ABSTRACT
Background
The question of whether influenza vaccine effectiveness (VE) wanes over the winter season is still open and some contradictory findings have been reported. This study investigated the possible decline in protection provided by the available influenza vaccines.
Research design and methods
An individual-level pooled analysis of six test-negative case-control studies conducted in Italy between the 2018/2019 and 2022/2023 seasons was performed. Multivariable logistic regression analyses were performed to estimate weekly change in the odds of testing positive for influenza 14 days after vaccination.
Results
Of 6490 patients included, 1633 tested positive for influenza. Each week that had elapsed since vaccination was associated with an increase in the odds of testing positive for any influenza (4.9%; 95% CI: 2.0–8.0%) and for A(H3N2) (6.5%; 95% CI: 2.9–10.3%). This decline in VE was, however, significant only in children and older adults. A similar increase in the odds of testing positive was seen when the dataset was restricted to vaccinees only. Conversely, VE waning was less evident for A(H1N1)pdm09 or B strains.
Conclusions
Significant waning of VE, especially against influenza A(H3N2), may be one of the factors associated with suboptimal end-of-season VE. Next-generation vaccines should provide more durable protection against A(H3N2).
1. Introduction
Influenza vaccination is currently the best way to prevent influenza disease and its complications [Citation1], which go far beyond respiratory illness [Citation2]. However, the vaccine effectiveness (VE) of the available inactivated influenza vaccines (IIVs) is imperfect and varies widely from season to season [Citation3]. This suboptimal protection is driven by a number of factors related to the vaccine, vaccinee, and virus [Citation4]. With regard to the virus, IIVs are well known to have comparatively low VE against the A(H3N2) subtype, especially in older adults [Citation5].
The protection induced by IIVs (as well as their regulatory approval) mainly relies on antibodies toward viral hemagglutinin [Citation6,Citation7]. After reaching peak concentrations approximately 2–6 weeks following vaccination [Citation8], antibody titers begin to decline [Citation9]. A recent pooled analysis reported slow antibody waning over the course of six months in children and older adults and against all virus (sub)types. Nevertheless, seroprotection rates were still higher at six months than during the pre-vaccination period, suggesting some level of protection even at the end of a typical influenza season [Citation10]. Antibody decay seems to be driven by a variety of factors, including previous exposures, age of vaccinees and influenza strain. For instance, it has been shown [Citation11] that post-infection titers start higher against A(H3N2) but decay more rapidly than against A(H1N1)pdm09. Younger individuals are typically able to maintain high levels of antibody titers into the following season, while in the elderly vaccine-induced humoral response is lower and wane more rapidly [Citation12]. In any case, the magnitude of antibody titers does not always imply protection and some evidence suggests [Citation13] that fold-change in post-vaccination titers is not associated with IIV VE.
Given that humoral immunogenicity is not a perfect correlate of protection [Citation4], it is crucial, from the policy perspective, to evaluate the intra-season duration of IIV-induced VE. Evidence on the waning of VE is still open to discussion and seems to depend on the surveillance period, the predominant virus strains, methodological approaches and other determinants. For instance, while several studies have found a steady decline in absolute VE [Citation14–17], others [Citation18,Citation19] have reported only modest or non-significant waning over the period of six months. Notably, these studies used two different approaches in evaluating VE waning. Most analyses [Citation14,Citation15,Citation17,Citation20] were based on test-negative case-control (TNCC) studies, in which both vaccinated and unvaccinated persons were included. However, this approach may be subject to the so-called ‘depletion-of-susceptibles’ bias, which arose from differential depletion of susceptible individuals between vaccinated and unvaccinated groups and may lead to the apparent VE waning [Citation21]. To reduce the effects of this bias, the second approach considers only vaccinees and evaluates the risk of influenza at different time points since vaccination [Citation22,Citation23]. This vaccine-only approach may instead underestimate risk of influenza in the early vaccinees and consequently underestimate VE waning [Citation22].
The duration of IIV VE has important public health implications, as it allows decision-makers to establish the optimal timing of the roll-out of immunization campaigns. One modeling study [Citation24] estimated that, while delaying the vaccination campaign could prevent many cases, this approach would inevitably result in more missed opportunities to vaccinate, and thus decreased uptake; overall, delaying vaccination appeared to impact negatively on the total number of cases. Simulation results were highly sensitive to the assumed effect size of VE waning [Citation24], suggesting the need for more research on the issue. In sum, the timing of IIV administration should strike a balance between reducing the probability of VE waning and increasing vaccine uptake in all principal target groups [Citation25]. Uncertainties regarding the effect size of intra-season VE waning, together with a lack of specific Italian data, prompted us to conduct this individual-level pooled study, in which we aimed to assess whether, and to what extent, IIV VE waned in the recent influenza seasons.
2. Methods
2.1. Study design
We performed a pooled analysis of IIV VE studies conducted by the CIRI-IT network (Genoa, Italy) between the 2018/2019 and 2022/2023 northern hemisphere influenza seasons. Specifically, seven TNCC studies were conducted in four geographically representative (Northern, Central and Southern) Italian regions (Liguria, Tuscany, Lazio and Apulia) during the last five influenza seasons. Two of these studies (2018/2019 and 2019/2020) were conducted in primary care settings and enrolled subjects with influenza-like illness (ILI), while the remaining five studies (from 2018/2019 to 2022/2023) were conducted in hospital settings and enrolled individuals with severe acute respiratory infection (SARI) requiring hospitalization. All but one of the seasonal studies were conducted within the DRIVE (Development of Robust and Innovative Vaccine Effectiveness) project [Citation26], while one study was conducted in a large hospital during the 2022/2023 season [Citation27]. SARI surveillance in the 2020/2021 season was seriously affected by the COVID-19 pandemic, and only two influenza virus detections were registered. This study was therefore excluded. In summary, six studies conducted in both outpatient (2018/2019 and 2019/2020) and inpatient (2018/2019, 2019/2020, 2021/2022, and 2022/2023) settings were included the present individual-level pooled analysis. The protocols of all studies had been approved by the pertinent ethics committees. All participants had provided written informed consent.
2.2. Study participants and procedures
Full protocols and results of each seasonal study may be consulted elsewhere [Citation26–29]. Briefly, enrollment of subjects aged ≥6 months took place between mid-October and mid-April and was conducted by general practitioners/pediatricians and in emergency departments for outpatient and inpatient surveillance studies, respectively. Regarding primary care studies, ILI was defined according to the European criteria as follows: the sudden onset of at least one systemic (fever/feverishness, malaise, headache, myalgia) and at least one respiratory (cough, sore throat, shortness of breath) sign or symptom. Concerning hospital-based studies, SARI was defined as the presence of at least one systemic symptom (fever/feverishness, malaise, headache, myalgia), or deterioration of general condition, and at least one respiratory symptom (cough, sore throat, shortness of breath) on admission or within 48 hours following admission. We excluded subjects if: (i) swab delay (i.e. period between symptom onset and swab) was >7 days; (ii) IIV status was unknown; (iii) IIV date was unknown; (iv) IIV was administered <14 days before the onset of symptoms.
On enrollment, relevant demographic and clinical data were collected from all participants (see below) and a naso/oropharyngeal swab was performed. Swabs were then tested in real-time reverse transcription polymerase chain reaction (RT-PCR) for the detection of influenza virus types (A or B) and/or subtypes [A(H1N1)pdm09 and A(H3N2)] and lineages (B/Victoria and B/Yamagata). Subjects testing positive were defined as cases, while those testing negative were defined as controls.
2.3. Study variables
The study outcome (dependent variable) was positivity to any influenza virus, influenza virus type and subtype. Lineage-specific outcomes were not assessed owing to the small number of type B detections. The independent variable of interest was the number of weeks between the time-point of two weeks after IIV administration (henceforth referred to as ‘time since IIV’) and symptom onset [Citation14,Citation15]. Sex (male vs female), age (continuous), presence of co-morbidities (present vs absent), swab delay (discrete from 0 to 7), setting (inpatient vs outpatient) and dummy variables of influenza seasons and study site were taken as potential confounders.
2.4. Statistical analysis
Categorical variables were expressed as proportions with Clopper-Pearson’s 95% confidence intervals (CIs) and compared by means of the chi-square test. Normally distributed variables were expressed as means with standard deviations (SDs) and compared by means of the independent t test. Medians with interquartile ranges (IQRs) were computed for skewed variables and compared by applying the Mann-Whitney test.
To address our research question, we used the two above-mentioned complementary approaches. For the first, we included both vaccinated and unvaccinated individuals, where the latter was the reference category. The time since IIV was coded as the number of weeks between the date of symptom onset and IIV administration for vaccinees, while it was set to zero for unvaccinated individuals [Citation14,Citation15,Citation20]. For the second approach, we included only vaccinated individuals [Citation22]. For both approaches, multivariable logistic regression analysis was performed in order to establish adjusted odds ratios (aORs) on the association between positivity for influenza and time since IIV. The resulting aOR was interpreted as change in the odds of testing positive for influenza for each week that had elapsed since IIV. Analogously, the weekly change in VE may also be expressed (1 – aOR) × 100% and therefore aOR > 1 indicates weekly decline in VE. The base-case models considered subjects of all ages. A subgroup analysis by virus (sub)type and age-group (children aged 6 months to 14 years, working-age adults aged 15–64 years and older adults aged ≥65 years) was also conducted. We then computed the predicted IIV VE over 24 weeks after IIV administration, a period which roughly reflects the entire influenza season, as described by Ferdinands et al. [Citation14,Citation15]. Non-parametric bootstrapped re-sampling of the data with replacement (500 re-samples) was used to estimate the 95% CIs for IIV VE point estimates. An analogous prediction was also made for the probability of influenza in vaccinated individuals only [Citation14,Citation15].
Three kinds of sensitivity analysis were conducted. First, considering that cases and controls were unbalanced in terms of the timing of symptom onset, a matching procedure was conducted to account for the confounding due to calendar time in each region. Cases and controls we matched on the date of symptom onset, season, and study site using the nearest neighbor algorithm; each case was matched to up to three controls [Citation14,Citation15]. Second, as outpatients and inpatients may represent two distinct populations [Citation30], we restricted the dataset to SARI patients only. Finally, as influenza and COVID-19 vaccine behaviors may be interrelated [Citation31], we excluded subjects positive for SARS-CoV-2.
Analyses were carried out by means of R software version 4.1.0 (R Foundation for Statistical Computing; Vienna, Austria).
3. Results
Across four seasons, 6640 subjects were enrolled and tested by means of RT-PCR. A total of 150 (2.3%) subjects were excluded for the following reasons: (i) 12 (0.2%) had swab delay >7 days; (ii) 13 (0.2%) had unknown IIV status; (iii) in 72 (1.1%), the IIV date was unknown; (iv) 53 (0.8%) had been vaccinated <14 days before the onset of symptoms. The final cohort therefore comprised 6490 patients.
A total of 1633 patients tested positive for influenza; the remaining 4857 individuals constituted test-negative controls. In the 2021/2022 and 2022/2023 seasons, 27.6% of subjects tested positive for SARS-CoV-2. Cases differed from controls in terms of all the variables considered, except for sex (). Specifically, most cases were detected during the 2018/2019 and 2019/2020 seasons, with a prevalence of positive samples of 32.2% and 26.5%, respectively. Conversely, the prevalence of positivity was significantly lower in the 2021/2022 (2.6%) and 2022/2023 (12.1%) seasons. Influenza was detected more frequently in outpatients than inpatients. Cases were significantly younger than controls, and consequently showed a lower prevalence of co-morbidities. Indeed, positivity prevalence decreased with age, being 37.1%, 23.5%, and 14.6% in children, working-age, and older adults, respectively. Vaccination coverage was significantly higher in controls (27.6%) than in cases (14.1%) ().
Table 1. Characteristics of the cases (N = 1633) and controls (N = 4857); Italy, 2018/2019 to 2022/2023.
Within cases, influenza type A was more frequently detected in all four seasons. A(H1N1)pdm09 (51.0%) co-circulated with A(H3N2) (43.2%) during the 2018/2019 season. The 2019/2020 season was characterized by the co-circulation of all viruses, with a slightly higher detection of A(H3N2) (38.5%). Conversely, A(H3N2) dominated in two later seasons (2021/2022: 94.7%; 2022/2023: 69.6%). Within influenza B, which was mainly detected in the 2019/2020 season, the Victoria lineage was more frequent than Yamagata (Table S1).
In the all-age model of both vaccinated and unvaccinated individuals (), each one week since IIV was associated with a 4.9% (p = 0.001) increase in the odds of testing positive for any influenza and a 6.5% (p < 0.001) increase in the odds of testing positive for A(H3N2). This association was not significant in the case of A(H1N1)pdm09 (aOR = 1.003; p = 0.91) or B (aOR = 1.073; p = 0.24) strains. As shown in , the projected VE against A(H3N2) was 47.1% (95% CI: 21.8–65.9%) two weeks after IIV, and reached 0% (95% CI: −54.7–48.4%) approximately 14 weeks post-vaccination (14 weeks: 46.1%; 95% CI: −11.4–78.8%). Conversely, VE against A(H1N1)pdm09 was constantly ≥ 45%. VE against influenza B was 75% (95% CI: 2.1–93.6%) two weeks post-vaccination, decreased more slowly and approached 0% (95% CI: −20.2–96.3%) between weeks 22 and 23, and thus at the very end of the influenza season. However, owing to a relatively small number of detections, the results concerning the B virus type should be interpreted cautiously. No significant two-way interaction emerged between time since IIV and age.
Figure 1. Projected weekly decline in influenza vaccine effectiveness in the total cohort (N = 6490) and probability of influenza among vaccinated individuals only (N = 1571), by virus (sub)type; Italy, 2018/2019 to 2022/2023.
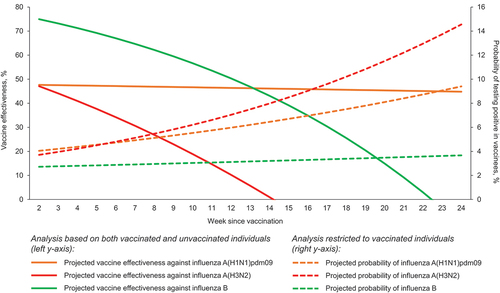
Table 2. Association between the time since vaccination and positivity to influenza virus, by virus (sub)type; Italy, 2018/2019 to 2022/2023.
In a second analysis, in which only vaccinated subjects (N = 1571) were included, each one week since vaccination was associated (p < 0.001) with a 6% and 7% increase in the odds of testing positive for any influenza and A(H3N2), respectively. For instance, while the probability of A(H3N2) detection in the vaccinated cohort was 3.7% two weeks post-vaccination, it rose to 14.6% at week 24. With regard to both A(H1N1)pdm09 (aOR = 1.042; p = 0.13) and B (aOR = 1.083; p = 0.23), the increase was not significant. Notably, although not statistically significant, the risk of A(H1N1)pdm09 in vaccinated individuals increased over time ().
When subjects were stratified by age (), significant waning of VE against any influenza and A(H3N2) was observed only in children and older adults. Conversely, the association between time since IIV and positivity for any influenza virus was not significant in working-age adults. Age-specific results were similar for the analysis based on all subjects and for that restricted to vaccinated individuals only.
Table 3. Association between the time since vaccination and positivity to influenza virus, by age-group and virus (sub)type; Italy, 2018/2019 to 2022/2023.
In the first sensitivity analysis to account for the confounding due to calendar time (), the estimated increase in the odds of any influenza for each one week since IIV raised up to 6.3% (p < 0.001) and 7.6% (p = 0.001) in the entire and vaccine-only cohorts, respectively. For the A(H3N2) subtype, VE waning was significant in the vaccine-only subset (aOR = 1.076; p = 0.022) and not in the total cohort (aOR = 1.046; p = 0.085). The odds of influenza A(H1N1)pdm09 barely (p = 0.050) increased (8.6%) with each week post-vaccination. No significant changes were found for influenza B.
Table 4. Association between the time since vaccination and positivity to influenza virus: a sensitivity analysis based on the matching procedure to reduce the confounding due to calendar time.
When only SARI cases (N = 4495) were considered (Table S2), results were similar to the base case. However, in the cohort of vaccinees only, the odds of testing positive for A(H1N1)pdm09 turned significant (p = 0.021) with an estimated weekly increase of 8.5%. Lastly, when SARS-CoV-2 positive subjects (N = 333) were excluded (Table S3), results were very close to the base case.
4. Discussion
This large pooled analysis showed that the comparatively low end-of-season VE against A(H3N2) [Citation5] is also driven by rapid waning of VE against this subtype, especially in children and older adults. While at the beginning of the influenza season VE against A(H3N2) was similar to VE against A(H1N1)pdm09, the former decreased rapidly. In any case, it should be stressed that the available vaccines were still able to provide protection against A(H3N2) for approximately the first three months. Our second major finding is that both approaches used to estimate VE waning (i.e. with or without inclusion of unvaccinated subjects) produced roughly comparable results. Similar findings emerged in a US study [Citation14] that covered a period from 2011/2012 to 2014/2015. As noted by those authors, there is no well-established method for modeling the association between VE and time since IIV [Citation14]. We therefore believe that both approaches, which have their own strengths and weaknesses, may be scrutinized for eventual differences.
In a systematic review and meta-analysis of 14 TNCC studies, Young et al. [Citation17] compared VE measured 15–90 and 91–180 days after vaccination. They found a significant decline in VE against A(H3N2) (45% vs 13%) and B (62% vs 43%) strains, while the difference was not significant for A(H1N1)pdm09 (62% vs 54%). Compared with previous research, we noted that our findings are most coherent with those reported by other studies conducted in Europe. Thus, our results are in full agreement with a pooled analysis of the I-MOVE TNCC studies conducted between 2011/2012 and 2014/2015 seasons [Citation20]. In that study, modeled VE against influenza A(H3N2) declined from 50.6% 38 days after IIV to 0% from 111 days (15.8 weeks) onwards. VE against influenza B declined more slowly, being 70.7% 44 days post-vaccination and 21.4% at the end of the influenza season. Conversely, VE against A(H1N1)pdm09 was relatively stable, varying by only 5% (from 55.3% to 50.3%). Analogously, a Spanish study [Citation32] documented a lower risk of influenza among late vaccinees during A(H3N2)-dominant seasons and non-significant differences between late and early vaccinees during the seasons dominated by A(H1N1)pdm09 or B/Yamagata. On the other hand, studies conducted in the US [Citation14,Citation15] showed a steady decline in IIV VE for all vaccine strains. For instance, a study conducted by Ferdinands et al. [Citation15] on adult (≥18 years) inpatients estimated a monthly decline in VE of 7.5%, 8.5% and 8.0% against A(H3N2), A(H1N1)pdm09 and B/Yamagata, respectively. We believe that some of the differences between the US and European studies may be explained by the earlier start of the vaccination campaign in the US. For example, in Italy, the first vaccine doses are typically administered in mid-October, and most vaccinations are performed in November, while in the US vaccination should be offered soon after vaccines becomes available and the first doses may be administered as early as August [Citation14,Citation33].
Several concurrent explanations for the relatively low end-of-season VE against A(H3N2) have been hypothesized, including waning immunity, rapid within-season A(H3N2) antigenic drift, increasing glycosylation of hemagglutinin, the co-circulation of different virus subclades and the complex effects of repeated vaccination [Citation17,Citation34,Citation35]. Waning antibody concentrations is likely a key contributor. Hoa et al. [Citation11] demonstrated a comparatively rapid decline of seroprotection rates following natural A(H3N2) infection. Among vaccinated individuals, Song et al. [Citation36] showed a significant decrease in seroprotection rates toward A(H3N2) six months post-vaccination in all subgroup of adults, especially in the elderly. Cobey et al. [Citation37] claimed that poor vaccine immunogenicity and not egg-adaptive mutations in the A(H3N2) strain were responsible for the low VE observed in a season. Second, the effect of repeated vaccination is to be considered. The latest systematic review and meta-analysis [Citation38] reported a decrease of 18% in VE for A(H3N2) for vaccination in both seasons compared with the current season only. In comparison, the estimates for A(H1N1)pdm09 and B were −9% and −7%, respectively. Thompson et al. [Citation39] documented an inverse relationship between post-vaccination antibody titers and the number of prior vaccinations. Notably, at the end of season, subjects with one prior IIV dose maintained higher antibody titers against A(H3N2) and had higher seroprotection rates than individuals with four prior vaccinations. Underlying immunological mechanisms through which prior vaccinations can affect VE of subsequent vaccinations remain unclear [Citation40]. According to the antigenic distance hypothesis, the effect of prior IIV is determined by the antigenic distance between the vaccine strains in the prior dose versus the subsequent doses and the antigenic distance between the vaccine strain and the circulating virus. The blunting of IIV immunogenicity and VE by prior vaccination would be the most pronounced when the vaccine strain was unchanged, but an antigenically distinct virus became the predominant strain [Citation40]. Finally, although a relatively high mutation rate of A(H3N2) strains may lead to co-circulation of antigenically different virus populations, the available evidence suggests [Citation41] that within-season and within-host antigenic drift of the A(H3N2) subtype is unlikely a major determinant of IIV failures.
We observed that IIV VE waned significantly only in children and older adults, and not in working-age adults. Analogous results have been reported by Belongia et al. [Citation33], who noted a significant interaction between age and vaccination timing. The observed decline was particularly pronounced at the extremes of the age-range: children aged two years and adults aged 75 years showed aORs of 1.20 and 1.31, respectively, for influenza A positivity for each two-week increase in time since vaccination [Citation33]. These authors suggested that the underlying mechanisms probably differ between children and older adults. Indeed, young children have an immature immune system, and most of them have not been primed by natural infection, while older adults are subject to immunosenescence phenomena [Citation33]. This finding closely reflects the available evidence from immunogenicity studies. In a meta-analysis of ≥ 74 trials [Citation42], the odds of (sub)type-specific seroprotection following IIV were 60–90% lower in both children and in older adults than in working-age adults.
For what concerns influenza A(H1N1)pdm09 and B, we did not find a compelling evidence of IIV VE decline. Similarly to the meta-analysis by Young et al. [Citation17], the estimated VE decrease for A(H1N1)pdm09 was not significant in both base-case models. However, when the analysis was restricted to SARI cases, the effect size increased significantly, being similar to that of A(H3N2). This may be ascribable to the fact that individuals infected with A(H1N1)pdm09 experience the most severe outcomes [Citation43]. For influenza B, the projected VE waning was evident (), but the point estimates were highly imprecise and did not reach significance. It is therefore likely that our analysis for influenza B was underpowered.
The main study strengths of the present study lie in the inclusion of multiple seasons, consistent protocols and a relatively large number of positive samples collected from representative Italian locations. However, some important limitations must be acknowledged. First, although a recent analysis suggested a parsimonious approach to confounder adjustment in TNCC studies [Citation44], our estimates may have been affected by residual confounding. For instance, we were unable to adjust for IIV administration during the previous season (as we discussed above, it may interfere with the decline in VE), as information on this variable was missing in a large number of participants. Second, while pluriannual data are of interest for policy makers, a limited number of cases in the post-COVID-19 period did not allow us to estimate season-specific VE waning, which likely varies from season to season. Third, as we also noted earlier, the estimates related to influenza B may be underpowered owing to a smaller number of B strain detections, especially among adult and vaccinated individuals. Analogously, not all participating laboratories were able to determine the lineage of type B virus detections, which prevented us from estimating lineage-specific VE decline. Finally, despite the fact that we tried different approaches that produced similar output, our results may be still affected by the depletion-of-susceptibles bias.
5. Conclusion
In conclusion, we observed a significant decline in IIV VE, which was primarily driven by the A(H3N2) subtype. The question of whether the vaccination campaign should be delayed is highly controversial. While a modest delay could potentially prevent some additional cases [Citation24,Citation25], this benefit may be completely offset by the missed opportunities for vaccination [Citation24]. The issue is further complicated by the unpredictable epidemiology of influenza. For instance, in the 2016/2017 [Citation45] and 2022/2023 [Citation46] seasons in Europe, both of which were dominated by A(H3N2), the epidemic started early, reaching its peak as early as in December. In terms of increasing the currently insufficient vaccine uptake, postponing the immunization campaign, even for a few weeks, does not seem to be advisable. It is clear, instead, that vaccination should be recommended and actively offered throughout the entire influenza season and every time that eligible subjects come into contact with healthcare services, in order to minimize missed opportunities for vaccination [Citation47].
Another way to increase the durability of protection could be a two-dose regimen, which is currently recommended only for unprimed children <9 years [Citation48]. A recent US modeling study [Citation49] indicated that two IIV doses may substantially decrease cases and hospitalizations in older adults. On the other hand, the experimental evidence on the utility of a booster dose is controversial. For instance, in one trial [Citation50] compared with the single-dose regimen, seroprotection rates at 10 weeks were higher in solid organ transplant recipients administered two IIV doses. Other studies on immunocompromised subjects [Citation51,Citation52] showed no or little advantage of the second dose. Conversely, it is well known that the so-called ‘enhanced’ IIVs, such as high-dose or adjuvanted IIVs, are more immunogenic and effective than standard-dose non-adjuvanted IIVs [Citation53] and have been recently granted a preferential recommendation for older adults [Citation48,Citation54]. Additionally, Noh et al. [Citation55] reported that in adults with end-stage renal disease antibody titers at six months post-vaccination tended to be higher among recipients of the adjuvanted than non-adjuvanted standard-dose IIVs. We therefore believe that the second off-label dose may be considered only in some particular cases, while the adoption of the enhanced IIV formulations should be increased. Hopefully, next-generation influenza vaccines, some of which are in the late stage of clinical development [Citation56,Citation57], will address the unmet need to improve VE against A(H3N2). Finally, we suggest that the duration of protection should be evaluated in clinical trials of these vaccine candidates.
Declaration of interests
A Domnich provided consultation and received speaker fees from CSL Seqirus, GSK and SD Biosensor. A Orsi provided consultation and/or received speaker fees from CSL Seqirus, Moderna, Novavax and SD Biosensor. D Panatto provided consultation for Pfizer and CSL Seqirus and received grants to conduct observational studies from Sanofi, Pfizer, GSK and Viatris. C Rizzo provided consultation and received speaker fees from CSL Seqirus, GSK, MSD, AstraZeneca and Sanofi. G Icardi provided consultation and/or received grants to conduct experimental and/or observational studies for GSK, Sanofi, MSD, CSL Seqirus and Pfizer. The authors have no other relevant affiliations or financial involvement with any organization or entity with a financial interest in or financial conflict with the subject matter or materials discussed in the manuscript apart from those disclosed.
Reviewer disclosures
Peer reviewers on this manuscript have no relevant financial or other relationships to disclose.
Author contributions
All named authors meet the International Committee of Medical Journal Editors (ICMJE) criteria for authorship for this article. All authors contributed to study conception and design, data acquisition, analysis, and interpretation, drafting and revising of the manuscript.
Ethical approval and patient consent
All studies have been approved by the pertinent ethics committees. All participants have provided written informed consent.
Supplemental Material
Download MS Word (13.7 KB)Supplemental Material
Download MS Word (13.6 KB)Supplemental Material
Download MS Word (13.6 KB)Supplementary material
Supplemental data for this article can be accessed online at https://doi.org/10.1080/14760584.2024.2331073.
Additional information
Funding
References
- World Health Organization (WHO). Vaccines against influenza: WHO position paper – May 2022. Wkly Epidemiol Rec. 2022;97(19):185–208.
- Sellers SA, Hagan RS, Hayden FG, et al. The hidden burden of influenza: a review of the extra-pulmonary complications of influenza infection. Influenza Other Respir Viruses. 2017;11(5):372–393. doi: 10.1111/irv.12470
- Centers for Disease Control and Prevention (CDC). Past seasons’ vaccine effectiveness estimates. [cited 2023 Nov 15]. Available from: https://www.cdc.gov/flu/vaccines-work/past-seasons-estimates.html
- Domnich A, Manini I, Calabrò GE, et al. Mapping host-related correlates of influenza vaccine-induced immune response: an umbrella review of the available systematic reviews and meta-analyses. Vaccines (Basel). 2019;7(4):215. doi: 10.3390/vaccines7040215
- Belongia EA, Simpson MD, King JP, et al. Variable influenza vaccine effectiveness by subtype: a systematic review and meta-analysis of test-negative design studies. Lancet Infect Dis. 2016;16(8):942–951. doi: 10.1016/s1473-3099(16)00129-8
- Domnich A, Manini I, Panatto D, et al. Immunogenicity measures of influenza vaccines: a study of 1164 registered clinical trials. Vaccines (Basel). 2020;8(2):325. doi: 10.3390/vaccines8020325
- Wen S, Wu Z, Zhong S, et al. Factors influencing the immunogenicity of influenza vaccines. Hum Vaccin Immunother. 2021;17(8):2706–2718. doi: 10.1080/21645515.2021.1875761
- Rastogi S, Gross PA, Bonelli J, et al. Time to peak serum antibody response to influenza vaccine. Clin Diagn Lab Immunol. 1995;2(1):120–121. doi: 10.1128/cdli.2.1.120-121.1995
- Kositanont U, Assantachai P, Wasi C, et al. Kinetics of the antibody response to seasonal influenza vaccination among the elderly. Viral Immunol. 2012;25(6):471–476. doi: 10.1089/vim.2012.0024
- Doyon-Plourde P, Przepiorkowski J, Young K, et al. Intraseasonal waning immunity of seasonal influenza vaccine – a systematic review and meta-analysis. Vaccine. 2023;41(31):4462–4471.
- Hoa LNM, Sullivan SG, Mai LQ, et al. Influenza A(H1N1)pdm09 but not A(H3N2) virus infection induces durable seroprotection: results from the ha nam cohort. J Infect Dis. 2022;226(1):59–69. doi: 10.1093/infdis/jiaa293
- Carlock MA, Ingram JG, Clutter EF, et al. Impact of age and pre-existing immunity on the induction of human antibody responses against influenza B viruses. Hum Vaccin Immunother. 2019;15(9):2030–2043. doi: 10.1080/21645515.2019.1642056
- Gilbert PB, Fong Y, Juraska M, et al. HAI and NAI titer correlates of inactivated and live attenuated influenza vaccine efficacy. BMC Infect Dis. 2019;19(1):453. doi: 10.1186/s12879-019-4049-5
- Ferdinands JM, Fry AM, Reynolds S, et al. Intraseason waning of influenza vaccine protection: evidence from the US influenza vaccine effectiveness network, 2011–12 through 2014–15. Clin Infect Dis. 2017;64(5):544–550.
- Ferdinands JM, Gaglani M, Martin ET, et al. Waning vaccine effectiveness against influenza-associated hospitalizations among adults, 2015–2016 to 2018–2019, United States Hospitalized Adult Influenza Vaccine Effectiveness Network. Clin Infect Dis. 2021;73(4):726–729.
- Hu W, Sjoberg PA, Fries AC, et al. Waning vaccine protection against influenza among department of defense adult beneficiaries in the United States, 2016–2017 through 2019–2020 influenza seasons. Vaccines (Basel). 2022;10(6):888.
- Young B, Sadarangani S, Jiang L, et al. Duration of influenza vaccine effectiveness: a systematic review, meta-analysis, and meta-regression of test-negative design case-control studies. J Infect Dis. 2018;217(5):731–741. doi: 10.1093/infdis/jix632
- Radin JM, Hawksworth AW, Myers CA, et al. Influenza vaccine effectiveness: maintained protection throughout the duration of influenza seasons 2010-2011 through 2013–2014. Vaccine. 2016;34(33):3907–3912.
- Petrie JG, Ohmit SE, Truscon R, et al. Modest waning of influenza vaccine efficacy and antibody titers during the 2007–2008 influenza season. J Infect Dis. 2016;214(8):1142–1149.
- Kissling E, Nunes B, Robertson C, et al. I-MOVE multicentre case-control study 2010/11 to 2014/15: is there within-season waning of influenza type/subtype vaccine effectiveness with increasing time since vaccination? Euro Surveill. 2016;21(16). doi: 10.2807/1560-7917.es.2016.21.16.30201
- Lipsitch M, Goldstein E, Ray GT, et al. Depletion-of-susceptibles bias in influenza vaccine waning studies: how to ensure robust results. Epidemiol Infect. 2019;147:e306. doi: 10.1017/S0950268819001961
- Ray GT, Lewis N, Klein NP, et al. Intraseason waning of influenza vaccine effectiveness. Clin Infect Dis. 2019;68(10):1623–1630. doi: 10.1093/cid/ciy770
- Chung H, Campitelli MA, Buchan SA, et al. Measuring waning protection from seasonal influenza vaccination during nine influenza seasons, Ontario, Canada, 2010/11 to 2018/19. Euro Surveill. 2024;29(8). doi: 10.2807/1560-7917.ES.2024.29.8.2300239.
- Costantino V, Trent M, MacIntyre CR. Modelling of optimal timing for influenza vaccination as a function of intraseasonal waning of immunity and vaccine coverage. Vaccine. 2019;37(44):6768–6775. doi: 10.1016/j.vaccine.2019.08.069.
- Newall AT, Chen C, Wood JG, et al. Within-season influenza vaccine waning suggests potential net benefits to delayed vaccination in older adults in the United States. Vaccine. 2018;36(39):5910–5915. doi: 10.1016/j.vaccine.2018.08.007.
- DRIVE consortium. Core protocol for type/brand specific influenza vaccine effectiveness studies (test-negative design studies). [cited 2023 Nov 15]. Available from: https://www.drive-eu.org/index.php/results/deliverables/
- Domnich A, Orsi A, Ogliastro M, et al. Influenza vaccine effectiveness in preventing hospital encounters for laboratory-confirmed infection among Italian adults, 2022/23 season. Vaccine. 2023;41(33):4861–4866. doi: 10.1016/j.vaccine.2023.06.072
- Rizzo C, Gesualdo F, Loconsole D, et al. Moderate vaccine effectiveness against severe acute respiratory infection caused by A(H1N1)pdm09 influenza virus and no effectiveness against A(H3N2) influenza virus in the 2018/2019 season in Italy. Vaccines (Basel). 2020;8(3):427.
- Panatto D, Domnich A, Chironna M, et al. Surveillance of severe acute respiratory infection and influenza vaccine effectiveness among hospitalized Italian adults, 2021/22 season. Vaccines (Basel). 2022;11(1):83. doi: 10.3390/vaccines11010083
- Tenforde MW, Chung J, Smith ER, et al. Influenza vaccine effectiveness in inpatient and outpatient settings in the United States, 2015–2018. Clin Infect Dis. 2021;73(3):386–392.
- Doll MK, Pettigrew SM, Ma J, et al. Effects of confounding bias in coronavirus disease 2019 (COVID-19) and influenza vaccine effectiveness test-negative designs due to correlated influenza and COVID-19 vaccination behaviors. Clin Infect Dis. 2022;75(1):e564–e571. doi: 10.1093/cid/ciac234
- Puig-Barberà J, Mira-Iglesias A, Tortajada-Girbés M, et al. Waning protection of influenza vaccination during four influenza seasons, 2011/2012 to 2014/2015. Vaccine. 2017;35(43):5799–5807. doi: 10.1016/j.vaccine.2017.09.035
- Belongia EA, Sundaram ME, McClure DL, et al. Waning vaccine protection against influenza a (H3N2) illness in children and older adults during a single season. Vaccine. 2015;33(1):246–251. doi: 10.1016/j.vaccine.2014.06.052
- Belongia EA, McLean HQ. Influenza vaccine effectiveness: Defining the H3N2 problem. Clin Infect Dis. 2019;69(10):1817–1823. doi: 10.1093/cid/ciz411
- Pando R, Stern S, Nemet I, et al. Diversity in the circulation of influenza A(H3N2) viruses in the Northern Hemisphere in the 2018-19 season. Vaccines (Basel). 2021;9(4):375.
- Song JY, Cheong HJ, Hwang IS, et al. Long-term immunogenicity of influenza vaccine among the elderly: risk factors for poor immune response and persistence. Vaccine. 2010;28(23):3929–3935. doi: 10.1016/j.vaccine.2010.03.067
- Cobey S, Gouma S, Parkhouse K, et al. Poor immunogenicity, not vaccine strain egg adaptation, may explain the low H3N2 influenza vaccine effectiveness in 2012–2013. Clin Infect Dis. 2018;67(3):327–333.
- Jones-Gray E, Robinson EJ, Kucharski AJ, et al. Does repeated influenza vaccination attenuate effectiveness? A systematic review and meta-analysis. Lancet Respir Med. 2023;11(1):27–44. doi: 10.1016/S2213-2600(22)00266-1
- Thompson MG, Naleway A, Fry AM, et al. Effects of repeated annual inactivated influenza vaccination among healthcare personnel on serum hemagglutinin inhibition antibody response to A/Perth/16/2009 (H3N2)-like virus during 2010–11. Vaccine. 2016;34(7):981–988. doi: 10.1016/j.vaccine.2015.10.119
- Thompson MG, Cowling BJ. How repeated influenza vaccination effects might apply to COVID-19 vaccines. Lancet Respir Med. 2022;10(7):636–638. doi: 10.1016/S2213-2600(22)00162-X
- Debbink K, McCrone JT, Petrie JG, et al. Vaccination has minimal impact on the intrahost diversity of H3N2 influenza viruses. PLoS Pathog. 2017;13(1):e1006194. doi: 10.1371/journal.ppat.1006194
- Seidman JC, Richard SA, Viboud C, et al. Quantitative review of antibody response to inactivated seasonal influenza vaccines. Influenza Other Respir Viruses. 2012;6(1):52–62. doi: 10.1111/j.1750-2659.2011.00268.x
- Sumner KM, Masalovich S, O’Halloran A, et al. Severity of influenza-associated hospitalisations by influenza virus type and subtype in the USA, 2010–19: a repeated cross-sectional study. Lancet Microbe. 2023;4(11):e903–e912.
- Stuurman AL, Levi M, Beutels P, et al. Investigating confounding in network-based test-negative design influenza vaccine effectiveness studies-experience from the DRIVE project. Influenza Other Respir Viruses. 2023;17(1):e13087.
- European Centres for Disease Prevention and Control (ECDC). Summary of the influenza 2016–2017 season in Europe. [cited 2023 Nov 15]. Available from: https://www.ecdc.europa.eu/en/publications-data/summary-influenza-2016-2017-season-europe#:~:text=The%20season%20lasted%20for%2027,%2F2016%20and%205%2F2017
- European Centres for Disease Prevention and Control (ECDC). Seasonal influenza 2022−2023. Annual epidemiological report for 2023 [cited 2023 Nov 15]. Available from: https://www.ecdc.europa.eu/sites/default/files/documents/seasonal-influenza-annual-epidemiological-report-2022-2023.pdf
- Domnich A, Orsi A, Ogliastro M, et al. Exploring missed opportunities for influenza vaccination and influenza vaccine co-administration patterns among Italian older adults: a retrospective cohort study. Eur J Public Health. 2023;33(6):1183–1187. doi: 10.1093/eurpub/ckad155
- Italian Ministry of Health. Prevention and control of influenza: recommendations for the 2023-24 season. [cited 2023 Nov 15]. Available from: https://www.trovanorme.salute.gov.it/norme/renderNormsanPdf?anno=2023&codLeg=93294&parte=1%20&serie=null
- Williams KV, Krauland MG, Harrison LH, et al. Can a two-dose influenza vaccine regimen better protect older adults? An agent-based modeling study. Vaccines (Basel). 2022;10(11):1799. doi: 10.3390/vaccines10111799
- Cordero E, Roca-Oporto C, Bulnes-Ramos A, et al. Two doses of inactivated influenza vaccine improve immune response in solid organ transplant recipients: Results of TRANSGRIPE 1–2, a randomized controlled clinical trial. Clin Infect Dis. 2017;64(7):829–838.
- Ljungman P, Nahi H, Linde A. Vaccination of patients with haematological malignancies with one or two doses of influenza vaccine: a randomised study. Br J Haematol. 2005;130(1):96–98. doi: 10.1111/j.1365-2141.2005.05582.x
- Nunes MC, Cutland CL, Moultrie A, et al. Immunogenicity and safety of different dosing schedules of trivalent inactivated influenza vaccine in pregnant women with HIV: a randomised controlled trial. Lancet HIV. 2020;7(2):e91–e103. doi: 10.1016/S2352-3018(19)30322-4
- Domnich A, de Waure C. Comparative effectiveness of adjuvanted versus high-dose seasonal influenza vaccines for older adults: a systematic review and meta-analysis. Int J Infect Dis. 2022;122:855–863. doi: 10.1016/j.ijid.2022.07.048
- Grohskopf LA, Blanton LH, Ferdinands JM, et al. Prevention and control of seasonal influenza with vaccines: recommendations of the Advisory Committee on Immunization Practices—United States, 2023–24 influenza season. MMWR Recomm Rep. 2023;72(2):1–25. doi: 10.15585/mmwr.rr7202a1
- Noh JY, Song JY, Choi WS, et al. Immunogenicity of trivalent influenza vaccines in patients with chronic kidney disease undergoing hemodialysis: MF59-adjuvanted versus non-adjuvanted vaccines. Hum Vaccin Immunother. 2016;12(11):2902–2908. doi: 10.1080/21645515.2016.1191717
- Shartouny JR, Lowen AC. Message in a bottle: mRNA vaccination for influenza. J Gen Virol. 2022;103(7):001765. doi: 10.1099/jgv.0.001765
- Shinde V, Cho I, Plested JS, et al. Comparison of the safety and immunogenicity of a novel matrix-M-adjuvanted nanoparticle influenza vaccine with a quadrivalent seasonal influenza vaccine in older adults: a phase 3 randomised controlled trial. Lancet Infect Dis. 2022;22(1):73–84. doi: 10.1016/s1473-3099(21)00192-4