ABSTRACT
Introduction
Current vaccines vary widely in both their efficacy against infection and disease, and the durability of the efficacy. Some vaccines provide practically lifelong protection with a single dose, while others provide only limited protection following annual boosters. What variables make vaccine-induced immune responses last? Can breakthroughs in these factors and technologies help us produce vaccines with better protection and fewer doses? The durability of vaccine-induced protection is now a hot area in vaccinology research, especially after COVID-19 vaccines lost their luster. It has fueled discussion on the eventual utility of existing vaccines to society and bolstered the anti-vaxxer camp. To sustain public trust in vaccines, lasting vaccines must be developed.
Areas covered
This review summarizes licensed vaccines’ protection. It analyses immunological principles and vaccine and vaccinee parameters that determine longevity of antibodies. The review concludes with challenges and the way forward to improve vaccine durability.
Expert opinion
Despite enormous advances, we still lack essential markers and reliable correlates of lasting protection. Most research has focused on humoral immune responses, but we must also focus on innate, mucosal, and cellular responses – their assessment, correlates, determinants, and novel adjuvants. Suitable vaccine designs and platforms for durable immunity must be found.
1. Introduction
‘Waning of immunity’ became a buzzword not only in academia and industry but also among the lay public when it became clear that the protection conferred by COVID-19 vaccines is not as durable as offered by other vaccines like measles. The excitement over the speedy development and rapid deployment of COVID-19 vaccines gradually waned off, and soon, a debate surrounding the durability of immune protection ensued. Thus, the durability of vaccine-induced protection is a hot area of research today. What factors determine the durability of vaccine-induced immune protection? Does the nature of the pathogen (fast mutating virus vs. ‘stable’ virus), the biology of the vaccine (live attenuated vs. inactivated viral vaccine, the addition of an adjuvant and the type), or the vaccination schedule (dose, number of doses and interval between doses) have any bearing on the long term persistence of vaccine-derived immunity? It is also known that natural infections caused by most human coronaviruses, including Severe Acute Respiratory Syndrome Coronavirus-2 (SARS-CoV2) and influenza, fail to provide long-lasting protection, unlike other respiratory pathogens like measles, rubella, varicella, and mumps [Citation1]. The current generation of vaccines against all these pathogens also follows a similar pattern.
This review provides an overview of the durability of protection offered by the currently used licensed vaccines. It summarizes the current knowledge, recent advances, knowledge gaps, challenges, and bottlenecks regarding the long-term protection induced by vaccines and how to overcome these.
2. Overview of the durability of protection conferred by the key licensed vaccines
To perform an analysis of the durability of immune protection accorded by the currently licensed vaccines, we have broadly classified them under three heads ():
Vaccines that provide long duration of protection (>20 years).
Vaccines that provide moderate duration of protection (5-20 years).
Vaccines that provide short duration of protection (<5 years).
Table 1. A summary of currently licensed vaccines grouped based on their duration of protection, along with other vital parameters.
2.1. Vaccines that provide a long duration of protection (>20 years)
2.1.1. Measles vaccines
A single dose of vaccine offers lifelong protection to nearly all vaccinees. The primary immune response is boosted with exposure to wild viruses or after re-vaccination [Citation2]. Once seroconverted, protective anti-measles antibodies ≥ 120 mIU/mL are detected for a very long period, probably for life, without any significant waning in the antibody titers. A modeling study estimated the half-life of anti-measles antibodies to be more than 3000 years [Citation3].
2.1.5. Rubella vaccine
Rubella vaccine also induces a robust immune response that is presumed to be lifelong [Citation4]. Rubella virus-specific humoral immune responses wane with time after vaccination, but they do so moderately [Citation5]. Though no absolute immune correlate of protection has been established, a cutoff level of anti-rubella IgG antibodies of ≥ 10 IU/mL is generally protective against rubella disease [Citation4]. One longitudinal study estimated that rubella-specific immunity was maintained, with an estimated half-life of 114 years and no significant rate of titer decrease [Citation3].
2.1.3. Yellow fever vaccine
The live-attenuated yellow fever vaccine has excellent long-term effectiveness. The titer of neutralizing antibodies is the correlate of protection. Plaque reduction neutralization test (PRNT50) assays with a titer of 1 in 10 are used in most clinical trials to correlate protection.
According to Poland et al. [Citation6], 80.6% of World War II soldiers had neutralizing antibodies that lasted longer than thirty years. Neutralizing antibodies have also shown long-term persistence in several additional studies conducted in both endemic and non-endemic countries [Citation7–10]. Since there are no documented cases of Yellow Fever infection in individuals with documented seroconversion, it has been argued that the hypothesis that the vaccine provides lifelong protection is correct [Citation11].
2.1.4. Hepatitis-B vaccine
The Hepatitis B vaccine is a T-cell-dependent subunit vaccine, and hepatitis B infection has a long incubation period, allowing enough time for an anamnestic response to occur if there is contact with the virus. Hence, once seroconversion occurs, the vaccine can provide long-term protection even if antibody titers fall below the protective level of 10mIU/mL.
2.1.5. Hepatitis-A inactivated vaccine
Many modeling and anti-HAV kinetic studies have confirmed that a high level of seroprotection can be achieved for at least 30 years by a single or two-dose vaccine schedule [Citation12,Citation13]. Furthermore, protection against the clinical disease may persist even without detectable antibodies in the presence of memory B and T cells [Citation14].
2. Vaccines that provide moderate duration of protection (5–20 years)
2.2.1. Oral polio vaccine (OPV)
After seroconversion, OPV offers almost lifelong protection against paralytic poliomyelitis, even if the antibody titers fall below detectable levels later in life. However, seroconversion and efficacy vary between different geographical settings: higher in temperate climates in industrialized countries than in developing countries with tropical conditions. According to an Italian study, immune protection against paralytic disease persisted up to 18 years after administration of the last OPV dose, with protective antibodies against poliovirus type 1 and 2 detected in more than 95% of the subjects even after 30 years of the last OPV dose [Citation15].
2.2.2. Inactivated poliovirus vaccine (IPV)
Studies from industrialized countries have indicated that IPV-induced antibodies protect from paralytic polio for many decades, probably for life [Citation16]. According to a retrospective cohort study from Italy, neutralizing antibodies against all three polio types persisted in more than 90% of study participants 18 years after the last vaccine dose [Citation17]. In this study, the IPV induced higher titers of neutralizing antibodies than OPV.
2.2.3. Hepatitis A live attenuated vaccine
Like inactivated vaccines, live attenuated hepatitis-A vaccines also offer a long duration of protection. After a single dose of H2 strain-based live attenuated vaccine in an endemic region, at least 15–17 years of protection has been demonstrated along with the persistence of immune memory [Citation18–20].
2.2.4. Human papilloma virus (HPV) vaccines
These vaccines are unique in that they offer long-lasting protection against HPV-related illnesses even with a single dose, and the protection they offer is virtually sterilizing, meaning that they prevent infection rather than just illness [Citation21]. This is despite them being non-live attenuated vaccines. Bivalent, quadrivalent, and nonavalent vaccines have shown the most extended durations of preventative effect: 11 years in the Costa Rica trial [Citation22], 14 years in the FUTURE II trial [Citation23], and eight years in the long-term follow-up (LTFU) extension study [Citation24] and Scandinavian Study [Citation25]. An Australian real-world effectiveness study [Citation26] has the longest observation time of 12 years for vaccine efficacy (for the quadrivalent vaccine).
2.2.5. Bacille Calmette-Guérin (BCG) vaccine
Even while the BCG vaccine’s efficacy varies greatly depending on the clinical entity and demography, it offers quite a long period of protection – even up to 50 years [Citation27–29]. A thorough analysis found that in certain groups, protection following a baby BCG vaccine could continue up to 15 years; nevertheless, the protective efficacy does eventually wane [Citation30].
2.2.6. Diphtheria
Protective levels of diphtheria antibodies (0.1IU/mL) persist for decades after vaccination, although they wane in a section of vaccinees after ten years [Citation31]. The half-life of diphtheria-specific antibodies is estimated to be around 19 years [Citation32]. Considering this and the fact that diphtheria infection has a short incubation period, and the natural boosting is also limited with high vaccination coverage, its booster is recommended every ten years, especially in industrialized countries, to maintain protective levels. However, recent studies have suggested that the protective titers persist much longer in the majority, and less frequent boosters should be sufficient [Citation33].
2.2.7. Tetanus
The duration of protection accorded by the tetanus vaccine is also similar to the diphtheria antigen. The protective level of 0.1 IU/ml of anti-tetanus antibodies persists for 3–5 years after the primary schedule of 3 doses. Then, it may persist lifelong after boosters in the second and fifth years of life and another during the adolescent age in some people [Citation33]. The half-life of tetanus-specific antibodies is estimated to be around 11 years [Citation31]. However, in most persons, the level approaches minimal protective levels ten years after the last dose [Citation34]. Thus, its booster is recommended every ten years to maintain protective levels. Recent studies have indicated that less frequent boosting might be enough [Citation35].
2.2.8. Varicella zoster vaccine
Many studies from the U.S.A. and Japan demonstrated the long-term persistence of anti-varicella antibodies after a single dose of varicella vaccine [Citation36]. However, there are concerns that these studies were conducted when the virus had a substantial circulation in the community, and there may be some waning now due to the absence of widespread transmission. Later studies have demonstrated a waning in immunity after a single dose of the OKA-strain varicella vaccine [Citation37]. Though anti-varicella antibodies are estimated to have a half-life of 50 years, there are frequent fluctuations in the titers.
2.2.9. Mumps vaccines
Although wild mumps virus infection provides lifelong immunity, recurrences of mumps disease are reported [Citation38]. There are at least 13 different strains of Mumps virus employed in live attenuated vaccines [Citation39]. They differ in efficacy to a certain extent, but overall, they protect 80% of the recipients.
Some studies have reported 60–90% effectiveness after a single dose [Citation39]. According to a Cochrane review of 64 MMR vaccine studies, one dose’s effectiveness was 69%-81% [Citation40].
Another study finds that mumps-specific antibody titers persist for at least 32 months after vaccination and slowly wane off. It is estimated that 85% protection is there 6–7 years after two doses of the vaccine [Citation41] and decreases to pre-vaccination level by 12 years without natural boosting [Citation42]. The antibody titers persist longer with natural boosting: for example, a study from Finland reported 74% seropositivity 20 years after MMR vaccination [Citation43]. A longitudinal study estimated the half-life of anti-mumps antibodies to more than 500 years (95% CI: 90 to infinity) and showed no significant decrease in titers over time [Citation3].
2.2.10. Pneumococcal conjugate vaccines (PCVs)
Though the exact duration of protection has yet to be established, PCVs provide reasonably good protection following a primary infantile schedule [Citation44]. There are few longitudinal studies to measure persistent immunity against pneumococcal diseases. Different experts have observed a marked variation in the protection rate of different serotypes. A study with PCV7 on nasopharyngeal carriage noticed sustained protection for five years [Citation45]. In South Africa, an experimental PCV, PCV9, showed consistent protection for 6.3 years after vaccination against Invasive Pneumococcal Disease (IPD) [Citation46].
2.2.11. Rabies vaccine
Vaccine-induced neutralizing antibody (VNA) after rabies vaccination is considered to be a valuable correlate of protection against rabies, and a titer of ≥ 0.5 IU is taken as a cutoff [Citation47]. Briggs et al. found that 80% of vaccinated subjects still had detectable VNA titer after nine years [Citation47]. Significantly, they did not find any correlation between the VNA titer and the number of vaccine doses received as a part of PrEP or PEP or the time elapsed since the last dose of the vaccine series. However, the intramuscular (IM) schedule induced neutralizing antibodies for a longer duration than the intradermal schedule [Citation48]. A German study of the longevity of vaccination-induced rabies VNA found detectable antibodies in 18 subjects 14 years after their last dose in the Pr-EP and PEP schedules [Citation49]. Furthermore, rabies vaccine induced a robust immune memory that provides excellent ‘immune recall’ even after several years of primary schedule [Citation50].
2.2.12. Herpes zoster recombinant vaccine
This new zoster vaccine is based on glycoprotein E of the varicella-zoster virus (VZV) and has an AS01B adjuvant system. The persistence of humoral immune response [anti-Glycoprotein E (gE) antibodies] and cellular immune response (gE-specific CD4+ T-cells) till six years after two doses of the vaccine has been demonstrated [Citation51]. Boutry et al. followed up with 7413 participants enrolled in the long-term follow-up study of the previous clinical trials to study the durability of vaccine efficacy. They found 84.1% efficacy after eight years of the second dose [Citation52].
2.2.13. Whole cell pertussis (wP)
Various studies have estimated the duration of protection offered by whole-cell pertussis (wP) vaccines, which ranges from 4 to 12 years [Citation53]. Long-term studies have shown a definite waning of protection after wP vaccines and natural infection over this period [Citation53–55]. A study by Campbell et al. from the UK cited 83.7% efficacy in children 10–16 years of age following three primary doses, though a decline in vaccine efficacy was noticed within 1–2 years of the third dose [Citation56]. According to a systematic review, annual loss in the efficacy of wP vaccines ranges from 2–13% [Citation57].
2.2.14. Pneumococcal polysaccharide vaccine (PPSV)
Pneumococcal polysaccharide vaccine is recommended in older adults and children with specific risk factors. On vaccination, the antibody levels decline over time after an initial rise and reach almost baseline levels within 4–7 years of vaccination [Citation58]. Extensive studies in older adults have shown vaccine effectiveness wanes over time, and effectiveness may be absent after five years. However, a large cohort study, mainly in adults over 65, has shown good protection against severe pneumococcal infections even after nine years [Citation59].
2.2.15. Haemophilus influenzae type b (hib) conjugate vaccine
Haemophilus influenzae type b (Hib) conjugate vaccine is highly immunogenic with an estimated efficacy of 95–100% after primary series [Citation60]. Most experts believe that Hib conjugate vaccine provides a long-lasting immunity despite waning in titers [Citation61]. However, no exact duration of protective immunity is known, and the need for a booster dose following the primary schedule is still uncertain [Citation62]. It is generally believed that the Hib conjugate vaccine protects at least for five years after completion of the primary series. Many countries have successfully controlled the Hib disease with a three-dose primary schedule, but some countries had to introduce a booster dose after a few years [Citation62,Citation63]. As per some studies, the protection offered by three doses in infancy lasts only till 2nd year of life and wanes quickly [Citation64,Citation65]. The anamnestic response to booster dose is excellent [Citation64].
2.3. Vaccines that provide short duration of protection (<5 years)
2.3.1. Meningococcal vaccines
Meningococcal polysaccharide vaccines, being T-cell independent vaccines, do not provide durable protection/memory-cell response. In younger children, 2–4 years of age, the protection lasts only a few months, while in older children, it lasts 2–3 years. Revaccination again protects for a similar duration [Citation66,Citation67].
Meningococcal conjugate vaccines offer better protection than polysaccharide vaccines, but even with these, the efficacy wanes over the years. The serological correlate of protection is serum bactericidal titer of ≥ 1:8 using baby rabbit complement (rSBA) [Citation68,Citation69]. Immunogenicity study in children aged 7–9 years has shown that seropositivity rates fall from 97.3% to 63.6% for serogroup A and 87.9% for other serogroups (C, W, and Y135) 2 years after vaccination and that falls to a mere 20% after six years [Citation70]. Cohn et al. have shown that the vaccine efficacy of a single dose falls from 79% (49% to 91%) at <1 year post-vaccination to 61% (25% to 79%) at 3 to <8 years post-vaccination [Citation71]. The waning in the protective antibody titers following meningococcal conjugate vaccines also varies with the child’s age, with waning faster in kids under five years of age than kids above five years of age [Citation70].
2.3.2. Acellular pertussis (aP) vaccines
Compared to wP vaccines, waning is faster with acellular pertussis (aP) vaccines. Schwartz et al. observed that the duration of protection following aP vaccines declined rapidly following four years of vaccination despite high early effectiveness [Citation72]. The efficacy drops from around 90% in the first year after the last dose of the aP vaccine to 30–40% 6–8 years after the last dose [Citation73–76]. Waning of protection after TdaP vaccines is even faster: some studies have shown the protection wanes quickly, with efficacy dropping to as low as 8.9% after four years [Citation77–80].
2.3.3. Dengue vaccines
Currently, two live attenuated dengue vaccines – CYD-TDV (Dengvaxia™ by Sanofi) and TAK-003 (Qdenga™ by Takeda) are licensed and approved for use in different populations in some countries. According to data from three efficacy trials, CYD-TDV offers protection for five years against severe dengue among seropositive individuals [Citation81]. Another live dengue vaccine, TAK-003, has been evaluated for 54 months. The published data showed a 62.0% efficacy against virologically confirmed dengue (VCD) and 83.6% against hospitalized VCD [Citation82]. According to unpublished follow-up data, the vaccine offered 64.2% vaccine efficacy against VCD in seropositive individuals and 53.5% VE in seronegative individuals after 54 months. The vaccine efficacy against hospitalized dengue was more than 80% after 54 months of follow-up [Citation83].
2.3.4. Cholera vaccines
Two types of cholera vaccines are available globally: monovalent and bi-valent-killed whole-cell oral cholera vaccines. While the prototype of the former is WC-rBS (recombinant B subunit of cholera toxin – Dukoral®), there are three different bi-valent vaccine products available globally that contain O1 and O139 strains (without the B subunit) and marketed as Shanchol™, Euvichol™ and mORCVAX™ All of them, barring mORCVAX™ (mainly used in Vietnam), are WHO-Pre-qualified [Citation84].
The estimated duration of protection of these cholera vaccines ranges from 6 months to 5 years. For the monovalent Dukoral™ vaccine, the protection lasts only six months for children and two years for adults. For the bivalent, whole-cell vaccine, Shanchol™, protection lasts up to 5 years after vaccination. In a large RCT conducted in Kolkata, the efficacy differed in different age groups – highest in older children and adults and lowest in young kids. The combined VE after five years was estimated to be 65% [Citation85].
2.3.5. Japanese encephalitis vaccines
Japanese encephalitis vaccine-live (SA-14-14-2): Studies from Nepal show that this live attenuated JE vaccine based on the SA-14-14-2 strain provides reasonably good protection for five years after a single dose. A case-control study in Nepalese children found 96.2% effectiveness after five years of a single dose [Citation86]. Another case-control study among adults finds 77.0% (95% CI: 67.0–83.0) effectiveness after seven years of a single dose administration in two districts of Assam, India [Citation87]. However, a recent cross-sectional study conducted in the Gorakhpur district of India finds that 97.74% of samples were negative for anti-JEV-specific IgG antibodies after two years of the second vaccine dose [Citation88].
Inactivated Vero cell-derived vaccines (IXIARO®/JESPECT® (Valneva, Austria) & JEEV®(BE, India): Data on the long-term protection against the clinical disease from the endemic region is limited. A Philippine study with IXIARO® among 300 children finds that 90% of un-boosted subjects had protective anti-JE antibodies after three years of primary vaccination of two doses [Citation89]. A 5-year follow-up study among two-dose recipients of the IXIARO vaccine in Austria finds that 81% of the subjects retained the protective antibody titers [Citation90]. Another study from the non-endemic region demonstrated the persistence of sero-protection for six years after two primary doses of IXIARO and a booster dose after 15 months [Citation91].
2.3.6. Typhoid vaccines
Vi-polysaccharide vaccine: A T-cell independent antigen, it does not induce memory cells and immunoglobulin class switching. Hence, it does not offer long-term protection and requires revaccination every three years for continued protection [Citation92]. Even within the three years, vaccine efficacy falls from 64% after 21 months to ~ 55% in the third year [Citation93].
Typhoid conjugate vaccines: Conjugate vaccines, being T-cell dependent, induce robust immune response with the production of memory cells, thus offering long-term protection. One study conducted with the vaccine developed with a nontoxic recombinant protein that is antigenically identical to Pseudomonas aeruginosa (Vi-rEPA conjugate vaccine) among two to five-year-old Vietnamese children demonstrated 89% efficacy after 27 weeks of active surveillance followed by 19 weeks of passive surveillance [Citation94]. Studies performed on another conjugate vaccine using tetanus toxoid as a carrier protein, Vi-TT (Typbar-TCV™), demonstrated that protection following a single dose might persist for up to five years [Citation95,Citation96]. A longitudinal study finds the persistence of anti-Vi-IgG antibodies up to 7 years following primary vaccination of children in both boosted and un-boosted cohorts. However, there was a significant waning in the titers after five years, and the manufacturer recommended a booster after five years of the primary dose [Citation96].
2.3.7. Herpes zoster live attenuated vaccine
This live attenuated vaccine gives protection for a shorter duration than the recombinant, inactivated vaccine. Efficacy against Herpes zoster and post-herpetic neuralgia hospitalization is higher and better maintained. A real-world effectiveness study demonstrated that herpes zoster vaccine efficiency decreased from 67% (95% CI: 65% to 69%) in the first year to 15% (5% to 24%) after ten years [Citation97]. Efficacy against post-herpetic neuralgia dropped from 83% (78% to 87%) to 41% (17% to 59%) during the same period. Vaccine efficacy against herpes zoster ophthalmicus decreased from 71% (63% to 76%) to 29% (18% to 39%), while that against herpes zoster hospitalization dropped from 90% (67% to 97%) to 53% (25% to 70%) in five to eight years [Citation97].
2.3.8. Rotavirus vaccines
Vaccine effectiveness of live attenuated rotavirus vaccines declines more rapidly in regions with high burden-high mortality than in industrialized countries having low burden-low morbidity [Citation98]. According to a meta-regression study, the efficacy of live oral rotavirus vaccines waned more rapidly in high-mortality settings, and pooled efficacy after 12 months was only 44%. In contrast, in low and middle mortality settings, the corresponding figure was 77%–94% [Citation99]. Some efficacy studies from the low burden-low low mortality countries have demonstrated sustained protection against severe rotavirus disease even after three years of primary vaccination [Citation100,Citation101].
2.3.9. Influenza vaccines
Vaccines against influenza do not produce long-term protection. After immunization, the anti-HA antibodies peak 2–4 weeks after vaccination in primed individuals, whereas in unprimed individuals and older adults, they peak ≥4 weeks after vaccination. After six months, the titers drop by more than 50%, with the amount of the drop proportional to the highest titers attained. The titers then stay constant for two to three years [Citation102]. According to clinical trial data, immunity against antigenically identical strains to those in the vaccination lasts for roughly 6–8 months [Citation103].
The vaccine effectiveness (VE) of the various influenza subtypes varies significantly. AH3 viruses have a more marked decrease in VE than AH1 viruses. Moreover, the VE is influenced by the interval since immunization. According to a comprehensive review and meta-analysis, VE for influenza virus subtypes A/H3 and type B is significantly less at 3–6 months after vaccination than 0–3 months after vaccination [Citation104].
A different study discovered that the influenza A(H3N2), influenza B, and influenza A(H1N1) pdm09 viruses caused a 7% (absolute) monthly drop in VE. Patients who had received influenza vaccinations in the previous season showed a more marked decline in VE [Citation105]. An Australian study discovered an intra-seasonal drop in influenza vaccine effectiveness. The study found that the best defense against influenza verified in a lab was when immunization was given within two months of the onset of symptoms [Citation106]. Vaccine effectiveness could disappear as soon as 90 days after inoculation, as per a 2018 analysis of 11 recent studies on the vaccine’s durability [Citation107].
2.3.10. SARS-CoV-2 vaccines
There is no known broad estimate of the duration of protection offered by the SARS-CoV-2 vaccines against COVID-19 disease, which varies according not only to the disease state and type but also to the circulating variants. Further, despite the extensive use of different vaccines and hundreds of studies over three years, no reliable immune correlate of protection against the disease could be ascertained so far. Overall, neither the infection nor the vaccines provide lasting protection. The effectiveness of these vaccines rapidly wanes over time after primary and booster vaccination. Most studies, including systematic reviews, found that protection waned significantly within 3–6 months of a vaccine dose, especially against the Omicron variant.
An extensive review evaluated 78 studies on vaccine-specific vaccine efficacy/effectiveness of four COVID-19 vaccines before the advent of the Omicron variant concluded that VE against symptomatic disease waned by 20–30% by six months of the primary series. However, the VE remained high, around 70% against severe disease, and declined only 10% after six months [Citation108].
Another review based on 40 peer-reviewed studies finds that the VE against the omicron variant was only 20% after six months of the primary series and 30% after nine months of a booster dose – a faster waning of VE noted against the Omicron than the Delta variant [Citation109].
Another review based on 41 studies on the performance of exclusively inactivated COVID-19 vaccines found that primary VE had waned significantly after six months [Citation110]. The study noted VE of 52.8% against the Delta variant, which went down sharply against the Omicron (16.4%). However, like mRNA vaccines, the VE against severe disease remained good even after six months of primary series, which improved after a booster dose [Citation110].
3. Immunological basis of long-term protection conferred by vaccines
Vaccines induce immune responses that are highly complex and include orchestrated actions of a wide range of immune cells in both innate and adaptive immune systems (). Ultimately, with most current vaccines, protection is provided through pathogen-specific antibodies, which target specific epitopes in pathogens or their toxic components. Antibodies are exclusively produced by a subset of B-lymphocytes (B-cells) called plasma cells. The process of antibody production begins with the vaccine antigens reaching the draining lymph nodes from the injection site, where they are captured by specialized macrophages in the sub-capsular region and then transported to B- -cell zone (follicles) (step ‘a’ in ). Here, B-cells are activated, and activated B cells migrate toward the border region between B-cell follicles and the T-cell zone (called marginal zone) [Citation111].
Figure 1. Schematic representation of vaccine-induced immune responses in the draining lymph node.
(Adapted from: Chen Z, Gao X, Yu D. Longevity of vaccine protection: Immunological mechanism, assessment methods, and improving strategy. View, 2022; 3, 20200103).
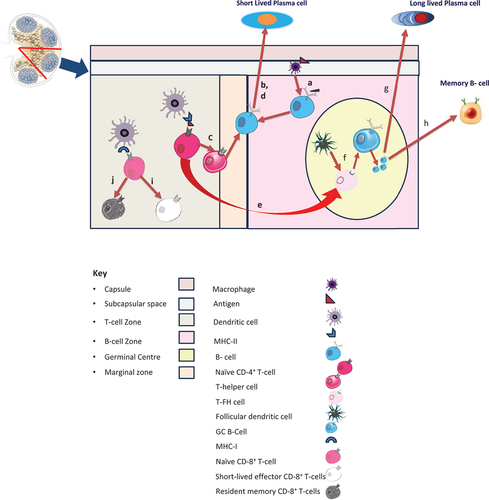
After that, there are two pathways: One, activated B-cells can induce extra-follicular response (without any help from T-cells), which leads to the production of short-lived plasma cells (step ‘b’ in Figure-1). Bacterial antigens like lipopolysaccharides induce this type of response. As the name suggests, these short-lived plasma cells secrete antibodies for a short time; thus, the vaccine-induced protection is only short duration. Without T-cell help, the antibodies produced are of low to modest affinity and mostly IgM type with no isotype switching [Citation111]. Of note, this reaction is relatively rapid, leading to the appearance of IgM antibodies within a few days of vaccination. Vi Polysaccharide typhoid vaccine is a classic example of this [Citation112].
The second pathway is the germinal center (GC) response induction. This pathway involves intricately coordinated activities in different lymph node zones and is relatively slower: it takes roughly two weeks for the germinal centers to develop [Citation113]. In the T-cell zone, the antigen is presented by major histocompatibility complex (MHC) class II of antigen-presenting cells (APC) like dendritic cells (DC) to naïve CD4+ T cells, leading to their functional differentiation to helper T cells (Th cells) (step ‘c’ in Figure-1). These Th cells provide helper signals to activate B-cells in the marginal zone to lead to extra-follicular response, producing short-lived plasma cells (step ‘d’ in ). These short-lasting plasma cells start dying after some time, resulting in reduced antibody titers noted 4–8 weeks after vaccination [Citation114]. However, because T-cells help in the form of type-1 cytokine interferon-gamma (IFN-γ) (produced by follicular helper T-cells (TFH cells) and Th1 cells) or Type 2 cytokines interleukin-4 (IL-4) [produced by TFH and Th2 cells], there is isotype switching to IgG1/IgG3 or IgG1 and IgE respectively. Some primed CD4+T-cells are committed to differentiation toward TFH cells (step ‘e’ in ), and they support the initiation and maintenance of GC reaction [Citation114,Citation115].
The GC reaction is initiated by the migration of some activated B cells toward germinal centers on getting signals from primed T-cells and follicular dendritic cells (FDCs) (step ‘f’ in ). The FDCs are essential here: they attract antigen-specific B and TFH cells and capture/retain antigens for extended periods. B cells attracted by antigen-bearing FDCs become the founders of the GC reaction. Each GC contains B-cells of a single antigen only. These B-cells undergo massive clonal proliferation, somatic hypermutation, and affinity maturation [selection of fittest B-cells (with highest affinity) after several ‘rounds’ of selection].
Moreover, they differentiate into long-lasting plasma cells (step ‘g’ in ) that secrete large amounts of high-affinity antibodies for extended periods (primary immune response) [Citation115]. Some GC B-cells differentiate into memory B cells (step ‘h’ in ). Memory cells persist as resting cells until re-exposed to the same antigen when they re-proliferate and differentiate into plasma cells, secreting large amounts of high-affinity antibodies (secondary immune response) [Citation115].
The decline of antibody titers after this secondary immune response is much slower than that of the primary immune response. Thus, a rapid increase in antibody titer is noted within days of infection with the same pathogen later or after a booster dose of the vaccine. During the GC reaction, a fraction of plasma cells can migrate toward long-term survival niches mainly located within the bone marrow, where their survival and antibody production may persist for years [Citation116]. The long persistence of antibodies can be predicted by measuring antibody titers between 6–12 months of vaccination, as by this time, all short-term plasma cells have disappeared, and in the absence of repeat vaccination, the antibodies in serum are those secreted by long-lasting plasma cells only [Citation117].
In addition to this antibody-mediated immune response (humoral immune response), CD8+ T cell-mediated cytolysis is a crucial mechanism of cellular immunity against intracellular infections. It is induced in the T-cell zone where naïve CD8+ T-cells are activated by cross-presentation of the antigen via the MHC class I molecules on dendritic cells that drive their differentiation into short-lived effector CD8+ T-cells (step ‘i’ in ) or resident memory CD8+ T-cells (step ‘j’ in ) [Citation118]. Like a humoral immune response, most short-lived cytotoxic effector cells enter programmed cell death at the end of primary immune responses. In contrast, memory CD8+ T cells persist over a more extended period, and they can rapidly proliferate into a massive wave of effector cells upon re-exposure to the same pathogen. In addition to protecting intracellular antigens, the memory function of CD8+ T cells has been suggested to be a significant protection mechanism for the elderly with inadequate antibody responses [Citation114].
Moreover, a new subset of CD8+ T cells that persist in non-lymphoid tissues for an extended period and can control reinfection has been recently identified. Termed tissue-resident memory T cells (TRM) they are of great interest for developing vaccines against infections entering through respiratory and digestive tracts where conventional antibody-based vaccinations cannot induce sufficient capacity to neutralize pathogens during initial infections [Citation119].
The critical determinants of the persistence of humoral immunity are two broad immune mechanisms – memory B-cell dependent and memory B-cell independent. The immunity in the latter is provided through antibodies produced by the long-lived plasma cells residing in the bone marrow niches [Citation120]. The memory B-cells themselves do not provide protection, but they divide and differentiate into plasma cells after antigenic exposure to secrete large amounts of antigen-specific antibodies [Citation3]. So, to assess the longevity of immunity induced by an immunogen, measuring the memory B-cell counts should provide an estimate of the durability of immunity. However, studies have shown that this correlation does not exist for all vaccines in real life. While in the case of specific antigens like measles and rubella, the memory B-cell population remains stable and correlates well with antibody levels decades later, whereas, in the case of some other antigens like varicella – zoster virus, Epstein – Barr virus, tetanus, or diphtheria, no such correlation exists [Citation3]. It is also noticed that this association fails to exist for those vaccines requiring multiple or repeated doses. This observation reflects that the mere persistence of memory B-cells may not guarantee the durability of antibodies, and some other mechanism may be involved in maintaining the long-term antibody levels. One such mechanism could be ‘imprinted lifespan’ phenomenon in which B-cell signaling during the induction of antigen-specific immune response determines the life span of the plasma cells [Citation121]. Most antigens induce memory B-cell formation, but the duration of antibody production is not the same. This hypothesis may explain why certain antigens provide long-term immunity while others provide only short-term protection.
During the process, the exact mechanisms responsible for differentiating immune cells toward different subsets and pathways are also poorly understood even today. However, the induction of good GC reactions, many long-lasting plasma cells, and the formation of memory B-cells and T-cells are essential for durable, long-lasting vaccine-induced protection [Citation115] ().
Figure 2. Key immunological determinants of durable vaccine-induced immune responses.
Both B-cell and T-cell responses help in providing durability to vaccine-induced immune responses. A. Germinal centers (encircled) in lymph nodes are factories of B-cell production and their maturation. B. Memory B cells in lymphoid tissues like the spleen. C. Long-lived plasma cells move to the bone marrow niches and survive there, providing long-lasting immunity.
D. Memory T-cells in the lamina propria and epithelium of the intestine. E. Tissue-resident memory T-cells (TRM) in the nasal passages and lungs. These cells are in non-lymphoid tissues, such as skin, gut, and lung airways, and do not recirculate through the blood. Most T cells mature in the thymus gland before subsequent export to the periphery.
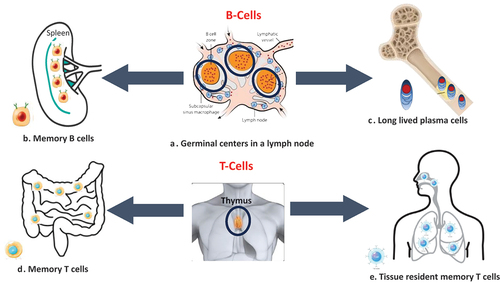
4. Determinants of the longevity of antibody response
Certain known factors determine how long the vaccine-induced antibody responses can be expected to last ():
Table 2. Factors determining durability of protective immunity.
4.1. Vaccine- and vaccination-related factors
4.1.1. Vaccine platform
The vaccine platform is crucial to determining longevity. Among all vaccination platforms, only live-attenuated and virus-like particle (VLP)-based vaccines elicit long-lasting antibody responses that can endure for several decades, or even a lifetime, without the need for further exposure to the antigen or reactivation of immunological memory. Amanna et al. conducted a long-term study on the levels of specific antibodies against viral antigens (vaccinia, measles, mumps, rubella, varicella-zoster virus, and Epstein-Barr virus) and non-replicating antigens (tetanus and diphtheria) in 45 individuals over up to 26 years. They discovered that the antibody responses against the viruses remained remarkably stable, with estimated half-lives ranging from 50 years for the varicella-zoster virus to over 200 years for other viruses like measles and mumps [Citation3]. The antibody responses to non-replicating antigens (tetanus and diphtheria) declined faster, with estimated half-lives of 11 years and 19 years, respectively [Citation3,Citation121]. Live vaccinations, in contrast to non-live antigens, cause activation of several lymph nodes due to the multiplication and spread of microbes [Citation117]. The process of microbial replication leads to a constant production of antigens, which in turn contributes to an intense and persistent immune response. VLP-based vaccines, such as the Human Papilloma Virus (HPV) vaccine, consist of supramolecular nanoparticles with highly repetitive antigen sequences displayed on their surfaces. The high antigen valency of VLPs, which refers to how densely the vaccine antigen is presented to the immune system, can significantly enhance early B-cell activation and the persistence of GC responses. This, in turn, leads to increased, long-lasting plasma cell differentiation and thus results in a durable and long-standing immune response [Citation122].
COVID-19 vaccines are attractive, as multiple vaccines have been developed, primarily based on its spike antigen, but on different platforms [Citation123]. For example, Moderna’s mRNA vaccine induces high titers of vaccine-induced binding and neutralizing antibodies and CD4 and CD8 cells. However, these wane off by six months, while Janssen’s vaccine, which is a virus vector (Adenovirus-26) based vaccine, induces antibody titers, CD4 and CD8 cells that have lower peak than mRNA vaccine, but persist longer (up to 8 months) [Citation124]. Further, studies have shown that boosting with a vaccine from a different platform (heterologous boosting) induces a better immune response than a homologous schedule [Citation125].
4.1.2. Antigen form
The form of the antigen influences the immune response. Particulate antigens, such as virus particles or aggregated proteins, stimulate more effective antigen uptake and migration by dendritic cells. They lead to more robust responses from CD4+ and CD8+ T cells than when the antigen is monomeric or soluble [Citation126]. Therefore, particle antigens and heat- or chemical-induced aggregated antigens elicit a more robust and long-lasting immune response than soluble, monomeric antigens. Nevertheless, several practical instances deviate from this principle. For instance, the recently approved RTS, S vaccine for malaria consists of a repeating, particulate antigen combined with a potent adjuvant [Citation127]. Nevertheless, this vaccine induces a protective immune response only in around 36% of individuals at vaccination, and the level of protection diminishes to 0% over three years [Citation128].
Another recent development in vaccinology is the ‘structure-based’ design of protein antigens (now termed ‘designer antigens’), which delineate the antigenic structures in the ‘pre-fusion’ and ‘post-fusion’ forms. The advent of new imaging techniques like X-ray crystallography and cryogenic electron microscopy (Cryo-EM) has made it possible to study these forms. Antibodies directed against the ‘post-fusion’ (after the antigen fuses with the host cell) shape do not thoroughly neutralize the circulating form of the virus before it binds to cells, known as the ‘prefusion’ form. Antibodies directed against the epitopes, exposed during only the pre-fusion form, are highly potent in neutralizing the virus. The knowledge about this concept led to the successful development of respiratory syncytial virus (RSV) vaccines and has also been employed in designing a few mRNA and protein-based COVID-19 vaccines [Citation129].
Antigen type is a crucial determinant of the longevity of immune responses elicited by a vaccine. Polysaccharide antigens are ‘T-cell independent’ and do not induce TFH cells and GC reaction in the absence of T-cell help (vide supra) [Citation117]. Thus, the antibody response to these antigens is predominantly IgM type, low titers of low-affinity IgG, and only of short-duration [Citation117]. Hence, the antibody response’s durability and ‘quality’ are suboptimal. Conjugation of polysaccharide antigen to a ‘carrier’ protein can lead to induction of TFH cells, GC reaction, and thus high-affinity antibody response with isotype switching and immune memory, leading to longer-term protection, as seen with Pneumococcal conjugate and typhoid conjugate vaccines [Citation130].
Adjuvants. Adjuvants improve the immune response to inactivated vaccines in many ways, such as modifying how the antigen is delivered, how long it persists (using depot or slow-release formulations), and boosting Th responses (immunomodulation) [Citation131]. Adjuvants can enhance the durability of vaccine-induced immunity by enhancing both the quality and intensity of immunological responses.
Antigen dose is also a factor as far as the durability of vaccine-induced immunity is concerned. An increase in antigen dose, in general, enhances immune response by increasing the availability of the antigen for B/T-cell binding. However, there is some complexity as the antigen dose is also shown to impact the induction of T-regulatory cells (Tregs) apart from affecting Th1/Th2 bias and recruitment of Th17 and TFH cells [Citation132].
The vaccination schedule also regulates the magnitude and duration of the antibody response. When priming doses are administered at intervals of less than one month, the resulting immune responses are less long-lasting than when the same number of vaccine doses are given at longer intervals (1-2 months). This is because fewer post-GC B cells are generated, which are capable of long-term survival. A shorter period between waves of primary reactions increases competition, negatively impacting durability. For the best memory retrieval and enhanced immune responses, it is necessary to have longer intervals of at least 3 to 4 months. The optimal timing for a booster dosage is at least 4-6 months after the last priming dose, allowing for the necessary affinity maturation of B-cells in the germinal center and creating memory cells [Citation117]. Administering the booster dose ‘prematurely’ leads to elevated antibodies at the boosting time, creating antigen-antibody complexes, which diminishes the antigens available for B-cell priming. Additionally, antibody-mediated feedback mechanisms may directly affect B cells negatively. The optimal schedule for non-live T-cell dependent immunizations that operate on the ‘prime-boost principle’ is ‘0, 1, 6 months’ [Citation117].
Route of administration. The immunological response to a vaccine can differ depending on the route of administration. For instance, Styles et al. showed in their animal study that intradermal vaccination led to better antigen retention in the lymph nodes than intramuscular administration, which resulted in a more robust and longer-lasting activation of antigen-specific GC T and B cells [Citation133]. Jegaskanda et al. showed that intranasal live-attenuated influenza vaccine, followed by a booster of intramuscular subunit vaccine, resulted in a robust immune response [Citation134]. Similarly, administering intramuscular mRNA-lipid nanoparticles as the initial dose, followed by intranasal protein vaccine as a booster, led to a strong and effective immune response against SARS-CoV2 [Citation124]. Hence, a combination of various platforms and delivery methods can enhance the durability of protection.
4.2. Vaccinee-related factors
Age at vaccination: The vaccinee’s age at the time of vaccination also affects vaccine antibody persistence, as the response is shorter at both extremes of age because of immaturity and senescence of the immune system, respectively [Citation117]. In infancy, the immune response to live attenuated vaccines is also adversely affected by preexisting antibodies transferred antenatally through the placenta [Citation135]. Even immune response to non-live vaccines is affected by these maternally-derived antibodies but to a lesser extent.
Gender: Although this aspect is not yet well studied, some indications indicate that immune response might also vary with gender. Studies have demonstrated that females elicit more exuberant immune responses to infections than males [Citation136,Citation137].
Genetic factors: Antigen epitopes associated with many MHC molecules are more likely to induce response. Gene polymorphisms in critical molecules required for B and T cell activation/differentiation molecules affect the quality of antibody responses. Individual and community variations of MHC molecules [human leukocyte antigen A2 (HLA A2)] affect T-cell responses significantly [Citation138].
Disease conditions: Certain disease conditions also affect vaccine antibody persistence. Increased catabolism (as in HIV) or urinary or digestive tract antibody loss reduces vaccination antibody persistence. Immunosuppressive medicines and conditions impact the durability of vaccine-induced protection. Recent studies suggest obesity may accelerate the waning of COVID-19 and influenza vaccine efficacy [Citation139].
Gut microbiome : Recent research reveals that the gut microbiome impacts the development of the immune system and immunological response to vaccines. The way by which the establishment of gut microbiome at/after birth affects childhood vaccination response is unknown [Citation140,Citation141].
4.3. Target-pathogen related factors
The target pathogen and its pathogenicity significantly influence the immune response and longevity. For instance, the incubation period and the force of infection transmission (the pace at which illness is acquired in a community) impact the effectiveness of vaccines. A lengthier incubation period allows for a more efficient memory response and enhanced long-term protection against symptomatic illness [Citation124]. This phenomenon has been extensively documented during the SARS-CoV-2 pandemic, wherein the level of protection against infection declined as the incubation periods of new strains of SARS-CoV-2 reduced [Citation142]. Moreover, it has been found that the effectiveness of vaccines is generally greater in regions where viral transmission, or the force of infection, is lower. This phenomenon was demonstrated in the case of a pentavalent rotavirus vaccine, which showed real-world efficiency ranging from 45% to 90% [Citation143]. OPV also exhibited significant variations in effectiveness [Citation144].
Licensed and experimental vaccines against viruses that replicate on mucosal surfaces without causing systemic infection/significant viremia also fail to induce durable protection. Natural infection with these viruses [like influenza, parainfluenza, RSV, SARS-CoV2, and other ‘human common cold viruses’] also does not provide long-term protection. The possible factors include: (a) They do not come in contact with multiple immune compartments and immune-competent cells, unlike other viruses like measles, mumps, rubella, smallpox, etc., (b) they have a shorter incubation period, as they start causing illness just after mucosal replication (the time required for systemic spread after mucosal replication is cut-off) [Citation1]. Due to the shorter incubation period, the immune system does not get adequate time to mount an adequate/robust immune response; (c) the half-life of nasal secretary IgA is much shorter than serum IgG. Thus, the viruses that induce mainly mucosal immune responses do not result in durable protection against these viruses [Citation145,Citation146]. There is another explanation from an evolutionary point of view: Unlike other immune compartments, mucosal surfaces regularly come in contact with a much larger number of antigens. Hence, they have evolved to ‘tolerate’ less dangerous antigens and down-regulate immune responses to them to minimize bystander tissue damage, focussing on more dangerous antigens for robust immune response. The mechanisms for this immune ‘decision-making’ have not been fully understood yet [Citation1]. Viral genetic variability (number and variety of viral strains/variants that are circulating) also affects vaccine efficacy adversely, as is readily observed with influenza and SARS-CoV2 viruses [Citation147,Citation148].
4.4. Other factors
Some other ‘environmental factors,’ largely unknown, might also affect vaccine-induced immune response. For example, there is a drastic difference in immune response to oral polio vaccine (OPV) among tropical and temperate countries [Citation144]. One hypothesis that explains this effect is that in tropical countries, multiple enteric pathogens are in circulation that affect the ‘uptake’ of the vaccine by the immune system [Citation149].
Even the time of day a vaccine is delivered has been shown to affect immune response robustness. The effect of the circadian clock on critical immune cell functions, including cytokine production, cell trafficking, dendritic cell activity, and T and B cell activity, has been shown; however, the exact mechanism of its effect on the immune response at the cellular or molecular level is still unclear [Citation150,Citation151].
5. How can we improve the durability of vaccine-induced protection?
5.1. Challenges and the way ahead
Despite the vaccines’ 225-year success in controlling infectious disease, improving quality of life, increasing life expectancy, and generating substantial economic benefits, it is ironic that we are still learning how the best vaccines work and how to improve vaccine design to improve protective efficacy. Most of the current vaccines have been empirically developed. Vaccine durability assessment is also a ‘wait-and-see’ method due to ignorance of immunological memory mechanisms and challenges in measuring cellular components that induce long-lasting immune protection [Citation152]. Recent advancements are beginning to reduce our knowledge gaps in underlying mechanisms of vaccine-mediated protection and long-term immunity. We may induce more effective and long-lasting immunity with fewer dosages by exploiting these nuances and optimizing immune response linkages.
Natural infection-induced immunity is usually more persistent than vaccine-induced protection. Exploring this disparity is one approach to developing more durable protection vaccines. Beukema et al. [Citation153] prolonged antigen delivery to replicate natural infection antigen dynamics to improve influenza vaccination durability in mice. They compared the immune response to standard prime-boost immunization with a 28-day interval to that of daily doses of whole-inactivated influenza virus vaccine for 14, 21, or 28 days. The 28 days of extended antigen administration achieved the highest cellular and humoral immune response levels, followed by 21 and 14 days of delivery and prime-boost immunization [Citation153]. Prolonging vaccine delivery also improved the quality of antibody response, as shown by significantly high levels of high avidity, balanced IgG subclass, and cross-reactive antibodies. Lee et al. found that long-prime, slow-delivery (12 days) priming of HIV envelope protein immunogen in rhesus monkeys led to more memory B-cells, higher antibody somatic hypermutation, and more robust immune responses to non-immunodominant epitopes [Citation154]. Further research is needed to identify the optimal antigen delivery methods and formulations that prolong antigen availability, elicit long-lasting and protective immunity against influenza and other viruses, and vaccines that provide long-term protection with fewer doses. Phase 1 of the HIV vaccine clinical trial on this concept is underway (NCT05471076) [Citation124].
Unlike unadjuvanted platforms or those with a single adjuvant, replicating viral vectors mimic natural infections and activate several receptors and inflammatory pathways to sustain immune responses. Therefore, early innate signals differ by pathogen or platform and may be surrogate endpoints to accelerate vaccine development and enable more durable protection [Citation124]. Immune correlates of protection by most modern vaccines are antibodies against pathogen antigens [Citation155]. Since the cellular response is difficult to measure, we have mainly focused on humoral antigen response. However, the quantitative analysis and functional characterization of CD4+ and CD8+ T-cell memory have advanced in the recent 2–3 decades. Multiple methods, such as MHC Class I tetramers, peptide-induced intracellular cytokine labeling, and IFNγ ELISPOT, can evaluate the frequency of antigen-specific T-cell responses [Citation156]. With advanced flow cytometry equipment and reagents, many T-cell functions and phenotypic markers can be evaluated simultaneously. These investigations and advanced microarray analysis of T-cell gene expression profiles have provided information on memory T-cell response [Citation126]. For instance, antiviral T-cell memory lasts decades after smallpox immunization [Citation157,Citation158] and SARS-CoV-1 infection [Citation159]. Despite all the information and the growing consensus that for the most challenging infectious diseases (e.g. AIDS, malaria, and tuberculosis), a combination of effective pathogen-specific humoral and cell-mediated immunity may be needed to create an effective vaccine, we are still far from harnessing cellular immunity. Identification and validation of T cell-specific correlates of immunity (or co-correlates of immunity of T cells and antibodies needed for vaccine-mediated protection) will go a long way in achieving tremendous success in this field.
New adjuvants should also be explored to prolong vaccine-induced protection. Inactivated and recombinant vaccines use adjuvants to boost vaccine-mediated immune responses, increasing seroconversion rates, antigen dose sparing, and fewer doses for similar protection. Alum salts, the most popular adjuvants, face the same issues as vaccines: their mechanism of action is still debated [Citation160]. Newer adjuvant systems may target innate immune system signaling pathways like Toll-like receptors (TLR) to direct vaccination-induced immunity and better match pathogen-specific immunity requirements (e.g. skewing of cellular vs. humoral immunity), resulting in a longer-lasting immune response. For example, AS04, a combination of MPL (monophosphoryl lipid A) (a TLR-4 agonist) and aluminum hydroxide, is used in an HPV vaccine, TCV™. This HPV vaccine Cervarix®, when compared to Cervarix®, on the same vaccine platform (Virus-like Particle, VLP) but with only an aluminum salt (aluminum hydroxy phosphate sulfate) as adjuvant, induced a 2- to 9-fold greater antibody response but had a similar serum-antibody decay showing that ASO4 does not substantially change humoral immune response kinetics [Citation161]. Thus, a better adjuvant system can help us achieve a ‘tailor-made’ immune response. Given the theoretical possibility of immune-mediated disease induction with such an adjuvant, caution is warranted toward safety when pursuing this approach.
6. Conclusion
Vaccination research and development have made great strides in the last two centuries, yet most vaccines have been developed empirically. We must still understand their mechanisms, especially the complicated interaction of innate, humoral, and cellular components and their subcomponents [Citation162]. Discoveries in this field are beginning to reduce our knowledge gaps. Similarly, bioengineering technologies are evolving rapidly. With nanoparticle and virus-like particle vaccinations, antigen valence and density are finely regulated. Antigen delivery can be controlled and sustained via newer biomaterials. New adjuvants, especially mucosal ones, can activate specific innate immune pathways. As the mechanisms of immune response durability become clearer, we can construct vaccines strategically to provide durable vaccine-induced protection with fewer doses.
7. Expert opinion
Vaccines have contributed immensely to the reduction of morbidity and mortality associated with infectious diseases, and ultimately reduction in infant/under-five mortality rates, Disability Life Years (DALY), school and work absenteeism, and thus rise in productivity, the standard of living and life expectancy, We have achieved a lot despite having only a handful of vaccines that provide long term (>20 years/lifelong) protection with one or two doses; vast majority of vaccines provide protection for 7–10 years, and often require multiple doses. Quite a few vaccines provide only short-term protection (<5 years). Enhancing longevity to the vaccine-induced protection would undoubtedly act as a ‘force multiplier’ in achieving all the benefits enumerated above. Further, by helping drastically reduce the cost of immunization programs, it has tremendous direct and indirect economic benefits to the nations, adding to the reputation of vaccines in particular and medical science in general.
The last few years have seen some rapid strides in the development of vaccines. The gestation period of developing a new vaccine has been cut down significantly – from the approval of a concept to the licensing of a product. Thanks mainly to the advent of new vaccine platforms, like nucleic acid-based mRNA and DNA vaccines, coupled with the advanced knowledge of biologically active forms of antigens and their interaction with the host cell, helped experts to design more effective and potent formulations, as shown in the successful development of RSV vaccines. Some progress in ensuring vaccine-induced immunity’s longevity is also there, but it is much slower. As discussed in our review, HPV vaccines shine out in this aspect. Based on the VLP vaccine platform, they have supramolecular nanoparticles with highly repetitive antigen sequences on their surfaces that significantly promote the early B-cell activation and the persistence of GC responses, leading to enhanced long-lasting plasma cell differentiation and durable immune response. Thus, despite being inactivated vaccines, they offer long-term protection, similar to live attenuated vaccines, without needing boosters. Many other strategies are currently being hotly researched to improve the durability of vaccine protection. Tweaking conventional immunization techniques such as long-prime, slow-delivery (12 days) immunization approach is one such strategy in this direction, as demonstrated in some animal studies with experimental HIV vaccine [Citation154] and influenza vaccine development [Citation153].
Most currently licensed vaccines have been developed empirically with a ‘develop and then see/assess’ approach. The need of the hour is to develop vaccines strategically. Undoubtedly, there are challenges regarding our understanding of immunology and the exact correlates of long-term protection. Thus, there is a need to identify ‘protective thresholds’ for different vaccine formulations and bio-markers of protective immunity so that minimum antibody levels should be maintained above the threshold to avoid a breakthrough infection [Citation107].
As elaborated in the review, the two vital immunological mechanisms that enhance the persistence of immune responses are memory B cell generations in the germinal centers and the survival of long-lived plasma cells in the bone marrow niches. There is a need to recognize factors that lead to the genesis and persistence of germinal centers apart from the nature and kinetics of their delivery. Similarly, identifying early innate signals that differ by agents and vaccine platforms could help develop and ensure the survival of long-lasting plasma cells in the bone marrow, providing a lasting immune response. Working on adjuvanted SARS-CoV-2 vaccine on non-human primates, Prabakaran et al. have demonstrated a sensitive method to identify and isolate antigen-specific long-lived plasma cells (LLPCs). Their published work has shown that spike protein vaccination can elicit and maintain LLPCs [Citation163].
Targeting specific epitopes or genes in antigenic sites conserved in different variants can also ensure the durability of protection. Further, there is a need to focus beyond the measurement of antibody production and target key mucosal sites to demonstrate tissue-generated memory cells at different mucosal tissues, which may help prevent breakthrough infections and ensure longevity.
Identifying solutions to these gaps in our understanding should form the future research agenda in the vaccinology field. We are confident that we are moving slowly but surely from an era of empirically developed vaccines to that of ‘designer vaccines,’ with particular emphasis on the durability of protection. In the coming years, the vaccine industry shall be able to resolve the current shortcomings with the help of advancements in biomaterials and bio-engineering for vaccine development.
Article highlights
Only a handful of currently licensed vaccines provide long-lasting protection
There are still gaps in our understanding of reliable correlates of durable protection.
Induction of vigorous germinal center reaction and induction of a large number of long-lasting plasma cells along with the formation of memory B-cells and memory T-cells is essential for durable vaccine-induced protection
There are several determinants of the longevity of antibody response, such as vaccine platform, target pathogen, vaccinee’s characteristics, environmental and genetic factors
New developments in the field of vaccinology, like improved vaccine design, novel adjuvants, and molecular delineation of antigenic forms, hold hope for developing durable vaccines
Further research is needed to identify the optimal antigen delivery methods and formulations that result in prolonged antigen availability and can elicit long-lasting and protective immunity
Declaration of interests
The authors have no relevant affiliations or financial involvement with any organization or entity with a financial interest in or financial conflict with the subject matter or materials discussed in the manuscript. This includes employment, consultancies, honoraria, stock ownership or options, expert testimony, grants or patents received or pending, or royalties.
Reviewer disclosures
Peer reviewers on this manuscript have no relevant financial or other relationships to disclose.
Author contributions
VMV and PK have substantially contributed to the conception and design of the review article and interpreting the relevant literature, and been involved in writing the review article or revised it for intellectual content.
Additional information
Funding
References
- Morens DM, Taubenberger JK, Fauci AS. Rethinking next-generation vaccines for coronaviruses, influenza viruses, and other respiratory viruses. Cell Host Microbe. 2023;31(1):146–157.
- Markowitz LE, Preblud SR, Fine PE, et al. Duration of live measles vaccine-induced immunity. Pediatr Infect Dis J. 1990; 9(2): 101–110. doi: 10.1097/00006454-199002000-00008
- Amanna IJ, Carlson NE, Slifka MK. Duration of humoral immunity to common viral and vaccine antigens. N Engl J Med. 2007;357(19):1903–1915.
- World Health Organization. Rubella vaccines: WHO position paper. Wkly Epidemiol Rec. 2020;95:301–324.
- Crooke SN, Riggenbach MM, Ovsyannikova IG, et al. Durability of humoral immune responses to rubella following MMR vaccination. Vaccine. 2020;38(51):8185–8193. doi: 10.1016/j.vaccine.2020.10.076
- Poland JD, Calisher CH, Monath TP, et al. Persistence of neutralizing antibody 30-35 years after immunization with 17D yellow fever vaccine. Bull World Health Organ. 1981;59(6):895–900.
- Reinhardt B, Jaspert R, Niedrig M, et al. Development of viremia and humoral and cellular parameters of immune activation after vaccination with yellow fever virus strain 17D: a model of human flavivirus infection. J Med Virol. 1998;56(2):159–67. doi: 10.1002/(SICI)1096-9071(199810)56:2<159:AID-JMV10>3.0.CO;2-B
- Groot H, Riberiro RB. Neutralizing and haemagglutination-inhibiting antibodies to yellow fever 17 years after vaccination with 17D vaccine. Bull World Health Organ. 1962;27(6):699–707.
- Niedrig M, Lademann M, Emmerich P, et al. Assessment of IgG antibodies against yellow fever virus after vaccination with 17D by different assays: neutralization test, haemagglutination inhibition test, immunofluorescence assay and ELISA. Trop Med Int Health. 1999 Dec;4(12):867–71.
- Coulange Bodilis H, Benabdelmoumen G, Gergely A, et al. Persistance à long terme des anticorps neutralisants de la fièvre jaune chez les personnes âgées de 60 ans et plus [Long term persistence of yellow fever neutralising antibodies in elderly persons]. Bull Soc Pathol Exot. 2011;104(4):260–265. doi: 10.1007/s13149-011-0135-7
- Marchesani R, Thomas N, Monath, et al. Comparative safety and immunogenicity of two yellow fever 17D vaccines (ARILVAX and YF-VAX) in a phase III multicenter, double-blind clinical trial. Am J Trop Med Hyg. 2002;66(5):533–541. doi: 10.4269/ajtmh.2002.66.533
- World Health Organization. WHO position paper on hepatitis a vaccines – October 2022. Wkly Epidemiol Rec. 2022;97:493–512.
- Espul C, Benedetti L, Cuello H, et al. Persistence of immunity from 1 year of age after one or two doses of hepatitis a vaccine given to children in Argentina. Hepat Med. 2012;4:53–60. doi: 10.2147/HMER.S33847
- Urueña A, Badano MN, Baré P, et al. Humoral and cellular immune memory response 12 years following single dose vaccination against hepatitis a in Argentinian children. Vaccine. 2022;40(1):114–121. doi: 10.1016/j.vaccine.2021.11.037
- Bianchi FP, Larocca AMV, Bozzi A, et al. Long-term persistence of poliovirus neutralizing antibodies in the era of polio elimination: An Italian retrospective cohort study. Vaccine. 2021;39(22):2989–2994. doi: 10.1016/j.vaccine.2021.04.005
- World Health Organization. Polio vaccines: WHO position paper. Weekly Epidemiol Rec. 2022;97:277–300.
- Larocca AMV, Bianchi FP, Bozzi A, et al. Long-term immunogenicity of inactivated and oral polio vaccines: an Italian retrospective cohort study. Vaccines (Basel). 2022 17;10(8):1329. doi: 10.3390/vaccines10081329
- Bhave S, Sapru A, Bavdekar A, et al. Long term immunogenicity of single dose of live attenuated hepatitis a vaccine in Indian children - results of 15-year follow-up. Indian Pediatr. 2021;58(8):749–752. doi: 10.1007/s13312-021-2285-4
- Chen Y, Zhou CL, Zhang XJ, et al. Immune memory at 17-years of follow-up of a single dose of live attenuated hepatitis a vaccine. Vaccine. 2018;36(1):114–121. doi: 10.1016/j.vaccine.2017.11.036
- Ott JJ, Irving G, Wiersma ST. Long-term protective effects of hepatitis a vaccines. A systematic review. Vaccine. 2012;31(1):3–11. doi: 10.1016/j.vaccine.2012.04.104
- World Health Organization. Human papillomavirus vaccines: WHO position paper (2022 update). Wkly Epidemiol Rec. 2022;97:645–672.
- Porras C, Tsang SH, Herrero R, et al. Efficacy of the bivalent HPV vaccine against HPV 16/18-associated precancer: long-term follow-up results from the Costa Rica vaccine trial. Lancet Oncol. 2020;21(12):1643–1652. doi: 10.1016/S1470-2045(20)30524-6
- Kjaer SK, Nygård M, Sundström K, et al. Final analysis of a 14-year long-term follow-up study of the effectiveness and immunogenicity of the quadrivalent human papillomavirus vaccine in women from four Nordic countries. E-Clin Med. 2020;23:100401. doi: 10.1016/j.eclinm.2020.100401
- Olsson SE, Restrepo JA, Reina JC, et al. Long-term immunogenicity, effectiveness, and safety of nine-valent human papillomavirus vaccine in girls and boys 9 to 15 years of age: interim analysis after 8 years of follow-up. Papillomavirus Res. 2020;10:100203. doi: 10.1016/j.pvr.2020.100203
- Kjaer SK, Nygård M, Sundström K, et al. Long-term effectiveness of the nine-valent human papillomavirus vaccine in Scandinavian women: interim analysis after 8 years of follow-up. Hum Vaccin Immunother. 2021;17(4):943–949. doi: 10.1080/21645515.2020.1839292
- Shilling H, Garland SM, Atchison S, et al. Human papillomavirus prevalence and risk factors among Australian women 9–12 years after vaccine program introduction. Vaccine. 2021;39(34):4856–4863. doi: 10.1016/j.vaccine.2021.07.005
- Aronson NE, Santosham M, Comstock GW, et al. Long-term efficacy of BCG vaccine in American Indians and Alaska Natives: a 60-year follow-up study. JAMA. 2004 5;291(17):2086–91. doi: 10.1001/jama.291.17.2086
- Nguipdop-Djomo P, Heldal E, Rodrigues LC, et al. Duration of BCG protection against tuberculosis and change in effectiveness with time since vaccination in Norway: a retrospective population-based cohort study. Lancet Infect Dis. 2016;16(2):219–26. doi: 10.1016/S1473-3099(15)00400-4
- Mangtani P, Nguipdop-Djomo P, Keogh RH, et al. The duration of protection of school-aged BCG vaccination in England: a population-based case-control study. Int J Epidemiol. 2018;47(1):193–201. doi: 10.1093/ije/dyx141
- Abubakar I, Pimpin L, Ariti C, et al. Systematic review and meta-analysis of the current evidence on the duration of protection by bacillus Calmette-Guérin vaccination against tuberculosis. Health Technol Assess. 2013;17(37):1–372, v–vi. 10.3310/hta17370
- World Health Organization. Diphtheria vaccine: WHO position paper. Weekly Epidemiol Rec. 2006;91:21–32.
- Bruce MG, Bruden D, Hurlburt D, et al. Antibody levels and protection after hepatitis B vaccine: results of a 30-year follow-up study and response to a booster dose. J Infect Dis. 2016;214(1):16–22. doi: 10.1093/infdis/jiv748
- World Health Organization. WHO Immunologic Basis For Immunization: Module 3: Tetanus. 2018 [accessed 2024 Mar 1]. Available from: https://www.who.int/publications/i/item/9789241513616
- World Health Organization. Tetanus vaccines: WHO position paper. Weekly Epidemiol Rec. 2017;92:53–76.
- Ferlito C, Biselli R, Mariotti S, et al. R. Tetanus-diphtheria vaccination in adults: the long-term persistence of antibodies is not dependent on polyclonal B-cell activation and the defective response to diphtheria toxoid re-vaccination is associated to HLADRB1*01. Vaccine. 2018;36(45):6718–6725. doi: 10.1016/j.vaccine.2018.09.041
- World Health Organization. Varicella and herpes zoster vaccines: WHO position paper, June 2014. Wkly Epidemiol Rec. 2014;89(25):265–287.
- Bayer O, Heininger U, Heiligensetzer C, et al. Metaanalysis of vaccine effectiveness in varicella outbreaks. Vaccine. 2007;25(37–38):6655–60. doi: 10.1016/j.vaccine.2007.07.010
- World Health Organization. Position paper. Mumps virus vaccines. Wkly Epidemiol Rec. 2007;7:51–60
- Vashishtha VM, Yadav S, Dabas A, et al. IAP position paper on burden of mumps in India and vaccination strategies. Indian Pediatr. 2015;52(6):505–14. doi: 10.1007/s13312-015-0666-2
- Demicheli V, Rivetti A, Debalini MG, et al. Vaccines for measles, mumps and rubella in children. Cochrane Database Syst Rev. 2012;2:CD004407. doi: 10.1002/14651858.CD004407.pub3
- Cohen C, White JM, Savage EJ, et al. Vaccine effectiveness estimates, 2004-2005 mumps outbreak, England. Emerg Infect Dis. 2007;13(1):12–17. doi: 10.3201/eid1301.060649
- LeBaron CW, Forghani B, Beck C, et al. Persistence of mumps antibodies after 2 doses of measles-mumps-rubella vaccine. J Infect Dis. 2009;199(4):552–560. doi: 10.1086/596207
- Davidkin I, Jokinen S, Broman M, et al. Persistence of measles, mumps, and rubella antibodies in an MMR-vaccinated cohort: a 20-year follow-up. J Infect Dis. 2008 1;197(7):950–6. doi: 10.1086/528993
- World Health Organization. Pneumococcal conjugate vaccines in infants and children under 5 years of age: WHO position paper – February 2019. Wkly Epidemiol Rec. 2019;94:85–104.
- Le Polain De Waroux O, Flasche S, Prieto-Merino D, et al. The efficacy and duration of protection of pneumococcal conjugate vaccines against nasopharyngeal carriage: a meta-regression model. Pediatric Infectious Disease Journal. 2015;34(8):858–864. doi: 10.1097/INF.0000000000000717
- Madhi SA, Klugman KP, Kuwanda L, et al. Quantitative and qualitative anamnestic immune responses to pneumococcal conjugate vaccine in HIV-infected and HIV-uninfected children 5 years after vaccination. J Infect Dis. 2009;199(8):1168–76. doi: 10.1086/597388
- World Health Organization. Rabies vaccines: WHO position paper – April 2018. Wkly Epidemiol Rec. 2018; 93:201–220. doi: 10.1016/j.vaccine.2018.06.061
- Briggs DJ, Schwenke JR. Longevity of rabies antibody titre in recipients of human diploid cell rabies vaccine. Vaccine. 1992;10(2):125–9. doi: 10.1016/0264-410X(92)90029-J
- Thraenhart O, Kreuzfelder E, Hillebrandt M, et al. Long-term humoral and cellular immunity after vaccination with cell culture rabies vaccines in man. Clin Immunol Immunopathol. 1994;71(3):287–92. doi: 10.1006/clin.1994.1088
- Mansfield KL, Andrews N, Goharriz H, et al. Rabies pre-exposure prophylaxis elicits long-lasting immunity in humans. Vaccine. 2016;34(48):5959–5967. doi: 10.1016/j.vaccine.2016.09.058
- Chlibek R, Pauksens K, Rombo L, et al. Long-term immunogenicity and safety of an investigational herpes zoster subunit vaccine in older adults. Vaccine. 2016 3;34(6):863–8. doi: 10.1016/j.vaccine.2015.09.073
- Boutry C, Hastie A, Diez-Domingo J, et al. The adjuvanted recombinant zoster vaccine confers long-term protection against herpes zoster: interim results of an extension study of the pivotal phase 3 clinical trials ZOE-50 and ZOE-70. Clin Infect Dis. 2022;74(8):1459–1467. doi: 10.1093/cid/ciab629
- Wendelboe AM, Van Rie A, Salmaso S, et al. Duration of immunity against pertussis after natural infection or vaccination. Pediatr Infect Dis J. 2005;24(5 Suppl):S58–61. doi: 10.1097/01.inf.0000160914.59160.41
- Vashishtha VM, Bansal CP, Gupta SG. Pertussis vaccines: position paper of Indian Academy of Pediatrics (IAP). Indian Pediatr. 2013;50(11):1001–9. doi: 10.1007/s13312-013-0274-y
- Jenkinson D Duration of effectiveness of pertussis vaccine: evidence from a 10-year community study. Br Med J (Clin Res Ed). 1988;296(6622):612–614. 10.1136/bmj.296.6622.612
- Campbell H, Amirthalingam G, Andrews N, et al. Accelerating control of pertussis in England and Wales. Emerg Infect Dis. 2012;18(1):38–47. doi: 10.3201/eid1801.110784
- Mueller J, Koutangni T, Guiso N, et al. Comparative efficacy/effectiveness of schedules in infant immunisation against pertussis, diphtheria and tetanus: systematic review and meta-analysis. Part 2: whole-cell pertussis vaccine. 2014 [accessed 2024 Jan 6]. Available from: http://www.who.int/immunization/sage/meetings/2015/april/6_Report_wP_140813.pdf?ua=1
- World Health Organization. WHO position paper-pneumococcal vaccines. Duration of Protection and Revaccination. [accessed 2023 Dec 10]. Available from: https://cdn.who.int/media/docs/default-source/immunization/position_paper_documents/pneumococcus/ppv23_additional_summary_duration_protection_revaccination.pdf?sfvrsn=ddf846b_3.
- Butler JC. Pneumococcal polysaccharide vaccine efficacy. An evaluation of current recommendations. JAMA. 1993;270(15):1826–1831. doi: 10.1001/jama.1993.03510150060030
- Sara E, Oliver SE, Moro P, et al. Epidemiology and prevention of vaccine-preventable diseases. Haemophilus influenzae [accessed 2024 Jan 5]. Available from: https://www.cdc.gov/vaccines/pubs/pinkbook/hib.html#:~:text=Hib%20conjugate%20vaccines%20are%20highly,children%20who%20are%20fully%20vaccinated
- World Health Organization. Haemophilus influenzae type b (hib) vaccination position paper – July 2013. Wkly Epidemiol Rec. 2013;88(39):413–426.
- von Gottberg A, Cohen C, Whitelaw A, et al.; Group for Enteric, Respiratory, Meningeal Disease Surveillance in South Africa (GERMS-SA). Invasive disease due to Haemophilus influenzae serotype b ten years after routine vaccination, South Africa, 2003-2009. Vaccine. 2012;30(3):565–571. doi: 10.1016/j.vaccine.2011.11.066.
- Ladhani S, Heath PT, Ramsay ME, et al. Long-term immunological follow-up of children with Haemophilus influenzae serotype b vaccine failure in the United Kingdom. Clin Infect Dis. 2009;49(3):372–80. doi: 10.1086/600292
- Sharma H, Yadav S, Lalwani S, et al. Antibody persistence of two pentavalent DTwP-HB-Hib vaccines to the age of 15-18 months, and response to the booster dose of quadrivalent DTwP-Hib vaccine. Vaccine. 2013;31(3):444–447. doi: 10.1016/j.vaccine.2012.11.038
- Gunardi H, Rusmil K, Fadlyana E, et al. DTwP-HB-Hib: antibody persistence after a primary series, immune response and safety after a booster dose in children 18-24 months old. BMC Pediatr. 2018;18(1):177. doi: 10.1186/s12887-018-1143-6
- Hu Z, Rou YL, Chen C, et al. An epidemiological and serological study on duration of protection after meningococcal group a polysaccharide (APS) vaccination. In: Poolman JT, editor. Gonococci and Meningococci. Dordrech: Springer; 1988. p. 199–207.
- Reingold AL, Broome CV, Hightower AW, et al. Age-specific differences in duration of clinical protection after vaccination with meningococcal polysaccharide A vaccine. Lancet. 1985;2 (8447):114–118. doi: 10.1016/S0140-6736(85)90224-7
- Andrews N, Borrow R, Miller E Validation of serological correlate of protection for meningococcal C conjugate vaccine by using efficacy estimates from post-licensure surveillance in England. Clin Diagn Lab Immunol. 2003;10(5):780–786. doi: 10.1128/CDLI.10.5.780-786.2003
- Borrow R, Andrews N, Goldblatt D, et al. Serological basis for use of meningococcal serogroup C conjugate vaccines in the United Kingdom: reevaluation of correlates of protection. Infect Immun. 2001;69(3):1568–73. doi: 10.1128/IAI.69.3.1568-1573.2001
- Nolan T, Booy R, Marshall HS, et al. Immunogenicity and safety of a quadrivalent meningococcal ACWY-tetanus toxoid conjugate vaccine 6 years after MenC priming as toddlers. Pediatr Infect Dis J. 2019;38(6):643–650. doi: 10.1097/INF.0000000000002334
- Cohn AC, MacNeil JR, Harrison LH, et al. Active bacterial core surveillance (ABCs) team and MeningNet surveillance partners. Effectiveness and duration of protection of one dose of a meningococcal conjugate vaccine. Pediatrics. 2017;139(2):e20162193. doi: 10.1542/peds.2016-2193
- Schwartz KL, Kwong JC, Deeks SL, et al. Effectiveness of pertussis vaccination and duration of immunity. CMAJ. 2016;188(16):E399–E406. doi: 10.1503/cmaj.160193
- Mills KH Immunity to Bordetella pertussis. Microbes Infect. 2001;3(8):655–677. doi: 10.1016/S1286-4579(01)01421-6
- Klein NP, Bartlett J, Rowhani-Rahbar A, et al. Waning protection after fifth dose of acellular pertussis vaccine in children. N Engl J Med. 2012;367(11):1012–1019. doi: 10.1056/NEJMoa1200850
- Witt MA, Katz PH, Witt DJ. Unexpectedly limited durability of immunity following acellular pertussis vaccination in preadolescents in a North American outbreak. Clin Infect Dis. 2012;54(12):1730–5. doi: 10.1093/cid/cis287
- Bell CA, Russell ML, Drews SJ, et al. Acellular pertussis vaccine effectiveness and waning immunity in Alberta, Canada: 2010-2015, a Canadian Immunization Research Network (CIRN) study. Vaccine. 2019;37(30):4140–4146. doi: 10.1016/j.vaccine.2019.05.067
- Klein NP, Bartlett J, Fireman B, et al. Waning Tdap Effectiveness in Adolescents. Pediatrics. 2016;137(3):e20153326. doi: 10.1542/peds.2015-3326
- Koepke R, Eickhoff JC, Ayele RA, et al. Estimating the effectiveness of tetanus-diphtheria-acellular pertussis vaccine (Tdap) for preventing pertussis: evidence of rapidly waning immunity and difference in effectiveness by Tdap brand. J Infect Dis. 2014;210(6):942–53. doi: 10.1093/infdis/jiu322
- Acosta AM, DeBolt C, Tasslimi A, et al. Tdap vaccine effectiveness in adolescents during the 2012 Washington State pertussis epidemic. Pediatrics. 2015;135(6):981–9. doi: 10.1542/peds.2014-3358
- Briere EC, Pondo T, Schmidt M, et al. Assessment of Tdap vaccination effectiveness in adolescents in integrated health-care systems. J Adolesc Health. 2018;62(6):661–666. doi: 10.1016/j.jadohealth.2017.12.011
- Sridhar S, Luedtke A, Langevin E, et al. Effect of Dengue Serostatus on Dengue vaccine safety and efficacy. N Engl J Med. 2018;379(4):327–340. doi: 10.1056/NEJMoa1800820
- Rivera L, Biswal S, Sáez-Llorens X, et al. Three-year efficacy and safety of Takeda’s dengue vaccine Candidate (TAK-003). Clin Infect Dis. 2022;75(1):107–117. doi: 10.1093/cid/ciab864
- Tricou V Efficacy and safety of Takeda’s tetravalent dengue vaccine Candidate (TAK-003) after 4.5 years of follow-up. Presented at 8th Northern European Conference of Travel Medicine; Jun 2022 [accessed 2023 Dec 12]. Available from: https://assets-dam.takeda.com/raw/upload/v1668754472/legacy-dotcom/siteassets/system/what-we-do/areas-of-focus/vaccines/info/tides-fact-sheet.pdf
- World Health Organization. Cholera vaccines: WHO position paper–August 2017. Wkly Epidemiol Rec. 2017;92(34):477–498.
- Bhattacharya SK, Sur D, Ali M, et al. 5-year efficacy of a bivalent killed whole-cell oral cholera vaccine in Kolkata, India: a cluster-randomised, double-blind, placebo-controlled trial. Lancet Infect Dis. 2013;13(12):1050–1056. doi: 10.1016/S1473-3099(13)70273-1
- Tandan JB, Ohrr H, Sohn YM, et al. Single dose of SA 14-14-2 vaccine provides long-term protection against Japanese encephalitis: a case-control study in Nepalese children 5 years after immunization. Vaccine. 2007; 25(27):5041–5045. doi: 10.1016/j.vaccine.2007.04.052
- Khan SA, Choudhury P, Kakati S, et al. Effectiveness of a single dose of Japanese encephalitis vaccine among adults, Assam, India, 2012-2018. Vaccine. 2021;39(35):4973–4978. doi: 10.1016/j.vaccine.2021.07.041
- Preethi L, Alina MS, Chandran L, et al. Duration of seroprotection of the live attenuated SA-14-14-2 Japanese encephalitis vaccine in children in India. J Travel Med. 2023;30(2):taac147. doi: 10.1093/jtm/taac147
- Kadlecek V, Borja-Tabora CF, Eder-Lingelbach S, et al. Antibody persistence up to 3 years after primary immunization with inactivated Japanese encephalitis vaccine IXIARO in Philippine children and effect of a booster dose. Pediatr Infect Dis J. 2018;37(9):e233–e240. doi: 10.1097/INF.0000000000002124
- Taucher C, Kollaritsch H, Dubischar KL. Persistence of the immune response after vaccination with the Japanese encephalitis vaccine, IXIARO® in healthy adults: a five year follow-up study. Vaccine. 2019;37(19):2529–2531. doi: 10.1016/j.vaccine.2019.03.030
- Paulke-Korinek M, Kollaritsch H, Kundi M, et al. Persistence of antibodies six years after booster vaccination with inactivated vaccine against Japanese encephalitis. Vaccine. 2015;33(30):3600–4. doi: 10.1016/j.vaccine.2015.05.037
- World Health Organization. Typhoid vaccines: WHO position paper – March 2018. Wkly Epidemiol Rec. 2018;93:153–172.
- Klugman KP, Koornhof HJ, Robbins JB, et al. Immunogenicity, efficacy and serological correlate of protection of salmonella typhi vi capsular polysaccharide vaccine three years after immunization. Vaccine. 1996;14(5):435–8. doi: 10.1016/0264-410X(95)00186-5
- Lin FY, Ho VA, Khiem HB, et al. The efficacy of a Salmonella typhi Vi conjugate vaccine in two-to-five-year-old children. N Engl J Med. 2001;344(17):1263–1269.10.1056/NEJM200104263441701
- Voysey M, Pollard AJ Seroefficacy of Vi polysaccharide-tetanus toxoid typhoid conjugate vaccine (Typbar TCV). Clin Infect Dis. 2018;67(1):18–24. doi: 10.1093/cid/cix1145
- Vadrevu KM, Raju D, Rani S, et al. Persisting antibody responses to Vi polysaccharide-tetanus toxoid conjugate (Typbar TCV®) vaccine up to 7 years following primary vaccination of children < 2 years of age with, or without, a booster vaccination. Vaccine. 2021 Oct 29;39(45):6682–6690. doi: 10.1016/j.vaccine.2021.07.073
- Klein NP, Bartlett J, Fireman B, et al. Effectiveness of the live zoster vaccine during the 10 years following vaccination: real world cohort study using electronic health records. BMJ. 2023;383:e076321. doi: 10.1136/bmj-2023-076321
- World Health Organization. Rotavirus vaccines: WHO position paper - July 2021. Weekly Epidemiological Rec, 2021;96:301–319.
- Clark A, van Zandvoort K, Flasche S, et al. Efficacy of live oral rotavirus vaccines by duration of follow-up: a meta-regression of randomised controlled trials. Lancet Infect Dis. 2019;19(7):717–727. doi: 10.1016/S1473-3099(19)30126-4
- Vesikari T, Karvonen A, Ferrante SA, et al. Efficacy of the pentavalent rotavirus vaccine, RotaTeq®, in Finnish infants up to 3 years of age: the Finnish extension study. Eur J Pediatr. 2010;169(11):1379–86. doi: 10.1007/s00431-010-1242-3
- Phua KB, Lim FS, Lau YL, et al. Rotavirus vaccine RIX4414 efficacy sustained during the third year of life: a randomized clinical trial in an Asian population. Vaccine. 2012;30(30):4552–7. doi: 10.1016/j.vaccine.2012.03.030
- Vashishtha VM, Kalra A, Choudhury P. Influenza vaccination in India: position paper of Indian Academy of Pediatrics, 2013. Indian Pediatr. 2013;50(9):867–74. doi: 10.1007/s13312-013-0230-x
- Saha S, Chadha M, Shu Y, et al. Divergent seasonal patterns of influenza types a and B across latitude gradient in tropical asia. Influenza Other Respir Viruses. 2016;10(3):176–184. doi: 10.1111/irv.12372
- Young B, Sadarangani S, Jiang L, et al. Duration of Influenza vaccine effectiveness: a systematic review, meta-analysis, and meta-regression of test-negative design case-control studies. J Infect Dis. 2018;217(5):731–741. doi: 10.1093/infdis/jix632
- Ferdinands JM, Fry AM, Reynolds S, et al. Intraseason waning of influenza vaccine protection: evidence from the US influenza vaccine effectiveness network, 2011-12 through 2014-15. Clin Infect Dis. 2016;64(5):544–550. doi: 10.1093/cid/ciw816
- Regan AK, Fielding JE, Chilver MB, et al. Intra-season decline in influenza vaccine effectiveness during the 2016 southern hemisphere influenza season: a test-negative design study and phylogenetic assessment. Vaccine. 2019;37(19):2634–2641. doi: 10.1016/j.vaccine.2019.02.027
- Rambhia KJ, Rambhia MT. Early bird gets the flu: what should be done about waning intraseasonal immunity against seasonal influenza? Clin Infect Dis. 2019;68(7):1235–1240. doi: 10.1093/cid/ciy748
- Feikin DR, Higdon MM, Abu-Raddad LJ, et al. Duration of effectiveness of vaccines against SARS-CoV-2 infection and COVID-19 disease: results of a systematic review and meta-regression. Lancet. 2022;399(10328):924–944. doi: 10.1016/S0140-6736(22)00152-0
- Menegale F, Manica M, Zardini A, et al. Evaluation of waning of SARS-CoV-2 vaccine-induced immunity: a systematic review and meta-analysis. JAMA Netw Open. 2023;6(5):e2310650. doi: 10.1001/jamanetworkopen.2023.10650
- Xu S, Li J, Wang H, et al. Real-world effectiveness and factors associated with effectiveness of inactivated SARS-CoV-2 vaccines: a systematic review and meta-regression analysis. BMC Med. 2023;21(1):160. doi: 10.1186/s12916-023-02861-3
- Pulendran B, Ahmed R. Immunological mechanisms of vaccination. Nat Immunol. 2011;12(6):509–17. doi: 10.1038/ni.2039
- Kamat D, Mathur A. Vaccine immunology. In: Vashishtha V Kalra A (editors) IAP Textbook of Vaccines. 2nd ed. New Delhi: Jaypee Brothers Medical Publishers; 2020. p. 31–44.
- Elsner RA, Shlomchik MJ. Germinal Center and Extrafollicular B Cell Responses in Vaccination, Immunity, and Autoimmunity. Immunity. 2020;53(6):1136–1150. doi: 10.1016/j.immuni.2020.11.006
- Chen Z, Gao X, Yu D. Longevity of vaccine protection: immunological mechanism, assessment methods, and improving strategy. View. 2022;3(1):20200103.
- Stebegg M, Kumar SD, Silva-Cayetano A, et al. Regulation of the germinal center response. Front Immunol. 2018;9:2469. doi: 10.3389/fimmu.2018.02469
- Elgueta R, de Vries VC, Noelle RJ The immortality of humoral immunity. Immunol Rev. 2010;236(1):139–150. doi: 10.1111/j.1600-065X.2010.00924.x
- Siegrist C-A. Vaccine immunology. In: Plotkin S, Orenstein W, Offit P, editors. Vaccins. 6th ed. Philadelphia, PA: Elsevier-Saunders; 2013; p. 14–32.
- Chaplin DD. Overview of the immune response. J Allergy Clin Immunol. 2010;125(2 Suppl 2):S3–23. doi: 10.1016/j.jaci.2009.12.980
- Wu X, Wu P, Shen Y, et al. CD8+ resident memory T cells and viral infection. Front Immunol. 2018;9:2093. doi: 10.3389/fimmu.2018.02093
- Amanna IJ, Slifka MK, Crotty S Immunity and immunological memory following smallpox vaccination. Immunol Rev. 2006;211 1:320–337. doi: 10.1111/j.0105-2896.2006.00392.x
- Amanna IJ, Slifka MK. Mechanisms that determine plasma cell lifespan and the duration of humoral immunity. Immunol Rev. 2010;236(1):125–138. doi: 10.1111/j.1600-065X.2010.00912.x
- Kato Y, Abbott RK, Freeman BL, et al. Multifaceted effects of antigen Valency on B cell response composition and differentiation in vivo. Immunity. 2020;53(3):548–563.e8. doi: 10.1016/j.immuni.2020.08.001
- Heinz FX, Stiasny K. Distinguishing features of current COVID-19 vaccines: knowns and unknowns of antigen presentation and modes of action. NPJ Vaccin. 2021;6(1):104. doi: 10.1038/s41541-021-00369-6
- Palin AC, Alter G, Crotty S, et al. The persistence of memory: defining, engineering, and measuring vaccine durability. Nat Immunol. 2022;23(12):1665–1668. doi: 10.1038/s41590-022-01359-z.
- Kumar P. “Mix and match” of COVID vaccines (heterologous boosters) In: Vashishtha V, Kumar P, and Wadhwa A, editors. COVID-19 vaccines a comprehensive review. 1st ed. Mumbai: Tree Life Media Publishers, 2022: p. 269–279.
- Slifka MK, Amanna I. How advances in immunology provide insight into improving vaccine efficacy. Vaccine. 2014;32(25):2948–57. doi: 10.1016/j.vaccine.2014.03.078
- Gordon DM, McGovern TW, Krzych U, et al. Safety, immunogenicity, and efficacy of a recombinant produced Plasmodium falciparum circumsporozoite protein-hepatitis B surface antigen subunit vaccine. J Infect Dis. 1995;171(6):1576–1585. doi: 10.1093/infdis/171.6.1576
- Bejon P, White MT, Olotu A, et al. Efficacy of RTS, S malaria vaccines: individual-participant pooled analysis of phase 2 data. Lancet Infect Dis. 2013;13(4):319–327. doi: 10.1016/S1473-3099(13)70005-7
- Graham BS, Gilman MSA, McLellan JS. Structure-Based Vaccine Antigen Design. Annu Rev Med. 2019 Jan 27;70(1):91–104. doi: 10.1146/annurev-med-121217-094234
- Bugya Z, Prechl J, Szénási T, et al. Multiple levels of immunological memory and their association with vaccination. Vaccines (Basel). 2021 Feb 19;9(2):174. doi: 10.3390/vaccines9020174
- Awate S, Babiuk LA, Mutwiri G. Mechanisms of action of adjuvants. Front Immunol. 2013;4:114. doi: 10.3389/fimmu.2013.00114
- Billeskov R, Beikzadeh B, Berzofsky JA. The effect of antigen dose on T cell-targeting vaccine outcome. Hum Vaccin Immunother. 2019;15(2):407–411. doi: 10.1080/21645515.2018.1527496
- Styles TM, Gangadhara S, Reddy PBJ, et al. V2 hotspot optimized MVA vaccine expressing stabilized HIV-1 Clade C envelope Gp140 delays acquisition of heterologous Clade C Tier 2 challenges in Mamu-A*01 negative Rhesus Macaques. Front Immunol. 2022;13:914969. doi: 10.3389/fimmu.2022.914969
- Jegaskanda S, Mason RD, Andrews SF, et al. Intranasal Live Influenza Vaccine Priming Elicits Localized B Cell Responses in Mediastinal Lymph Nodes. J Virol. 2018;92(9):e01970–17. doi: 10.1128/JVI.01970-17
- Mok DZL, Chan KR. The effects of pre-existing antibodies on live-attenuated viral vaccines. Viruses. 2020;12(5):520. doi: 10.3390/v12050520
- Ciarambino T, Para O, Giordano M. Immune system and COVID-19 by sex differences and age. Womens Health. 2021;17:17455065211022262. doi: 10.1177/17455065211022262
- Cheung F, Apps R, Dropulic L, et al. Sex and prior exposure jointly shape innate immune responses to a live herpesvirus vaccine. Elife. 2023;12:e80652. doi: 10.7554/eLife.80652
- Cruz-Tapias P, Castiblanco J, Anaya JM, et al. Major histocompatibility complex: antigen processing and presentation. In: Anaya J, Shoenfeld Y Rojas-Villarraga A, editors. Autoimmunity: from bench to bedside [internet]. Bogota (Colombia): El Rosario University Press; 2013. Chapter 10. Available from: https://www.ncbi.nlm.nih.gov/books/NBK459467/
- van der Klaauw AA, Horner EC, Pereyra-Gerber P, et al. Accelerated waning of the humoral response to COVID-19 vaccines in obesity. Nat Med. 2023;29(5):1146–1154. doi: 10.1038/s41591-023-02343-2
- Jordan A, Carding SR, Hall LJ. The early-life gut microbiome and vaccine efficacy. Lancet Microbe. 2022;3(10):e787–e794. doi: 10.1016/S2666-5247(22)00185-9
- Moroishi Y, Gui J, Nadeau KC, et al. A prospective study of the infant gut microbiome in relation to vaccine response. Pediatr Res. 2023;93(3):725–731. doi: 10.1038/s41390-022-02154-0
- Xu X, Wu Y, Kummer AG, et al. Assessing changes in incubation period, serial interval, and generation time of SARS-CoV-2 variants of concern: a systematic review and meta-analysis. BMC Med. 2023;21(1):374. doi: 10.1186/s12916-023-03070-8
- Varghese T, Kang G, Steele AD. Understanding rotavirus vaccine efficacy and effectiveness in countries with high child mortality. Vaccines (Basel). 2022;10(3):346. doi: 10.3390/vaccines10030346
- Parker EP, Ramani S, Lopman BA, et al. Causes of impaired oral vaccine efficacy in developing countries. Future Microbiol. 2018;13(1):97–118. doi: 10.2217/fmb-2017-0128
- Reuman PD, Bernstein DI, Keely SP, et al. Influenza-specific ELISA IgA and IgG predict severity of influenza disease in subjects prescreened with hemagglutination inhibition. Antiviral Res. 1990;13(3):103–10. doi: 10.1016/0166-3542(90)90026-4
- Lavelle EC, Ward RW. Mucosal vaccines — fortifying the frontiers. Nat Rev Immunol. 2022;22(4):236–250. doi: 10.1038/s41577-021-00583-2
- Nuwarda RF, Alharbi AA, Kayser V. An overview of influenza viruses and vaccines. Vaccines (Basel). 2021;9(9):1032. doi: 10.3390/vaccines9091032
- Carabelli AM, Peacock TP, Thorne LG, et al. SARS-CoV-2 variant biology: immune escape, transmission and fitness. Nat Rev Microbiol. 2023;21:162–177. doi: 10.1038/s41579-022-00841-7
- Patriarca PA, Wright PF, John TJ. Factors affecting the immunogenicity of oral poliovirus vaccine in developing countries: review. Rev Infect Dis. 1991;13(5):926–39. doi: 10.1093/clinids/13.5.926
- Otasowie CO, Tanner R, Ray DW, et al. Chronovaccination: Harnessing circadian rhythms to optimize immunisation strategies. Front Immunol. 2022;13:977525. doi: 10.3389/fimmu.2022.977525
- Cervantes-Silva MP, Carroll RG, Wilk MM, et al. The circadian clock influences T cell responses to vaccination by regulating dendritic cell antigen processing. Nat Commun. 2022;13(1):7217. doi: 10.1038/s41467-022-34897-z
- Cohen J. How long do vaccines last? The surprising answers may help protect people longer. Science Magazine [accessed 2023 Dec 23]. Available from: https://www.science.org/content/article/how-long-do-vaccines-last-surprising-answers-may-help-protect-people-longer
- Beukema M, Gong S, Al-Jaawni K, et al. Prolonging the delivery of influenza virus vaccine improves the quantity and quality of the induced immune responses in mice. Front Immunol. 2023;14:1249902. doi: 10.3389/fimmu.2023.1249902
- Lee JH, Sutton HJ, Cottrell CA, et al. Long-primed germinal centres with enduring affinity maturation and clonal migration. Nature. 2022;609(7929):998–1004. doi: 10.1038/s41586-022-05216-9
- Plotkin SA. Vaccines: correlates of vaccine-induced immunity. Clin Infect Dis. 2008 1;47(3):401–409. doi: 10.1086/589862.
- Farber DL, Yudanin NA, Restifo NP. Human memory T cells: generation, compartmentalization and homeostasis. Nat Rev Immunol. 2014;14(1):24–35. doi: 10.1038/nri3567
- Hammarlund E, Lewis MW, Hansen SG, et al. Duration of antiviral immunity after smallpox vaccination. Nature Med. 2003;9(9):1131–1137. doi: 10.1038/nm917
- Crotty S, Felgner P, Davies H, et al. Cutting edge: long-term B cell memory in humans after smallpox vaccination. J Immunol. 2003;171(10):4969–4973. doi: 10.4049/jimmunol.171.10.4969
- Duan LJ, Cui XM, Zhu KL, et al. SARS-CoV-2 vaccine-induced antibody and T cell response in SARS-CoV-1 survivors. Cell Rep. 2022;40(9):111284. doi: 10.1016/j.celrep.2022.111284
- McKee AS, Munks MW, MacLeod MK, et al. Alum induces innate immune responses through macrophage and mast cell sensors, but these sensors are not required for alum to act as an adjuvant for specific immunity. J Immunol. 2009;183(7):4403–4414. 10.4049/jimmunol.0900164
- Einstein MH, Baron M, Levin MJ, et al. Comparative immunogenicity and safety of human papillomavirus (HPV)-16/18 vaccine and HPV-6/11/16/18 vaccine: follow-up from months 12–24 in a phase III randomized study of healthy women aged 18–45 years. Hum Vaccin. 2011;7(12):1343–1358. doi: 10.4161/hv.7.12.18281
- Pollard AJ, Bijker EM. A guide to vaccinology: from basic principles to new developments. Nat Rev Immunol. 2021;21(2):83–100. doi: 10.1038/s41577-020-00479-7
- Prabhakaran M, Matassoli F, Leggat D, et al. Adjuvanted SARS-CoV-2 spike protein vaccination elicits long-lived plasma cells in nonhuman primates. Sci Transl Med. 2024;16(728):eadd5960. doi: 10.1126/scitranslmed.add5960