment of efficacy against mosquito bite challenge in humans. Mol Ther. 2012;20:2355–2368.
- Biswas S, Choudhary P, Elias SC, et al. Assessment of humoral immune responses to blood-stage malaria antigens following ChAd63-MVA immunization, controlled human malaria infection and natural exposure. PLoS One. 2014;9:e107903.
- Chitnis CE, Mukherjee P, Mehta S, et al. Phase I clinical trial of a recombinant blood stage vaccine candidate for Plasmodium falciparum malaria based on MSP1 and EBA175. PLoS One. 2015;10:e0117820.
- Hall R, Hyde JE, Goman M, et al. Major surface antigen gene of a human malaria parasite cloned and expressed in bacteria. Nature. 1984;311:379–382.
- Epp C, Kauth CW, Bujard H, et al. Expression and purification of Plasmodium falciparum MSP-1(42): a malaria vaccine candidate. J Chromatogr B Analyt Technol Biomed Life Sci. 2003;786:61–72.
- Dijkman PM, Marzluf T, Zhang Y, et al. Structure of the merozoite surface protein 1 from Plasmodium falciparum. Sci Adv. 2021;7. doi: 10.1126/sciadv.abg0465
- Kauth CW, Epp C, Bujard H, et al. The merozoite surface protein 1 complex of human malaria parasite Plasmodium falciparum: interactions and arrangements of subunits. J Biol Chem. 2003;278:22257–22264.
- Joos C, Marrama L, Polson HEJ, et al. Clinical protection from falciparum malaria correlates with neutrophil respiratory bursts induced by merozoites opsonized with human serum antibodies. PLoS One. 2010;5:e9871.
- Boyle MJ, Reiling L, Feng G, et al. Human antibodies fix complement to inhibit Plasmodium falciparum invasion of erythrocytes and are associated with protection against malaria. Immunity. 2015;42:580–590.
- Reiling L, Boyle MJ, White MT, et al. Targets of complement-fixing antibodies in protective immunity against malaria in children. Nat Commun. 2019;10:610.
- Hill DL, Eriksson EM, Li Wai Suen CSN, et al. Opsonising antibodies to P. falciparum merozoites associated with immunity to clinical malaria. PLoS One. 2013;8:e74627.
- Osier FH, Feng G, Boyle MJ, et al. Opsonic phagocytosis of Plasmodium falciparum merozoites: mechanism in human immunity and a correlate of protection against malaria. BMC Med. 2014;12:108.
- Kana IH, Garcia-Senosiain A, Singh SK, et al. Cytophilic antibodies against key Plasmodium falciparum blood stage antigens contribute to protection against clinical malaria in a high transmission region of Eastern India. J Infect Dis. 2018;218:956–965.
- Odera DO, Tuju J, Mwai K, et al. Antibodies targeting merozoites induce natural killer cell degranulation and interferon gamma secretion and are associated with immunity against malaria. Sci Transl Med. 2023;151:eabn5993. doi: 10.1126/scitranslmed.abn5993.
- Feng G, Boyle MJ, Cross N, et al. Human immunization with a polymorphic malaria vaccine candidate induced antibodies to conserved epitopes that promote functional antibodies to multiple parasite strains. J Infect Dis. 2018;218:35–43.
- Garcia-Senosiain A, Kana IH, Singh S, et al. Neutrophils dominate in opsonic phagocytosis of P. falciparum blood-stage merozoites and protect against febrile malaria. Commun Biol. 2021;4:984.
- Nkumama IN, Odera D, Musasia F, et al. Breadth of Fc-mediated effector function delineates grades of clinical immunity following human malaria challenge. bioRxiv. 2022;2022:2010.2011.511755.
- Rosenkranz M, Nkumama IN, Kraker S, et al. Full-length merozoite surface protein 1 of Plasmodium falciparum is a major target of protective immunity following controlled human malaria infections. medRxiv. 2022;2022.2010.2012.22280947.
- Rosenkranz M, Fürle K, Hibbert J, et al. Multifunctional IgG/IgM antibodies and cellular cytotoxicity are elicited by the full-length MSP1 SumayaVac-1 malaria vaccine. NPJ Vaccines. 2023;8:112.
- Marsh K, Kinyanjui S. Immune effector mechanisms in malaria. Parasite Immunol. 2006;28:51–60.
- Conway DJ, Greenwood BM, McBride JS. Longitudinal study of Plasmodium falciparum polymorphic antigens in a malaria-endemic population. Infect Immun. 1992;60:1122–1127.
- Riley EM, ALLEN SJ, Wheeler JG, et al. Naturally acquired cellular and humoral immune responses to the major merozoite surface antigen (Pf MSP1) of Plasmodium falciparum are associated with reduced malaria morbidity. Parasite Immunol. 1992;14:321–337.
- Volz JC, Yap A, Sisquella X, et al. Essential role of the PfRh5/PfRipr/CyRPA complex during Plasmodium falciparum invasion of erythrocytes. Cell Host Microbe. 2016;20:60–71.
- Douglas AD, Baldeviano G, Lucas C, et al. A PfRH5-based vaccine is efficacious against heterologous strain blood-stage Plasmodium falciparum infection in Aotus monkeys. Cell Host Microbe. 2015;17:130–139.
- Payne RO, Silk SE, Elias SC, et al. Human vaccination against RH5 induces neutralizing antimalarial antibodies that inhibit RH5 invasion complex interactions. JCI Insight. 2017;2. doi: 10.1172/jci.insight.96381
- Douglas AD, Baldeviano GC, Jin J, et al. A defined mechanistic correlate of protection against Plasmodium falciparum malaria in non-human primates. Nat Commun. 2019;10:1953.
- Minassian AM, Silk SE, Barrett JR, et al. Reduced blood-stage malaria growth and immune correlates in humans following RH5 vaccination. Med (N Y). 2021;2:701–719.e719.
- Tan J, Sack BK, Oyen D, et al. A public antibody lineage that potently inhibits malaria infection through dual binding to the circumsporozoite protein. Nat Med. 2018;24:401–407.
- Kayentao K, Ongoiba A, Preston AC, et al. Safety and efficacy of a monoclonal antibody against malaria in Mali. N Engl J Med. 2022;387:1833–1842.
- Chappel JA, Holder AA. Monoclonal antibodies that inhibit Plasmodium falciparum invasion in vitro recognise the first growth factor-like domain of merozoite surface protein-1. Mol Biochem Parasitol. 1993;60:303–311.
- Uthaipibull C, Aufiero B, Syed SEH, et al. Inhibitory and blocking monoclonal antibody epitopes on merozoite surface protein 1 of the malaria parasite Plasmodium falciparum11Edited by J. A. Wells. J Mol Biol. 2001;307:1381–1394.
- Locher CP, Tam LQ, Chang SP, et al. Plasmodium falciparum: gp195 tripeptide repeat-specific monoclonal antibody inhibits parasite growth in vitro. Exp Parasitol. 1996;84:74–83.
- Patel PN, Dickey TH, Hopp CS, et al. Neutralizing and interfering human antibodies define the structural and mechanistic basis for antigenic diversion. Nat Commun. 2022;13:5888.
- Lin CS, Uboldi AD, Epp C, et al. Multiple Plasmodium falciparum merozoite surface protein 1 complexes mediate merozoite binding to human erythrocytes. J Biol Chem. 2016;291:7703–7715.