ABSTRACT
Introduction
Zebrafishes represent a proven model for human diseases and systems biology, exhibiting physiological and genetic similarities and having innate and adaptive immune systems. However, they are underexplored for human vaccinology, vaccine development, and testing. Here we summarize gaps and challenges.
Areas covered
Zebrafish models have four potential applications: 1) Vaccine safety: The past successes in using zebrafishes to test xenobiotics could extend to vaccine and adjuvant formulations for general safety or target organs due to the zebrafish embryos’ optical transparency. 2) Innate immunity: The zebrafish offers refined ways to examine vaccine effects through signaling via Toll-like or NOD-like receptors in zebrafish myeloid cells. 3) Adaptive immunity: Zebrafishes produce IgM, IgD,and two IgZ immunoglobulins, but these are understudied, due to a lack of immunological reagents for challenge studies. 4) Systems vaccinology: Due to the availability of a well-referenced zebrafish genome, transcriptome, proteome, and epigenome, this model offers potential here.
Expert Opinion
It remains unproven whether zebrafishes can be employed for testing and developing human vaccines. We are still at the hypothesis-generating stage, although it is possible to begin outlining experiments for this purpose. Through transgenic manipulation, zebrafish models could offer new paths for shaping animal models and systems vaccinology.
1. Introduction
Our two most recent and gravest pandemic threats – Ebola virus infection, which emerged in The Democratic Republic of the Congo in 2019, and COVID-19 from Central China – required global and coordinated efforts to rapidly accelerate, test, and distribute new vaccines. The lead vaccines that were successfully developed relied on a portfolio approach using multiple platforms [Citation1]. They included recombinant vesicular stomatitis virus (VSV) and adenovirus constructs, inactivated viruses, attenuated viruses, recombinant proteins produced either in mammalian cells or through microbial fermentation, virus-like particles (VLPs), DNAs delivered through electroporation, and mRNAs in lipid-nanoparticles [Citation1–4]. The lead target antigens and their adjuvant immunostimulants were simultaneously advanced into prototype vaccines through such platforms. These prototype vaccines were then accelerated through preclinical animal testing before the safest and most efficacious vaccines were selected for advanced product and clinical development.
This approach, in which identified vaccine targets are simultaneously advanced in multiple platforms for vaccine preclinical testing and evaluation, works; we now have proof-of-concept for its success in addressing pandemic threats. The vaccines for Ebola virus infection and COVID-19 saved millions of lives [Citation5]. However, there is always room to improve preparedness and efficiencies, reduce costs, and strive for faster development timelines. The international Coalition for Epidemic Preparedness Innovation (CEPI) aspires to whittle the sequence of vaccine discovery, development, and delivery in response to a pandemic threat down to 100 days [Citation6]. Among the bottlenecks to achieving this objective is the need for laboratory animal safety and immunogenicity studies. A second hurdle in shortening vaccine timelines is a better understanding of host immunogenetics and how to apply this information toward systems vaccinology [Citation7–10]. Here, we evaluate whether the zebrafish offers advantages for accelerating vaccine science or what additional information would be required to advance zebrafish models as an alternative for assessing vaccines to counter pandemic threats. This aspect also includes the potential for zebrafish models to address the aspirational principles of the ‘3Rs’ (replacement, reduction, and refinement) as advocated by the US National Institutes of Health and other national or international research organizations and committees [Citation11,Citation12]. As a case study, we also evaluate the potential for the zebrafish in the development of NextGen universal or mucosal coronavirus vaccines.
2. Overview and relevant background
The prospect of the zebrafish (Danio rerio) becoming a rapid or high-throughput laboratory animal model for testing new vaccine technologies or adjuvant formulations remains unknown. These teleosts or bony fish are underexplored for this purpose but offer some theoretical advantages. Although zebrafishes are more distant phylogenetically to humans than mice or non-human primates, they still exhibit multiple physiological and pharmacological similarities and a similar genome, with approximately 70% of human genes having a zebrafish equivalent [Citation13,Citation14]. In some cases, the zebrafish can replicate human illness more precisely than mouse or rodent models, a well-known example is the effects of thalidomide to replicate human birth defects in zebrafishes but not in mice [Citation15]. Studies conducted over the last four decades have shown how the zebrafish exhibits high levels of fertility and with the capacity for generating large numbers of embryos (including ones with targeted mutations) suitable for cost-effective genetic and pharmacological screens, as well as live imaging technologies [Citation16–18]. As a practical matter, zebrafishes can also be housed in densities (up to 50 adult fish per tank) higher than mice (4–6 mice per cage) or other common laboratory mammals [Citation18].
As zebrafish research has expanded there is increasing interest in this innovative alternative for animal experimentation, especially in the areas of infection and immunity [Citation19]. Briefly, teleosts such as the zebrafish exhibit both innate and adaptive immunity. Regarding the former, the zebrafish produces essential immunocompetent cell types, including natural killer cells, macrophages, neutrophils, eosinophils, and mast cells, in addition to the major cytokine families such as IFN-γ, IL-1β, IL-6, and TNF-α, which are also found in mammals. They also produce T and B cells required for adaptive immune responses and have T cell receptors, immunoglobulins, and genes required for V(D)J recombination. However, the zebrafish produces fewer antibody-producing B cells, by several orders of magnitude according to some estimates, and therefore a far smaller antibody repertoire [Citation20]. The capacity for the zebrafish to mount host immunity varies with their life history stages. As both embryos and larvae, the zebrafish can mobilize innate immune responses, but adaptive immunity with specific immunoglobulin gene rearrangement and antibody production does not manifest until around one month [Citation19,Citation20]. Zebrafishes sexually mature by three months, are useful as a laboratory test system for up to two years, and can live for five years [Citation21].
3. Evaluating vaccine safety
Zebrafishes have not been studied extensively to evaluate vaccine safety, but there are elements of zebrafish development to indicate that they offer some potential. This is especially true for zebrafish embryos, which develop through a series of discrete and observable stages, aided by the fact that they are transparent () [Citation21].
Figure 1. Reprinted from “zebrafish developmental timeline,” by BioRender.com (2024). Retrieved from https://app.biorender.com/biorender-templates. From Stephanie Lepage (Creator) and Ann Sanderson.
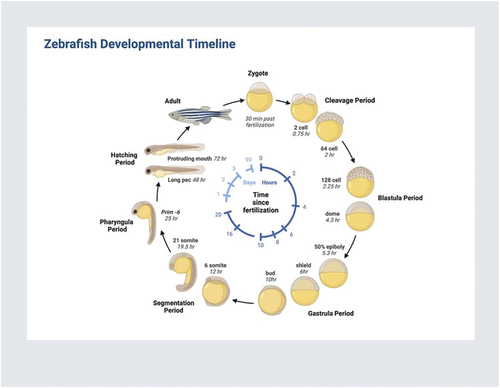
Optical transparency allows for straightforward microinjection of vaccine antigens, adjuvants, or other test articles, followed by observation through cell and organ live imaging [Citation20]. Organ development following fertilization and embryogenesis takes place for approximately 36 hours. During this period the effects of vaccines or their constituent antigens and adjuvants can be monitored, either by conventional histopathology or through fluorescent-tagged biomarkers attached to macromolecules or cell types [Citation19]. Immunocompetent cells linked to innate immunity, such as neutrophils, can also be labeled and followed [Citation20]. Because of the availability of a detailed and well-annotated reference genome [Citation14], as well as detailed zebrafish proteomes, metabolomes, transcriptomes, and other OMICs, changes following immunization or injection can also be assessed [Citation22–32]. These aspects could allow for the potential assessment or screening of the biological effects of a new vaccine, with the understanding that it might require the pooling of dozens or even hundreds of embryos together as opposed to assessing inter-embryo variability. However, a protocol could be potentially shaped that provides ‘one-stop shopping’ for analyzing the end-organ effects of immunization (or series of immunizations) on multiple systems at the whole organ, cellular, subcellular, and biochemical levels.
The zebrafish can also be used to evaluate general animal and organ safety and are potentially suitable for toxicology analyses that are typically required by global regulatory authorities [Citation33,Citation34]. Such studies could incorporate potential hepatotoxicity, nephrotoxicity, neurotoxicity, and cardiac effects [Citation34]. For this purpose, it is useful to keep in mind the different ages or stages of the zebrafish to be employed, each with potential advantages or disadvantages. Therefore, the adult zebrafish could help evaluate general toxicity but would be lower in throughput or might not adequately model pediatric effects. In contrast, zebrafish embryos or larvae exhibit high throughput potential but may not be representative of the toxicities in adults. The Organization for Economic Co-operation and Development (OECD), which includes the United States (US), has released guidelines for employing the zebrafish to evaluate acute toxicity [Citation35], although currently, the US Food and Drug Administration (FDA) does not list the zebrafish as a substitute for rodent or other ‘pharmacologically-relevant species’ for good laboratory practices (GLP) toxicology testing [Citation36,Citation37].
Another promising avenue is the study of vaccines or immunizations for inducing host epigenetic changes [Citation38,Citation39]. Increasingly, zebrafishes are employed to model epigenetic alterations in a variety of organ systems, especially following modifications through xenobiotic exposure [Citation40–46], although we are not aware of studies demonstrating epigenetic changes in the zebrafish following immunization. There might also be benefits to comparing the epigenetic changes that occur following immunization of the zebrafish versus mammalian models as a means to validate the findings in the former. Therefore, this gap area might warrant further study before such approaches can be refined or validated for examining the effects of immunization or become a cutting-edge modern tool for monitoring vaccine safety through epigenetics.
As a practical consideration in the US, the NIH Office of Laboratory Animal Welfare (OLAW) and Public Health Service (PHS) policies apply to egg-laying vertebrates such as zebrafish ‘only after hatching’ at three days post-fertilization [Citation47]. Although institutional animal care and use committees (IACUC) require stringent protocols for adults or breeding adults to produce fish embryos (as they would any other covered animal species), the use of zebrafish embryos could offer an NIH-supported approach for more ethical use of animals in scientific research. Specifically, zebrafish embryos substituting for rodents in toxicology or vaccine screening constitute an approach for addressing the principles of the 3Rs.
Still, another promising aspect of zebrafishes is their use for assessing vaccines or their components for longer-term effects on reproduction or intergenerational transmission [Citation46,Citation48]. Historically, developmental and reproductive toxicity (DART) studies in mammals are expensive and generally conducted only through specialized contractors; there are also limitations on the availability of some animal species for this purpose including non-human primates [Citation49,Citation50]. Zebrafish juveniles and adults have the potential as a vaccine DART model, with gonad differentiation beginning at around 10 days post-fertilization and oocytes visible around 20 days post-fertilization [Citation51–53]. An example is the evaluation of neural tubes and other target or end-organ effects [Citation54]. In the case of neural tube abnormalities, developing zebrafish embryos are exposed to varying doses of a xenobiotic before the beginning of neurulation, with development monitored at post-fertilization time intervals [Citation54,Citation55]. This approach could be refined or modified using vaccines and immunizations as test articles. However, the cellular processes required for zebrafish neural tube development have been shown to differ substantially from humans [Citation56], so the zebrafish might instead be employed for preliminary screening studies or as part of a larger DART initiative alongside more conventional animal species. This approach could enhance the sensitivity for detecting teratogenic effects, with phocomelia after thalidomide exposure as an example [Citation15]. Another option is to evaluate the impact of the zebrafish maternal immunity on embryogenesis or teratogenicity [Citation57].
4. Adjuvant selection and innate immunity
Zebrafishes have been employed to assess immune responses to a variety of viral and bacterial pathogens [Citation58], although less so for vaccines and immunization [Citation20,Citation57]. However, there is a small but relevant literature examining the effects of immunization using a variety of different antigen or vaccine delivery routes, including intramuscular or intraperitoneal injections, oral delivery, or whole fish immersion [Citation57]. Most of these studies examine innate immunity because of the similarities between zebrafishes and mammals in their myeloid precursors and cell types required [Citation19]. However, there are also important differences. For instance, many fish exhibit low sensitivity to lipopolysaccharide (LPS), due to negative regulators of LPS signaling or failures in LPS recognition [Citation59]. Like mammals, zebrafishes can detect both bacteria and viruses through pattern recognition receptors (PRRs) including toll-like receptors (TLRs) [Citation60]. TLRs are expressed on multiple immunocompetent cell types responsible for innate immunity; TLRs are also found in epithelial and other cell types. At least 20 TLR zebrafish genes have been identified including those corresponding to TLR1, TLR3, TLR4, TLR5, TLR7, and TLR11 [Citation19]. Both mammalian and zebrafish TLR3 recognize double-stranded RNA (dsRNA), whereas zebrafish TLR4 does not bind to LPS [Citation19]. Therefore, the zebrafish may not be useful for evaluating TLR-4 agonists used in some adjuvants such as monophosphoryl lipid A. Both mammalian and zebrafish TLR5 bind to flagellin, and both TLR9s bind to CpG oligodeoxynucleotides (CPGs) [Citation19]. However, it is unclear if the zebrafish TLR7 and TLR8 bind to single-stranded RNA (ssRNA), whereas this is the case for mammalian TLR7 and TLR8. In addition, zebrafishes have multiple subfamily members of TLR11. They include TLR20 for possible parasitic infections; TLR21, which also binds CpGs although possibly with different sequence specificities compared with TLR9; and TLR22, which like TLR3 recognizes dsRNA, although of shorter length than TLR3 [Citation19]. This information has relevance for employing the zebrafish to test adjuvants and other immunostimulants. Shown in is a comparison between the TLRs of humans and zebrafishes, their natural ligands, and their corresponding ligands for adjuvant development. In addition, there are three commonly used adjuvants for human vaccines – alum, MF59, and QS21 or other saponin derivatives such as Matrix-M – which may not generate innate immune responses through (membrane-bound) TLRs [Citation61] but instead may stimulate inflammasome NOD-like receptors (NLRs) found in the cell cytosol [Citation62,Citation63]. The zebrafishes also possess NLRs [Citation64], as well as novel immune-type receptors (NITRs) not found in mammals [Citation19].
Table 1. Comparison of TLRs (and NLRs) between humans and zebrafishes.
For innate immune responses to vaccines, zebrafish offer several attractive features to 1) study the mechanisms of new or existing adjuvants, and 2) screen new or existing adjuvants for targeting a specific innate immune response, especially as the demand for new or specific adjuvants expands. Rational adjuvant design includes the new field of ‘immuno-engineering’ for developing new natural or synthetic materials [Citation65], and the zebrafish could potentially serve as a rapid screen for these newly engineered products. Conversely, the zebrafish could help to down-select adjuvants with undesirable profiles, such as those that might contribute to Th2 or Th17 immune enhancement as specified by the NIH Accelerating COVID-19 Therapeutic Interventions and Vaccines (ACTIV) Working Group for COVID vaccines [Citation66]. The zebrafish could also be employed to determine whether adjuvants are required for a particular antigen if the antigen alone is sufficient to trigger adequate innate immunity or determine whether formulating antigens with adjuvants can alter the properties of each component as the two are combined.
To date, the zebrafish model has not yet been validated for innate immune responses to vaccines or adjuvants, but there is evidence to indicate that it holds promise on this front. Our best indication is the finding that zebrafish larvae can be infected with some pathogenic organisms resulting in innate immune responses that resemble those of humans. One of the major ones under study in the zebrafish is Mycobacterium marinum, a slow-growing organism found in salt water that closely resembles M. tuberculosis and causes human skin infections, but in fish produces organized granulomas and a systemic illness that resembles human tuberculosis [Citation67–69]. This infection can be modeled in the zebrafish after they are infected at 36–48 hours post-fertilization through hindbrain ventricle injection, although they can also be infected via the caudal vein inoculations [Citation69]. Assay readouts can include direct microscopy, macrophage recruitment or other cellular infiltration, inducible nitric oxide, and other innate cellular metrics [Citation69]. They could also include innovative assays for shifts in metabolomics patterns as recently described for experimental Trypanosoma cruzi infections to cause Chagas disease in mice [Citation70]. Using a similar approach, the epigenetic response to shigellosis infections in zebrafish neutrophils has been evaluated [Citation71].
For analyzing vaccines and responses to immunizations, zebrafish do not have lymph nodes but they possess a hindbrain ventricle, a cavity filled with cerebrospinal fluid into which immune cells can be recruited [Citation72,Citation73]. This is a convenient injection site to study host and viral factors involved in local and whole-body immune response and innate immunity [Citation73]. For vaccine adjuvants, these are injected in zebrafish larval hindbrains and compared, either alone or formulated with antigens. As one example, polyinosinic:polycytidylic acid or ‘poly (I:C)’ forms a synthetic dsRNA and TLR3 agonist that has been employed as a powerful experimental vaccine adjuvant in mammals. In zebrafish larvae, poly (I:C) was shown to induce epigenetic modifications, while influencing macrophage (but not neutrophil populations) [Citation74]. To validate the zebrafish as a model for studying TLR3 agonists, such modifications might need direct (head-to-head) comparisons with injections in mice or other animal models, or human immune responses. Similar studies could be employed to assess a range of immunostimulants used in both experimental and licensed vaccines. They might include components of the adjuvant system 01 (AS01) used in GlaxoSmithKline vaccines for shingles (Shingrix) or respiratory syncytial virus (RSV, Arexvy), containing monophosphoryl lipid A (MPLA) and QS21 [Citation75], or some of the TLR and NLR agonists currently added to recombinant protein vaccines such as CPGs, 3 M–052, or alum [Citation76]. As highlighted above, the zebrafish could be used to analyze new adjuvants, including NextGen adjuvants from immune-engineered materials [Citation65], and at least one study has used zebrafishes to look at post-exposure sequelae of protective mycobacterial vaccines [Citation77]. For each adjuvant, adjuvant system, or adjuvant-formulated vaccine, myeloid, or other immunocompetent cell populations could be counted and assessed. In this approach, readouts could incorporate transcriptomics, proteomics, metabolomics, or epigenomics. Because of the complex data sets that are likely to emerge from such studies, there could be opportunities to employ machine learning and meta-analysis tools or artificial intelligence (AI) to create analytic and predictive models [Citation78].
5. Antigen selection and adaptive immunity
For antibody responses, the adult zebrafish, like other teleost fish possess IgM and IgD immunoglobulin isotypes, and two recently discovered IgZ-like isotypes – IgZ-1 and IgZ-2; both IgM and IgZ-2 are also found on zebrafish B cell surfaces [Citation79]. In some teleosts, IgZ is named IgT (T for teleost) although there are calls to harmonize this nomenclature [Citation80]. The relative expression and amounts of these isotypes change over the zebrafish lifespan [Citation81]. There is no zebrafish IgG or IgA. Although zebrafish immunoglobulin genes do not undergo class switching [Citation19], there is some evidence for adaptive immunological memory, including enhanced protection and pathogen clearance correlating with a secondary immune response upon reinfection, which is comprised of accelerated antibody production, the expression of igm and igz2 heavy chain immunoglobulin genes, and an il13 Th2 signature cytokine gene [Citation82]. Overall, however, adaptive immune responses are slower in teleosts compared to mammals, with IgM secretion not detected until 4 weeks following post-immunization (using the intraperitoneal route) and antigen-binding B cells appearing not until 10–14 days post-immunization [Citation19]. There are also observed differences in the tissue distribution of the different antibody isotypes, with evidence for mucosal immunity [Citation57]. Mucosal infection or immunization is also possible, and results in the recruitment of B and T cells that resemble mucosa-associated lymphoid tissue (MALT) [Citation57]. Elevated IgZ levels occur in the peripheral serum and skin MALT, while IgZ2 is found in the skin and gill MALTs; these isotypes also vary in terms of their complement dependence or requirement for CD4+ T cells [Citation83]. Zebrafishes also generate cell receptor diversity and there are major histocompatibility complex (MHCI and MHCII) genes [Citation19].
Studies on the role of adaptive immunity and its use to measure humoral immunity to vaccine antigens are hindered by a general absence of both specific immunologic reagents and techniques. Even collecting blood as a survival procedure from the zebrafish is problematic, as only a few microliters can be collected from the adult zebrafish by inserting a heparinized glass capillary tube behind the gills (Dr. Jeff Yoder, personal communication). Greater volumes, however, can be obtained through non-survival methods [Citation84], or possibly by using the entire fish to collect all soluble protein after homogenization in buffer, centrifugation, and analysis of the supernatant, but the feasibility of this approach is unknown. Another challenge is the availability of reagents or kits to measure zebrafish antibodies. Our search identified only just a few vendors offering secondary antibodies or ELISA kits to measure zebrafish IgM antibodies, although there are more reagents and ELISA kits available to measure zebrafish cytokines. In rainbow trout, it was shown that these fish can develop IgM-neutralizing antibodies against a rhabdovirus known as viral hemorrhagic septicemia virus (VHSV) [Citation85]. In this case, only IgM but not IgT exhibited virus-neutralizing properties.
Because of the dearth of available reagents and techniques, it remains unclear whether it might be possible to employ the zebrafish to measure specific antibodies. Using as an example some of the recently developed COVID-19 antigens and vaccines, in the zebrafish, there is uncertainty regarding exactly which antibodies to measure, the optimal time for blood draws, and whether to use survival or non-survival analyses. During the pandemic, our Texas Children’s Hospital Center for Vaccine Development (CVD) developed two recombinant protein COVID-19 vaccine technologies leading to the development and authorization of Corbevax and Indovac that were scaled for production and delivery in India and Indonesia, respectively [Citation76]. Almost 100 million doses were administered in those two countries. Currently, our Texas Children’s CVD is developing NextGen coronavirus vaccines incorporating multiepitope universal and prime-boost strategies. Over the last decade, our group has produced multiple coronavirus antigens corresponding to different beta-coronaviruses or variants using both different adjuvant formulations of recombinant protein and mRNA technologies [Citation86–97]. These antigens can now be evaluated in combination to optimize immunogenicity and epitope broadening, durability, and safety.
Employment of the zebrafish to study either human coronavirus infections or vaccines is still at an early stage, and it is unclear whether a humanized zebrafish producing the appropriate angiotensin-converting enzyme-2 (ACE-2) receptor for virus entry will be required. A human angiotensin-converting enzyme-2 (ace-2) orthologous gene is present in the zebrafish, and zebrafishes respond to both SARS-CoV-2 pseudovirus host entry (through their neuromasts and olfactory organs) and coronavirus recombinant protein antigens through both innate and adaptive immunological mechanisms [Citation73,Citation98–102]. Moreover, recombinant SARS-2 coronavirus spike proteins (or antigens corresponding to their receptor binding domains) induce inflammatory cytokines through both TLR-dependent and independent signaling pathways, recruit neutrophil and macrophages, trigger myelopoiesis, and elicit IgM antibodies [Citation73,Citation98–102]. However, despite the presence of an ACE-2 orthologous receptor in the zebrafish, these animals remain resistant to SARS-2 infection and therefore unsuitable as a virus challenge model for evaluating protective immunity [Citation99]. Accordingly, efforts are underway to generate humanized zebrafish through xenotransplantation of human alveolar epithelial cells or transgenic zebrafish expressing the human ACE-2 receptor [Citation99]. An alternative would be to develop a zebrafish-adapted SARS-2 virus similar to how a mouse-adapted SARS virus was successfully produced [Citation94]. However, even without the availability of transgenic zebrafishes or zebrafish-adapted coronaviruses, it may be possible to evaluate adaptive immunity to new coronavirus vaccine antigen candidates including the ones generated in our laboratories. The advantages of employing the zebrafish for this aspect of COVID-19 vaccine development are similar to those outlined previously for zebrafish vaccine development by de Andrade Belo and Charlie-Silva and include high reproductive capacity – with large numbers of individual animals from a single spawning – to minimize genetic differences in host immunity; transparent embryos; low breeding costs; and significant overlap between human and fish innate and adaptive immune responses [Citation57].
Shown in is a potential list of experiments needed to evaluate adaptive immunity, using COVID-19 antigens as a real-life example. Most likely, this approach would require finding ways to measure specific IgM antibodies, including neutralizing antibodies versus pseudoviruses, and determining whether there are heightened antibody responses following multiple injections. These results would be compared across multiple antigen and adjuvant combinations, with studies to determine the advantage of combining these antigens in ways to induce epitope broadening. In this way, the zebrafish could be accelerated as an innovative model for assessing universal or pan-betacoronavirus vaccines.
Table 2. Gap areas for evaluating acquired immunity to COVID-19 antigens in the adult zebrafish.
6. Conclusion
Four decades of zebrafish research have yielded important new insights in the fields of genetics, genomics, and bioinformatics with relevance to human physiology and increasingly, translational biomedicine for new therapeutics [Citation17]. There are also successes in advancing the zebrafish to address fundamental questions in human infection and immunity, including breakthroughs in the use of the zebrafish for examining host-pathogen relationships for mycobacteria, Shigella, and other bacterial pathogens that can infect both mammals and fish [Citation67–69,Citation71]. Such model systems have tapped into the full range of OMICs capabilities of the zebrafish, including genomics, proteomics, metabolomics, and the study of the vertebrate epigenome.
For vaccinology, however, we are at a much earlier stage. There are multiple components in place to exploit the zebrafish model for vaccine safety studies, much as already happened for small molecule drug toxicology, especially for DART studies, but refinements are still required to make this approach work specifically for vaccines. Similarly, zebrafishes have much to offer for evaluating current and novel vaccine adjuvants or antigen-adjuvant formulations and their effects on host innate immunity, whereas there is still much to do to accelerate the zebrafish for examining adaptive immune responses. Summarized in are some potential uses and limitations in each development stage discussed in the body of the article.
Table 3. Summary table of advantages and disadvantages of embryos versus adults for evaluating vaccine safety as well as innate and adaptive immunity.
Overall, the biomedical literature on these topics is scant, but given the numerous experimental advantages of the zebrafish in terms of high-density animal housing, optical transparency for organ and cell imaging, the availability of a high-quality reference genome, and comprehensive OMICs capabilities, the future f the zebrafish for next-generation vaccine testing could become significant pending the future availability of specific reagents for this purpose.
6.1. Expert opinion
We are entering the post-COVID-19 era and now preparing for future pandemic threats. We should anticipate an urgent need for future vaccines to prevent the zoonotic viruses emerging from bats or other intermediate animal hosts, including coronaviruses, influenza viruses, Nipah viruses, and filoviruses, as well as the arbovirus infections currently accelerating because of climate change and urbanization [Citation103]. New vaccines to counter this next generation of emerging viruses will rely on accelerating a portfolio of new vaccine technologies and rapidly evaluating them for safety, immunogenicity, and protection either through animal challenge studies or clinical trials. Zebrafishes to date have not been a major resource in vaccine and pandemic preparedness, but because of increasing interest in systems vaccinology – the application of systems biology to the study of vaccines [Citation104] – and the adaptability of the zebrafish to study systems biology, there would be potential benefits for examining the zebrafish as a model for systems vaccinology. Zebrafish models are positioned to explore many of these elements, but beyond the urgent need for specific immunological reagents and techniques for measuring adaptive immunity, we may face a reality that the zebrafish system in its current form is still too limited without additional transgenic lines to monitor the different immune lineages. This may require stepped-up efforts to accelerate CRISPR technologies to generate these lines (J. Yoder, personal communication), or other ways to create fish embryos with knocked-out or knocked-down genes required to assess host immunity [Citation19], together with fluorescent labels to examine immunocompetent cell populations and the complex interactions between zebrafish development, exogenous infection, and host metabolism [Citation105].
Systems vaccinology could enhance our current approaches to developing and testing new vaccines. It is too soon to say whether the zebrafish could be the spark that ignites a new tipping point in vaccinology breakthroughs but given this model’s previous track record to date in systems biology, it is worthwhile to begin directing the zebrafish in this yet uncharted direction and path. To begin, the zebrafish could be tapped for examining antigen and adjuvant toxicology and for analyzing innate immunity to new and existing adjuvants, either alone or formulated with vaccine antigens. Examining adaptive immunity to new vaccines is at an earlier stage. The zebrafish community, much like the Caenorhabditis elegans biologists, is collegial, interactive, and enthusiastic about seeing the zebrafish advance in new areas of biomedicine. Taking the first steps in vaccine safety testing, exploring how new information about vaccine antigens and adjuvants can affect zebrafish innate and adaptive immune systems, and using the abundance of specific reagents and OMICs capabilities could lead to new insights in vaccinology. Pending some of the next steps outlined here, the prospect of the zebrafish for shaping the next wave of systems vaccinology studies could become clearer.
Article highlights
The zebrafish represents an innovative model for the study of systems biology and other modern frameworks in biomedicine, but so far this model has been underexplored for human vaccine development and testing.
The zebrafishes exhibit significant similarity to the human genome – approximately 70% of human genes have a zebrafish equivalent – as well as major components of mammalian innate and adaptive immune systems, with the added advantage of suitability for high-density animal housing and potentially addressing the principles of replacement, reduction, and refinement (3Rs).
For vaccine safety, the zebrafish could be adapted to examine the effects of vaccines and adjuvants on organ safety due to the optical transparency of the zebrafish’s embryonic and larval stages.
For innate immunity, the zebrafish offers refined ways to look at adjuvants, adjuvant systems, or adjuvanted vaccines on toll-like receptors (TLRs) or NOD-like receptors (NLRs) on myeloid cells or their OMICs. This could allow for rationally selecting existing adjuvants or new synthetic adjuvants through immuno-engineering.
For studying adaptive immunity, the zebrafish, like other teleost fish, produces IgM, IgD, and two types of IgZ immunoglobulins. This aspect of zebrafish immunology is not as well studied due to less availability of immunological reagents and kits.
Through a well-referenced zebrafish genome, transcriptome, proteome, metabolome, and epigenome (collectively known as ‘OMICs’), together with transgenic manipulation, the availability of genetic knock-outs and knock-downs, and possibly artificial intelligence applied to zebrafish bioinformatics, these teleosts have the potential for the study of systems vaccinology.
Declaration of interest
The team of scientists at Texas Children’s Hospital Center for Vaccine Development including its co-directors, Professors P Hotez and M E Bottazzi, are co-inventors of neglected tropical disease vaccines and a COVID-19 recombinant protein vaccine technology owned by Baylor College of Medicine (BCM). The COVID-19 vaccine technology was recently licensed by BCM non-exclusively and with no patent restrictions to several companies committed to advancing vaccines for low- and middle-income countries. The co-inventors have no involvement in license negotiations conducted by BCM. Similar to other research universities, a long-standing BCM policy provides its faculty and staff, who make discoveries that result in a commercial license, a share of any royalty income. Any such distribution will be undertaken following BCM policy. The authors have no other relevant affiliations or financial involvement with any organization or entity with a financial interest in or financial conflict with the subject matter or material discussed in the manuscript.
Reviewer disclosures
Peer reviewers on this manuscript have no relevant financial or other relationships to disclose.
Author contributions
PJ Hotez wrote the first draft of the article. All authors contributed to the conception and design of the review article and interpreting the relevant literature and have been involved in writing the review article or revising it for intellectual content.
Disclaimers
The findings and conclusions in this report are those of the authors and do not necessarily represent the official position of other entities.
Acknowledgments
The authors would like to acknowledge the thoughts and advice of the following individuals who responded to email queries regarding the potential for zebrafishes to serve as models for vaccine development and testing: Dr. Jeff Yoder, North Carolina State University, College of Veterinary Medicine, Dr. David Tobin, Duke University School of Medicine, Dr. Lalita Ramakrishnan, Cambridge University.
Additional information
Funding
References
- Hotez PJ, Gilbert S, Saville M, et al. COVID-19 vaccines and the pandemic: lessons learnt for other neglected diseases and future threats. BMJ Glob Health. 2023;8(6):e011883. doi: 10.1136/bmjgh-2023-011883
- Hogan MJ, Pardi N. mRNA vaccines in the COVID-19 pandemic and beyond. Annu Rev Med. 2022;73(1):17–39. doi: 10.1146/annurev-med-042420-112725
- Jacob-Dolan C, Barouch DH. COVID-19 Vaccines: Adenoviral Vectors. Annu Rev Med. 2022 Jan 27;73:41–54. doi: 10.1146/annurev-med-012621-102252
- Hotez PJ, Bottazzi ME. Whole inactivated virus and protein-based COVID-19 vaccines. Annu Rev Med. 2022;73:55–64. doi: 10.1146/annurev-med-042420-113212
- Hotez PJ. Global vaccine access demands combating both inequity and hesitancy. Health Aff. 2023;42(12):1681–1688. doi: 10.1377/hlthaff.2023.00775
- Dzau V, Swaminathan S, Baker C, et al. The 100 days mission: how a new medical-countermeasures network can deliver equity and innovation. Lancet. 2023;402(10412):1507–1510. doi: 10.1016/S0140-6736(23)01775-0
- Lee B, Nanishi E, Levy O, et al. Precision vaccinology approaches for the development of adjuvanted vaccines targeted to distinct vulnerable populations. Pharmaceutics. 2023;15(6):1766. doi: 10.3390/pharmaceutics15061766
- Casanova JL, Anderson MS. Unlocking life-threatening COVID-19 through two types of inborn errors of type I IFNs. J Clin Invest. 2023;133(3):e166283. doi: 10.1172/JCI166283
- Black S, Bloom DE, Kaslow DC, et al. Transforming vaccine development. Semin Immunol. 2020;50:101413. doi: 10.1016/j.smim.2020.101413
- Rappuoli R, De Gregorio E, Del Giudice G, et al. Vaccinology in the post-COVID-19 era. Proc Natl Acad Sci USA. 2021;118(3):e2020368118.
- Hubrecht RC, Carter E. The 3Rs and humane experimental technique: implementing change. Animals (Basel). 2019;9(10):754. doi: 10.3390/ani9100754
- Tang B, More V. Recent advances in drug discovery toxicology. Int J Toxicol. 2023;42(6):535–550. doi: 10.1177/10915818231189659
- Bondue T, Berlingerio SP, van den Heuvel L, et al. The zebrafish embryo as a model organism for testing mRNA-based therapeutics. Int J Mol Sci. 2023;24(13):11224. doi: 10.3390/ijms241311224
- Howe K, Clark MD, Torroja CF, et al. The zebrafish reference genome sequence and its relationship to the human genome. Nature. 2013;496(7446):498–503. doi: 10.1038/nature12111. Erratum in: Nature. 2014 Jan 9;505(7482):248. Cooper, James [corrected to Cooper, James D]; Eliott, David [corrected to Elliot, David]; Mortimer, Beverly [corrected to Mortimore, Beverley]; Begum, Sharmin [added]; Lloyd, Christine [added]; Lanz, Christa [added]; Raddatz, Günter [added]; Schuster, Ste.
- Dong W, Akasaka I, Komiyama A, et al. Augmentation of pectoral fin teratogenicity by Thalidomide in human cytochrome P450 3A-Expressing zebrafish. Pharmaceuticals (Basel). 2023;16(3):368. doi: 10.3390/ph16030368
- Streisinger G, Walker C, Dower N, et al. Production of clones of homozygous diploid zebra fish (brachydanio rerio). Nature. 1981;291(5813):293–296. doi: 10.1038/291293a0
- Brown K. An interview with Christiane Nüsslein-Volhard. Development. 2017;144(21):3851–3854. doi: 10.1242/dev.159582
- Zebrafish Core [Internet]. Houston: Baylor College of Medicine; 2024 [cited 2024 Jan 13]. Available from: https://www.bcm.edu/research/atc-core-labs/zebrafish-core.
- Traver D, Yoder J. Immunology (chapter 19) In: the zebrafish in biomedical research: biology, husbandry, diseases, and research applications. Am Coll Lab Animal Med. Academic Press. 2020. 191–216.
- Bailone RL, Fukushima HCS, Ventura Fernandes BH, et al. Zebrafish as an alternative animal model in human and animal vaccination research. Lab Anim Res. 2020;36(1):13. doi: 10.1186/s42826-020-00042-4
- D’Costa A, Shepherd IT. Zebrafish development and genetics: introducing undergraduates to developmental biology and genetics in a large introductory laboratory class. Zebrafish. 2009;6(2):169–177. doi: 10.1089/zeb.2008.0562
- Lai KP, Gong Z, Tse WKF. Zebrafish as the toxicant screening model: transgenic and omics approaches. Aquat Toxicol. 2021;234:105813. doi: 10.1016/j.aquatox.2021.105813
- Baranasic D, Hörtenhuber M, Balwierz PJ, et al. Multiomic atlas with functional stratification and developmental dynamics of zebrafish cis-regulatory elements. Nat Genet. 2022;54(7):1037–1050. doi: 10.1038/s41588-022-01089-w
- Bogdanović O, Fernández-Miñán A, Tena JJ, et al. The developmental epigenomics toolbox: ChIP-seq and MethylCap-seq profiling of early zebrafish embryos. Methods. 2013;62(3):207–215. doi: 10.1016/j.ymeth.2013.04.011
- Link V, Shevchenko A, Heisenberg CP. Proteomics of early zebrafish embryos. BMC Dev Biol. 2006;6:1. doi: 10.1186/1471-213X-6-1
- Harvey SA, Sealy I, Kettleborough R, et al. Identification of the zebrafish maternal and paternal transcriptomes. Development. 2013;140(13):2703–2710. doi: 10.1242/dev.095091
- Spanjaard B, Hu B, Mitic N, et al. Simultaneous lineage tracing and cell-type identification using CRISPR-Cas9-induced genetic scars. Nat Biotechnol. 2018;36(5):469–473.
- Farrell JA, Wang Y, Riesenfeld SJ, et al. Single-cell reconstruction of developmental trajectories during zebrafish embryogenesis. Science. 2018;360(6392):eaar3131. doi: 10.1126/science.aar3131
- Farnsworth DR, Saunders LM, Miller AC. A single-cell transcriptome atlas for zebrafish development. Dev Biol. 2020;459(2):100–108. doi: 10.1016/j.ydbio.2019.11.008
- Saunders LM, Srivatsan SR, Duran M, et al. Embryo-scale reverse genetics at single-cell resolution. Nature. 2023;623(7988):782–791. doi: 10.1038/s41586-023-06720-2
- Lange M, Granados A, VijayKumar S, et al. Zebrahub – multimodal zebrafish developmental atlas reveals the state-transition dynamics of late-vertebrate pluripotent axial progenitors. bioRxiv. 2023.03.06.531398. doi: 10.1101/2023.03.06.531398
- Zebrahub [Internet]. San Francisco: Chan Zuckerberg Biohub; 2024 [cited 2024 Jan 13]. Available from: https://zebrahub.ds.czbiohub.org/.
- Bambino K, Chu J. Zebrafish in toxicology and environmental health. Curr Top Dev Biol. 2017;124:331–367. doi: 10.1016/bs.ctdb.2016.10.007
- Miyawaki I. Application of zebrafish to safety evaluation in drug discovery. J Toxicol Pathol. 2020;33(4):197–210. doi: 10.1293/tox.2020-0021
- Organisation of Economic Cooperation and Development (OECD). Test No. 236: fish embryo acute toxicity (FET) test, OECD guidelines for the testing of chemicals, section 2, 2013. Paris: OECD Publishing; [cited Jan 7, 2024]. doi: 10.1787/9789264203709-en
- Prior H, Haworth R, Labram B, et al. Justification for species selection for pharmaceutical toxicity studies. Toxicol Res (Camb). 2020;9(6):758–770.
- Prior H, Baldrick P, Beken S, et al. Opportunities for use of one species for longer-term toxicology testing during drug development: a cross-industry evaluation. Regul Toxicol Pharmacol. 2020;113:104624. doi: 10.1016/j.yrtph.2020.104624
- Guo M, Xiong M, Peng J, et al. Multi-omics for COVID-19: driving development of therapeutics and vaccines. Natl Sci Rev. 2023;10(9):nwad161. doi: 10.1093/nsr/nwad161
- Parab A, Kumar Bhatt L, Omri A. Targeting epigenetic mechanisms: a boon for cancer immunotherapy. Biomedicines. 2023;11(1):169. doi: 10.3390/biomedicines11010169
- Sopel N, Müller-Deile J. The zebrafish model to understand epigenetics in renal diseases. Int J Mol Sci. 2021;22(17):9152. doi: 10.3390/ijms22179152
- Ping S, Lin W, Ming R, et al. Toxic effects of four cardiovascular drugs on the development and epigenetics of zebrafish (danio rerio). Sci Total Environ. 2022;846:157360. doi: 10.1016/j.scitotenv.2022.157360
- Wang S, Bryan C, Xie J, et al. Atrazine exposure in zebrafish induces aberrant genome-wide methylation. Neurotoxicol Teratol. 2022;92:107091. doi: 10.1016/j.ntt.2022.107091
- Akemann C, Meyer DN, Gurdziel K, et al. TCDD-induced multi- and transgenerational changes in the methylome of male zebrafish gonads. Environ Epigenet. 2020;6(1):dvaa010. doi: 10.1093/eep/dvaa010
- Falisse E, Ducos B, Stockwell PA, et al. DNA methylation and gene expression alterations in zebrafish early-life stages exposed to the antibacterial agent triclosan. Environ Pollut. 2018;243(Pt B):1867–1877. doi: 10.1016/j.envpol.2018.10.004
- Aluru N. Epigenetic effects of environmental chemicals: insights from zebrafish. Curr Opin Toxicol. 2017;6:26–33. doi: 10.1016/j.cotox.2017.07.004
- Terrazas-Salgado L, García-Gasca A, Betancourt-Lozano M, et al. Epigenetic transgenerational modifications induced by xenobiotic exposure in zebrafish. Front Cell Dev Biol. 2022;10:832982. doi: 10.3389/fcell.2022.832982
- US. National institutes of health office of laboratory animal welfare. Zebrafish; [cited 2023 Dec 24]. Available from: https://olaw.nih.gov/policies-laws/21st-century-cures-act/Zebrafish
- Xu B, Pu M, Jiang K, et al. Maternal or paternal antibiotics? Intergenerational transmission and reproductive toxicity in zebrafish. Environ Sci Technol. 2023 Dec 19. doi: 10.1021/acs.est.3c06090. Epub ahead of print. PMID: 38113251.
- Stebbings R, Maguire S, Armour G, et al. Developmental and reproductive safety of AZD1222 (ChAdox1 nCoV-19) in mice. Reprod Toxicol. 2021;104:134–142. doi: 10.1016/j.reprotox.2021.07.010
- Ackley D, Birkebak J, Blumel J, et al. FDA and industry collaboration: identifying opportunities to further reduce reliance on nonhuman primates for nonclinical safety evaluations. Regul Toxicol Pharmacol. 2023;138:105327. doi: 10.1016/j.yrtph.2022.105327
- Ye M, Chen Y. Zebrafish as an emerging model to study gonad development. Comput Struct Biotechnol J. 2020;18:2373–2380. doi: 10.1016/j.csbj.2020.08.025
- Dranow DB, Hu K, Bird AM, et al. Bmp15 is an oocyte-produced signal required for maintenance of the adult female sexual phenotype in zebrafish. PloS Genet. 2016;12(9):e1006323. doi: 10.1371/journal.pgen.1006323
- Wilson CA, Batzel P, Postlethwait JH. Direct male development in chromosomally ZZ zebrafish. Front Cell Dev Biol. 2024;12. doi: 10.1101/2023.12.27.573483
- Rajesh V, Divya PK. Embryonic exposure to decitabine induces multiple neural tube defects in developing zebrafish. Fish Physiol Biochem. 2023;49(6):1357–1379. doi: 10.1007/s10695-023-01261-x Epub ahead of print.
- Valdivieso A, Caballero-Huertas M, Moraleda-Prados J, et al. Exploring the effects of rearing densities on epigenetic modifications in the zebrafish gonads. Int J Mol Sci. 2023;24(21):16002. doi: 10.3390/ijms242116002
- MacGowan J, Cardenas M, Williams MK. Vangl2 deficient zebrafish exhibit hallmarks of neural tube closure defects. bioRxiv [Preprint]. 2023. doi:10.1101/2023.11.09.566412. PMID: 37986956; PMCID: PMC10659374.
- de Andrade Belo MA, Charlie-Silva I. Teleost fish as an experimental model for vaccine development. Methods Mol Biol. 2022;2411:175–194. doi: 10.1007/978-1-0716-1888-2_10
- Sullivan C, Soos BL, Millard PJ, et al. Modeling virus-induced inflammation in zebrafish: a balance between infection control and excessive inflammation. Front Immunol. 2021;12:636623. doi: 10.3389/fimmu.2021.636623
- Martínez-López A, Tyrkalska SD, Alcaraz-Pérez F, et al. Evolution of LPS recognition and signaling: the bony fish perspective. Dev Comp Immunol. 2023 Aug;145:104710. doi: 10.1016/j.dci.2023.104710. Epub 2023 Apr 18. PMID: 37080369.
- Weber ANR. Fish TLR5 develops a taste for viral RNA. EMBO Rep. 2022;23(8):e55443. doi: 10.15252/embr.202255443
- Mosaheb MM, Reiser ML, Wetzler LM. Toll-like receptor ligand-based vaccine adjuvants require intact MyD88 signaling in antigen-presenting cells for germinal center formation and antibody production. Front Immunol. 2017;8:225. doi: 10.3389/fimmu.2017.00225
- Marty-Roix R, Vladimer GI, Pouliot K, et al. Identification of QS-21 as an inflammasome-activating molecular component of saponin adjuvants. J Biol Chem. 2016;291(3):1123–1136. doi: 10.1074/jbc.M115.683011
- Zhu W, Dong C, Wei L, et al. Promising adjuvants and platforms for influenza vaccine development. Pharmaceutics. 2021;13(1):68. doi: 10.3390/pharmaceutics13010068
- Chang MX, Xiong F, Wu XM, et al. The expanding and function of NLRC3 or NLRC3-like in teleost fish: recent advances and novel insights. Dev Comp Immunol. 2021 Jan;114:103859. doi: 10.1016/j.dci.2020.103859
- Slezak A, Chang K, Hossainy S, et al. Therapeutic synthetic and natural materials for immunoengineering. Chem Soc Rev. 2024 Jan 3;53(4):1789–1822. doi:10.1039/d3cs00805c. Epub ahead of print.
- Haynes BF, Corey L, Fernandes P, et al. Prospects for a safe COVID-19 vaccine. Sci Transl Med. 2020;12(568):eabe0948. doi: 10.1126/scitranslmed.abe0948
- Flynn JL. Lessons from experimental mycobacterium tuberculosis infections. Microbes Infect. 2006;8(4):1179–1188. doi: 10.1016/j.micinf.2005.10.033
- Swaim LE, Connolly LE, Volkman HE, et al. Mycobacterium marinum infection of adult zebrafish causes caseating granulomatous tuberculosis and is moderated by adaptive immunity. Infect Immun. 2006;74(11):6108–6117. doi: 10.1128/IAI.00887-06. Erratum in: Infect Immun. 2007 Mar;75(3):1540.
- Cambier CJ, Takaki KK, Larson RP, et al. Mycobacteria manipulate macrophage recruitment through coordinated use of membrane lipids. Nature. 2014;505(7482):218–222. doi: 10.1038/nature12799
- Liu Z, Ulrich vonBargen R, Kendricks AL, et al. Localized cardiac small molecule trajectories and persistent chemical sequelae in experimental chagas disease. Nat Commun. 2023;14(1):6769. doi: 10.1038/s41467-023-42247-w. PMID: 37880260.
- Gomes MC, Brokatzky D, Bielecka MK, et al. Shigella induces epigenetic reprogramming of zebrafish neutrophils. Sci Adv. 2023;9(36):eadf9706. doi: 10.1126/sciadv.adf9706
- Renshaw SA, Trede NS. A model 450 million years in the making: zebrafish and vertebrate immunity. Dis Model Mech. 2012;5(1):38–47. doi: 10.1242/dmm.007138
- Tyrkalska SD, Martínez-López A, Arroyo AB, et al. Differential proinflammatory activities of spike proteins of SARS-CoV-2 variants of concern. Sci Adv. 2022;8(37):eabo0732. doi: 10.1126/sciadv.abo0732 Erratum in: Sci Adv. 2022 Dec 23;8(51):eadf9695.
- Wang Z, You X, Zhang Y, et al. Poly(I: C) induces anti-inflammatory response against secondary LPS challenge in zebrafish larvae. Fish Shellfish Immunol. 2023;144:109285. doi: 10.1016/j.fsi.2023.109285. Epub ahead of print. PMID: 38092095.
- Coccia M, Collignon C, Hervé C, et al. Cellular and molecular synergy in AS01-adjuvanted vaccines results in an early IFNγ response promoting vaccine immunogenicity. NPJ Vaccin. 2017 Sep;2(1):25. doi: 10.1038/s41541-017-0027-3 Erratum in: NPJ Vaccines. 2018;3:13.
- Hotez PJ, Adhikari R, Chen WH, et al. From concept to delivery: a yeast-expressed recombinant protein-based COVID-19 vaccine technology suitable for global access. Expert Rev Vaccines. 2023;22(1):495–500. doi: 10.1080/14760584.2023.2217917
- Myllymäki H, Niskanen M, Luukinen H, et al. Identification of protective postexposure mycobacterial vaccine antigens using an immunosuppression-based reactivation model in the zebrafish. Dis Model Mech. 2018;11(3):dmm033175. doi: 10.1242/dmm.033175
- Onoja A, von Gerichten J, Lewis HM, et al. Meta-analysis of COVID-19 metabolomics identifies variations in robustness of biomarkers. Int J Mol Sci. 2023;24(18):14371. doi: 10.3390/ijms241814371
- Hu YL, Xiang LX, Shao JZ. Identification and characterization of a novel immunoglobulin Z isotype in zebrafish: implications for a distinct B cell receptor in lower vertebrates. Mol Immunol. 2010;47(4):738–746. doi: 10.1016/j.molimm.2009.10.010
- Dornburg A, Ota T, Criscitiello MF, et al. From IgZ to IgT: a call for a common nomenclature for immunoglobulin heavy chain genes of ray-finned fish. Zebrafish. 2021;18(6):343–345. doi: 10.1089/zeb.2021.0071
- Zimmerman AM, Moustafa FM, Romanowski KE, et al. Zebrafish immunoglobulin IgD: unusual exon usage and quantitative expression profiles with IgM and IgZ/T heavy chain isotypes. Mol Immunol. 2011;48(15–16):2220–2223. doi: 10.1016/j.molimm.2011.06.441
- Jørgensen LVG, Korbut R, Jeberg S, et al. Association between adaptive immunity and neutrophil dynamics in zebrafish (danio rerio) infected by a parasitic ciliate. PLOS ONE. 2018;13(9):e0203297. doi: 10.1371/journal.pone.0203297
- Ji JF, Hu CB, Shao T, et al. Differential immune responses of immunoglobulin Z subclass members in antibacterial immunity in a zebrafish model. Immunology. 2021;162(1):105–120. doi: 10.1111/imm.13269
- Pedroso GL, Hammes TO, Escobar TD, et al. Blood collection for biochemical analysis in adult zebrafish. J Vis Exp. 2012 63;(63):e3865. doi: 10.3791/3865
- Castro R, Jouneau L, Pham HP, et al. Teleost fish mount complex clonal IgM and IgT responses in spleen upon systemic viral infection. PLOS Pathog. 2013;9(1):e1003098. doi: 10.1371/journal.ppat.1003098
- Chen WH, Du L, Chag SM, et al. Yeast-expressed recombinant protein of the receptor-binding domain in SARS-CoV spike protein with deglycosylated forms as a SARS vaccine candidate. Hum Vaccin Immunother. 2014;10(3):648–658. doi: 10.4161/hv.27464
- Chen WH, Chag SM, Poongavanam MV, et al. Optimization of the production process and characterization of the yeast-expressed SARS-CoV recombinant receptor-binding domain (RBD219-N1), a SARS vaccine candidate. J Pharm Sci. 2017;106(8):1961–1970. doi: 10.1016/j.xphs.2017.04.037
- Nyon MP, Du L, Tseng CK, et al. Engineering a stable CHO cell line for the expression of a MERS-coronavirus vaccine antigen. Vaccine. 2018 [Epub 2018 Feb 26];36(14):1853–1862. doi: 10.1016/j.vaccine.2018.02.065
- Chen WH, Hotez PJ, Bottazzi ME. Potential for developing a SARS-CoV receptor-binding domain (RBD) recombinant protein as a heterologous human vaccine against coronavirus infectious disease (COVID)-19. Hum Vaccin Immunother. 2020;16(6):1239–1242. doi: 10.1080/21645515.2020.1740560
- Chen WH, Wei J, Kundu RT, et al. Genetic modification to design a stable yeast-expressed recombinant SARS-CoV-2 receptor binding domain as a COVID-19 vaccine candidate. Biochim Biophys Acta Gen Subj. 2021;1865(6):129894. doi: 10.1016/j.bbagen.2021.129893
- Pollet J, Chen WH, Versteeg L, et al. SARSCoV-2 RBD219-N1C1: a yeast-expressed SARS-CoV-2 recombinant receptor-binding domain candidate vaccine stimulates virus neutralizing antibodies and T-cell immunity in mice. Hum Vaccin Immunother. 2021;17(8):2356–2366.
- Lee J, Liu Z, Chen WH, et al. Process development and scale-up optimization of the SARS-CoV-2 receptor binding domain-based vaccine candidate, RBD219-N1C1. Appl Microbiol Biotechnol. 2021;105(10):4153–4165.
- Pollet J, Strych U, Chen WH, et al. Receptor-binding domain recombinant protein on alum-CpG induces broad protection against SARS-CoV-2 variants of concern. bioRxiv [Preprint]. doi:10.1101/2021.07.06.451353 2022:2021.07.06.451353. Update in: Vaccine. 2022 May 8.
- Chen WH, Tao X, Agrawal AS, et al. Yeast-expressed SARS-CoV recombinant receptor-binding domain (RBD219-N1) formulated with aluminum hydroxide induces protective immunity and reduces immune enhancement. Vaccine. 2020;38(47):7533–7541. doi: 10.1016/j.vaccine.2020.09.061
- Pino M, Abid T, Pereira Ribeiro S, et al. A yeast expressed RBD-based SARS-CoV-2 vaccine formulated with 3M-052-alum adjuvant promotes protective efficacy in non-human primates. Sci Immunol. 2021;6(61):eabh3634.
- Chen WH, Pollet J, Strych U, et al. Yeast-expressed recombinant SARS-CoV-2 receptor binding domain RBD203-N1 as a COVID-19 protein vaccine candidate. Protein Expr Purif. 2022;190:106003. doi: 10.1016/j.pep.2021.106003
- Thimmiraju SR, Adhikari R, Villar MJ, et al. A recombinant protein XBB.1.5 RBD/Alum/CpG vaccine elicits high neutralizing antibody titers against omicron subvariants of SARS-CoV-2. Vaccines (Basel). 2023;11(10):1557. doi: 10.3390/vaccines11101557
- Choi SSA, Chan HH, Chan CM, et al. Neuromasts and olfactory organs of zebrafish larvae represent possible sites of SARS-CoV-2 pseudovirus host cell entry. J Virol. 2022;96(24):e0141822. doi: 10.1128/jvi.01418-22
- Tyrkalska SD, Candel S, Pedoto A, et al. Zebrafish models of COVID-19. FEMS Microbiol Rev. 2023;47(1):fuac042. doi: 10.1093/femsre/fuac042
- Tyrkalska SD, Martínez-López A, Pedoto A, et al. The spike protein of SARS-CoV-2 signals via Tlr2 in zebrafish. Dev Comp Immunol. 2023;140:104626. doi: 10.1016/j.dci.2022.104626
- Bastos TSB, de Paula AGP, Dos Santos Luz RB, et al. A novel insight on SARS-CoV-2 S-derived fragments in the control of the host immunity. Sci Rep. 2023;13(1):8060. doi: 10.1038/s41598-023-29588-8 Erratum in: Sci Rep. 2023 Jul 25;13(1):12012.
- Ventura Fernandes BH, Feitosa NM, Barbosa AP, et al. Toxicity of spike fragments SARS-CoV-2 S protein for zebrafish: a tool to study its hazardous for human health? Sci Total Environ. 2022;813:152345. doi: 10.1016/j.scitotenv.2021.152345
- Hotez PJ. Preventing the next pandemic: vaccine diplomacy in a time of anti-science. Baltimore, MD: Johns Hopkins University Press; 2021.
- Raeven RHM, van Riet E, Meiring HD, et al. Systems vaccinology and big data in the vaccine development chain. Immunology. 2019;156(1):33–46. doi: 10.1111/imm.13012
- Masud S, Torraca V, Meijer AH. Modeling infectious diseases in the context of a developing immune system. Curr Top Dev Biol. 2017;124:277–329. doi: 10.1016/bs.ctdb.2016.10.006