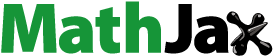
Abstract
Objective
To investigate the correlation between the aryl hydrocarbon receptor (AhR) and reactive oxygen species (ROS) in peripheral blood mononuclear cells (PBMCs) of premature infants, to demonstrate the protective role of AhR against hyperoxia-induced oxidative stress in premature infants and to provide a rational basis for the use of omeprazole (OM) as a new treatment for bronchopulmonary dysplasia (BPD).
Methods
From January 2021 to June 2021, 1–3 ml of discarded peripheral blood was collected from premature infants of gestational age less than 32 weeks who were not taking inhaled oxygen and were admitted to the Department of Neonatology of the Affiliated Hospital of Southwest Medical University. Using a random number table, the PBMCs were randomly assigned to each of the following groups: the control group, air + OM group, hyperoxia group, and hyperoxia + OM group. After 48 h of in vitro modeling and culture, PBMCs and the culture medium of each group were collected. Immunofluorescence analysis was used to examine ROS levels in PBMCs. A full-spectrum spectrophotometer was used to examine malondialdehyde (MDA) levels in the culture medium. Enzyme-linked immunosorbent assay (ELISA) was used to examine monocyte chemotactic protein 1 (MCP-1) levels in culture medium. Immunofluorescence analysis was used to examine the intracellular localization of AhR. Western blotting was used to examine the expression level of AhR in PBMCs.
Results
Compared with those in the control group, the levels of ROS, MDA, and MCP-1 and the cytoplasm-nuclear translocation rate of AhR in the air + OM group did not change significantly (p > 0.05), but the expression level of AhR increased significantly (p < 0.05). The levels of ROS, MDA, and MCP-1 and the cytoplasm-nuclear translocation rate of AhR significantly increased in the hyperoxia group (p < 0.05), and the expression level of AhR was significantly reduced (p < 0.05). Compared with those in the hyperoxia group, the levels of ROS, MDA, and MCP-1 in the hyperoxia + OM group were significantly reduced (p < 0.05), and the cytoplasm-nuclear translocation rate of AhR and the expression level of AhR were significantly increased (p < 0.05), but did not reach the level of the control group (p < 0.05).
Conclusion
OM can activate AhR to inhibit hyperoxia-induced oxidative stress in the PBMCs from premature infants.
1. Background
With the development and promotion of neonatal life support technology and the widespread use of new medical equipment, such as ventilators, the survival rates of preterm infants have continued to increase, as has the risks of oxygen exposure to preterm infants [Citation1]. Exposure to high oxygen levels can induce the mitochondrial respiratory chain to generate a large amount of oxygen free radicals through the leakage mechanism, which are then transformed into reactive oxygen species (ROS) and lead to lipid peroxidation damage and the release of inflammatory factors, thereby inducing cell damage. Premature infants lack antioxidant response kinases, so they cannot effectively eliminate excessive ROS accumulation in the body. ROS can hinder alveolar development and pulmonary angiogenesis and eventually develop into bronchopulmonary dysplasia (BPD), which seriously affects the quality of life of premature infants [Citation2–4]. Because the pathogenesis of BPD is still unclear, there is a lack of specific effective treatment methods in clinical practice.
Aryl hydrocarbon receptor (AhR) is a basic helix-loop-helix (bHLH) Per-Arnt-Sim homology domain (PAS) protein that belongs to the bHLH superfamily of transcription factors. AhR is highly expressed in human lung, thymus, kidney and liver tissue. AhR mainly regulates downstream genes, such as CYP1A1, CYP1A2, GST-α, NQO1, and ALDH, and participates in cell energy metabolism [Citation5–8]. Studies have shown that AhR plays an important role in maintaining the stability of body’s environment [Citation9]; AhR has an anti-inflammatory effect on smoking-induced lung inflammation [Citation10]. AhR has endogenous and exogenous ligands. Due to the different structures and properties of these ligands, different biological effects can occur after they bind with AhR [Citation11].
Omeprazole (OM) is a proton pump inhibitor that is mainly used in the clinic to treat gastric ulcers, duodenal ulcers and other diseases [Citation12]. Its mechanism of action is to inhibit the function of H+/K+-ATP, selectively regulate parietal cell enzymes to inhibit gastric acid secretion, and reduce ROS, thereby alleviating oxidative stress damage in epithelial cells and reducing the inflammatory response induced by NF-κB and STAT3/6 [Citation13–16]. Studies have shown that the AhR agonist OM can inhibit hyperoxia-induced oxidative stress damage by activating AhR and upregulating AhR expression [Citation17].
This study aims to investigate the correlation between AhR and ROS in the PBMCs from premature infants, to demonstrate the protective role of AhR with respect to hyperoxia-induced oxidative stress in premature infants and to provide a rational basis for the use of OM as a new treatment for BPD.
2. Materials and methods
2.1. Materials
The following materials were used: human lymphocyte separation solution (Haoyang Biological Co., Ltd., Tianjin, China), MitoSOXTM Red (Invitrogen, New York, USA), micro malondialdehyde kits (Nanjing Jiancheng Biological Co., Ltd., Nanjing, China), monocyte chemotactic protein 1 (MCP-1) (Duqiao Biological Co., Ltd., Shanghai, China), rabbit anti-human AhR antibodies (Wuhan Boster, Wuhan, China), rhodamine-labeled goat anti-rabbit IgG (H + L) (Zhongshan Jinqiao, Beijing, China), fetal bovine serum (Grand Island Biological Company, New York, USA), phosphate buffered saline (PBS, Soleibao, Beijing, China), a full-spectrum spectrophotometer (Thermo Fisher Scientific, Waltham, USA), a fluorescence microscope (Olympus, Japan), ultralow temperature refrigerators (Thermo Fisher Scientific, Waltham, USA), biological safety cabinets (Thermo Fisher Scientific, Waltham, USA), cell culture incubators (Thermo Fisher Scientific, Waltham, USA), and electrophoresis equipment (Bio-Rad, Hercules, CA).
2.2. Methods
2.2.1. Research object
Forty premature infants of gestational age less than 32 weeks and without oxygen treatment were admitted to the Department of Neonatology of the Affiliated Hospital of Southwest Medical University from January 2021 to June 2021. The inclusion criteria were as follows: premature babies with gestational age <32 weeks and no oxygen; the exclusion criteria were as follows: congenital heart disease and other congenital diseases [Citation18]. There were no statistically significant differences in sex, gestational age, or birth weight among the premature infants in each group (). This study was approved by the Ethics Committee of the Affiliated Hospital of Southwest Medical University (KY2021026), and the family members agreed and signed an informed consent form.
Table 1. Demographic characteristics of premature infants (±s).
2.2.2. Separation of PBMCs by Ficoll density gradient centrifugation
One to three milliliters of discarded peripheral blood was collected with the anticoagulant ethylenediaminetetraacetic acid (EDTA). The collected peripheral blood was thoroughly mixed with Hanks’ balanced salt solution (HBSS) in equal proportions, and PBMCs were collected by Ficoll density gradient centrifugation.
2.2.3. Establishment of cell cultures and the hyperoxia model
PBMCs were collected and randomly assigned into one of the following four groups by the random number table method: control group, air + OM group, hyperoxia group, and hyperoxia + OM group. The control group was placed in a 5% CO2 incubator at 37 °C for 48 h. In the air + OM group, 5 μmol/L OM was added, and the cells were placed in a 5% CO2 incubator for 48 h at 37 °C. In the hyperoxia group, the air mixer was fed CO2 and O2 mixed at a ratio of 5:95, and the cells were placed in a 5% CO2 incubator for 48 h at 37 °C after 10 min of hyperoxia. After 48 h, an ML-IICB digital intelligent oxygen meter was used to examine the culture flask and discard samples with an oxygen concentration of <90%. In the hyperoxia + OM group, 5 μmol/L OM was added and the modeling method was the same as that in the hyperoxia group. After the cells in each group were cultured in vitro for 48 h, the PBMCs and culture medium from each group were collected by centrifugation [Citation18].
2.2.4. Examination of ROS levels in PBMCs by fluorescence microscopy
PBMCs were collected from each group, and the cell density was adjusted to 2 × 106/ml. A total of 200 μl of the cell suspension was evenly spread on an adhered glass slide and fixed with 4% paraformaldehyde for 20 min at room temperature. In a dark environment, relevant solutions were prepared in strict accordance with the MitoSOXTM Red instructions. The cells were evenly coated with MitoSOXTM Red (400 μl), incubated at 37 °C for 30 min, incubated with DAPI at room temperature for 3 min, and mounted with 20 μl of anti-fluorescence quencher. A fluorescence microscope was used to examine the red fluorescence, and Image J analysis software was used to analyze and process the image, determine the average absorbance value and perform statistical analysis.
2.2.5. Examination of malondialdehyde (MDA) levels in culture medium by thiobarbituric acid colorimetry
As the final product of lipid peroxidation, MDA can condense with thiobarbituric acid and form a red product. The culture medium of each group was collected, and the level of MDA in the culture medium was examined by thiobarbituric acid colorimetry. The relevant reagents were prepared in strict accordance with the MDA kit instructions. The samples in each group were placed in a constant-temperature water bath at 95 °C for 80 min, the centrifuge tube was cooled with running water, the supernatant of each group was collected and transferred to a 96-well plate, and 200 μl of sample was added to each well. A full-spectrum spectrophotometer was used to measure the absorbance value of each well at 532 nm. The MDA level in plasma was calculated according to the MDA calculation formula.
2.2.6. Examination of monocyte chemotactic protein 1 (MCP-1) levels in culture medium by enzyme-linked immunosorbent assay (ELISA)
After the MCP-1 kit was rewarmed at room temperature for 30 min, the ELISA plate was removed, 50 μl of standard solution was added to the blank wells in the order of the standards, 50 μl of distilled water was added to the blank control wells, and 40 μl of sample was added to the remaining wells. Then, 10 μl of biotin-labeled antibody was added. After sealing the plate with a sealing film, the plate was incubated at 37 °C for 30 min. After the plate was washed 5 times with the washing solution, 50 μl of enzyme label solution was added to the standard group and sample group, and the plate was sealed with sealing film, placed in a humid box and incubated at 37 °C for 1 h. After being washed 5 times with the washing solution, the color developing solution was added and incubated at 37 °C for 10 min, and 50 μl of stop solution was added. A full-spectrum spectrophotometer was used to measure the absorbance value of each well at 450 nm, and the MCP-1 level in the medium was calculated according to the standard curve.
2.2.7. Examination of the intracellular localization of AhR in PBMCs by fluorescence microscopy
PBMCs were collected from each group, and the cell density was adjusted to 2 × 106/ml. Then, 200 μl of the cell suspension was evenly spread on the adhered glass slide, fixed with 4% paraformaldehyde at room temperature for 20 min, and incubated at room temperature with 0.3% Triton X-100 for 10 min. The cells were blocked with 3% bovine serum albumin at room temperature for 60 min. The cells were evenly covered with the primary antibody (50 μl of rabbit anti-human AhR antibody; 1:50) and incubated overnight at 4 °C in a humid chamber. Then, the attached glass slide was removed, and 50 μl rhodamine-labeled goat anti-rabbit IgG secondary antibody (1:50) was added. The cell layer was evenly covered and incubated at 37 °C in the dark for 60 min. DAPI was added and incubated at room temperature for 3 min, and 20 μl of anti-fluorescence quencher was used to seal the cover glass. Fluorescence microscopy was used to examine the intracellular localization of AhR.
2.2.8. Examination of the AhR protein expression levels in PBMCs by Western blotting
PBMCs from each group were collected and counted in parallel, and 0.1 ml of RIPA buffer was added per 106 cells. The samples were placed on ice for 10 min and then they were sonicated to lyse the cells. The supernatant was collected after centrifugation at 4 °C and 12,000 rpm for 5 min. The protein concentration was determined by the BCA method. The proteins were separated by SDS–PAGE, transferred to a PVDF membrane, and blocked with a blocking solution (5% skim milk powder in TBST) for 1 h. Rabbit anti-human AhR (1:1000) and rabbit anti-human GAPDH antibodies (1:10000) were added and incubated overnight at 4 °C. HRP-labeled goat anti-rabbit IgG (1:2000) was added, incubated at 37 °C for 1 h, and then washed 3 times with TBST for 10 min. The dark room was entered, excess luminescent agent was wiped off, and the film was exposed. Image J analysis software was used to analyze the gray value of the target band, and the results are expressed as AhR/GAPDH and were statistically analyzed.
2.2.9. Statistical analysis
SPSS 21.0 statistical software was used to perform statistical analysis of the data. Measurement data are expressed as the mean ± standard deviation (±s). One-way analysis of variance was used for comparisons between multiple groups, and the LSD-t test was used for pairwise comparisons between groups. p < 0.05 was considered statistically significant. Enumeration data are expressed as percentages (%). The chi-square test was used for comparisons among multiple groups, and p < 0.05 was considered statistically significant; pairwise comparisons between groups were performed using the chi-square test, and p < 0.009 was considered statistically significant.
3. Results
3.1. OM inhibits hyperoxia-induced ROS generation in the PBMCs from premature infants
Immunofluorescence analysis was used to examine the amount of ROS produced by PBMCs, red fluorescence labeled ROS, and blue fluorescence labeled nuclei. The stronger the red fluorescence was, the more ROS were generated (). Compared with the control group (26.25 ± 2.86), the air + OM group (22.33 ± 0.84) had no significant changes in ROS (p > 0.05). Compared with those in the control group, ROS levels in the hyperoxia group increased significantly (46.16 ± 6.59) (p < 0.05). Compared with those in the hyperoxia group, ROS levels in the hyperoxia + OM group (32.54 ± 10.03) were significantly reduced (p < 0.05); however, the levels did not reach those in the control group (p < 0.05) (). These results suggest that OM can inhibit hyperoxia-induced ROS generation in the PBMCs from premature infants.
3.2. OM inhibits hyperoxia-induced MDA production in the PBMCs from premature infants
Compared with the control group (0.99 ± 0.08 nmol/ml), the air + OM group (1.06 ± 0.13 nmol/ml) had no significant changes in MDA levels (p > 0.05). Compared with those in the control group, MDA levels in the hyperoxia group increased significantly (2.19 ± 0.49 nmol/ml) (p < 0.05). Compared with those in the hyperoxia group, MDA levels in the hyperoxia + OM group (1.52 ± 0.29 nmol/ml) were significantly reduced (p < 0.05), but the levels did not reach those of the control group (p < 0.05). These results suggest that OM can inhibit hyperoxia-induced MDA production in the PBMCs from premature infants ().
3.3. OM inhibits hyperoxia-induced MCP-1 production in the PBMCs from premature infants
Compared with the control group (88.13 ± 3.81 pg/ml), the air + OM group (98.18 ± 10.34 pg/ml) had no significant changes in MCP-1 levels (p > 0.05). Compared with those in the control group, MCP-1 levels in the hyperoxia group were significantly increased (133.52 ± 11.85 pg/ml) (p < 0.05). Compared with those in the hyperoxia group, MCP-1 levels in the hyperoxia + OM group (114.84 ± 3.82 pg/ml) were significantly reduced (p < 0.05), but the levels did not reach those of the control group (p < 0.05). OM inhibited hyperoxia-induced MCP-1 production in the PBMCs from premature infants ().
3.4. OM increases the hyperoxia-induced cytoplasm-nuclear translocation of AhR in the PBMCs from premature infants
AhR is labeled with red fluorescence, and the nucleus is labeled with blue fluorescence, which combined forms purple. In the control group, a separated red halo was observed around the nucleus, suggesting that AhR did not undergo cytoplasm-nuclear translocation. In the hyperoxia group, there was no separated red aperture around the nuclei of PBMCs, and uniform red fluorescence was observed in the nucleus, indicating that AhR had undergone cytoplasm-nuclear translocation (). Compared with the control group (18.3%), the air + OM group (17.2%) had no significant change in the AhR cytoplasm-nucleus translocation rate (p > 0.05). Compared with that in the control group, the AhR cytoplasm-nuclear translocation rate in the hyperoxia group (38.5%) was significantly increased (p < 0.05). Compared with that in the hyperoxia group, the AhR cytoplasm-nuclear translocation rate (67.4%) in the hyperoxia + OM group was significantly increased (p < 0.05). OM increased the hyperoxia-induced cytoplasm-nuclear translocation of AhR in the PBMCs from premature infants ().
3.5. OM promotes AhR protein expression in the PBMCs from premature infants
Compared with that in the control group (0.63 ± 0.02), the expression of AhR protein in the air + OM group (0.83 ± 0.02) did not change significantly (p > 0.05). Compared with that in the control group, the protein expression of AhR in the hyperoxia group was significantly reduced (0.23 ± 0.05) (p < 0.05). Compared with that in the hyperoxia group, the protein expression of AhR in the hyperoxia + OM group (0.47 ± 0.05) was significantly increased (p < 0.05), but the level did not reach the level of the control group (p < 0.05). OM upregulated hyperoxia-induced AhR expression in the PBMCs from premature infants ().
4. Discussion
BPD is a common type of chronic lung disease that is mainly characterized by alveolar simplification and pulmonary angiogenesis. BPD is characterized by reduced alveolar numbers, enlarged alveoli, simplified alveolar structure, abnormal pulmonary microvascular morphology and sustained inflammation [Citation19–24]. Oxidative stress is an important factor in the pathogenesis of BPD. Hyperoxia induces the production of large amounts of ROS, which in turn upregulate the expression of proapoptotic proteins, promote the opening of mitochondrial permeability transition pores, lead to lipid peroxidation damage of cell membranes, and even induce apoptosis in alveolar epithelial cells, resulting in long-term chronic lung disease (CLD). Inflammation plays an important role in the pathogenesis of BPD. Hyperoxia can induce the activation of NF-κB, proinflammatory cytokines, and chemotactic proteins, thereby inducing severe lung inflammation and further acute/chronic lung injury [Citation25–28]. Studies have shown that OM can inhibit oxidative stress and has anti-inflammatory effects by activating AhR, which is mainly related to the inhibition of ROS and MCP-1 production, in H441 cells [Citation17、Citation29–31].
In this study, our data show that hyperoxia induced ROS, MDA and MCP-1 generation in the PBMCs from premature infants. ROS, MDA and MCP-1 showed the same trend, which is consistent with previous research by our group. Interestingly, after OM administration, the levels of ROS, MDA and MCP-1 decreased significantly. These findings further demonstrate that OM can reduce the generation of oxygen free radicals in oxidative stress and that OM may reduce hyperoxia-induced oxidative stress damage and inflammatory damage in the PBMCs of preterm infants. AhR is located in the cytoplasm under normal conditions and binds to the heat shock protein 90 (HSP90) dimer and hepatitis B virus X protein-associated protein 2 (XAP2) to form a complex. When AhR is activated, AhR shuttles from the cytoplasm to the nucleus, forming a heterodimer with the aryl hydrocarbon receptor nuclear translocator (ARNT) and initiating the transcription of downstream genes, which are involved in cell energy metabolism [Citation32–34]. Interestingly, our data show that OM promotes the expression of AHR protein, but there is no obvious change in ROS, MDA, MCP-1 and cytoplasm-nuclear shuttling of AhR under normal conditions. However, OM can increase the cytoplasm-nuclear shuttling of AhR and activate AhR activity, thereby inhibiting the hyperoxia-induced generation of ROS, MDA and MCP-1, inhibiting oxidative stress and inducing anti-inflammatory effects.
In summary, OM can activate AhR to inhibit hyperoxia-induced oxidative stress in the PBMCs from premature infants. OM may become a therapeutic agent for the prevention and treatment of BPD in preterm infants. However, OM-mediated inhibition of oxidative stress and the associated specific anti-inflammatory signaling pathways and mechanisms need further experimental and clinical study in premature infants.
Disclosure statement
The authors declare that they have no conflicts of interest. The authors alone are responsible for the content and writing of this article.
Data availability statement
All data included in this study are available upon request by contacting the corresponding author.
Additional information
Funding
References
- Higgins RD. Oxygen saturation and retinopathy of prematurity. Clin Perinatol. 2019;46(3):593–599. doi:10.1016/j.clp.2019.05.008.
- Jobe AH. Mechanisms of lung injury and bronchopulmonary dysplasia. Am J Perinatol. 2016;33(11):1076–1078.
- Wang J, Dong W. Oxidative stress and bronchopulmonary dysplasia. Gene. 2018;678:177–183. doi:10.1016/j.gene.2018.08.031.
- Zhu X, Lei X, Wang J, et al. Protective effects of resveratrol on hyperoxia-induced lung injury in neonatal rats by alleviating apoptosis and ROS production. J Matern Fetal Neonatal Med. 2020;33(24):4150–4158.
- Hui W, Dai Y. Therapeutic potential of aryl hydrocarbon receptor ligands derived from natural products in rheumatoid arthritis. Basic Clin Pharmacol Toxicol. 2020;126(6):469–474. doi:10.1111/bcpt.13372.
- Beránek M, Fiala Z, Kremláček J, et al. Serum levels of aryl hydrocarbon receptor, cytochromes P450 1A1 and 1B1 in patients with exacerbated psoriasis vulgaris. Folia Biol (Praha). 2018;64(3):97–102.
- Miyajima A, Kuroda Y, Sakemi-Hoshikawa K, et al. Inhibitory and inductive effects of 4- or 5-methyl-2-mercaptobenzimidazole, thyrotoxic and hepatotoxic rubber antioxidants, on several forms of cytochrome P450 in primary cultured rat and human hepatocytes. Toxicol Rep. 2020; 7:979–985. doi:10.1016/j.toxrep.2020.08.003.
- Wang K, Lv Q, Miao YM, et al. Cardamonin, a natural flavone, alleviates inflammatory bowel disease by the inhibition of NLRP3 inflammasome activation via an AhR/Nrf2/NQO1 pathway. Biochem Pharmacol. 2018;155:494–509. doi:10.1016/j.bcp.2018.07.039.
- Fujii-Kuriyama Y, Kawajiri K. Molecular mechanisms of the physiological functions of the aryl hydrocarbon (dioxin) receptor, a multifunctional regulator that senses and responds to environmental stimuli. Proc Jpn Acad Ser B Phys Biol Sci. 2010;86(1):40–53. doi:10.2183/pjab.86.40.
- Thatcher TH, Maggirwar SB, Baglole CJ, et al. Aryl hydrocarbon receptor-deficient mice develop heightened inflammatory responses to cigarette smoke and endotoxin associated with rapid loss of the nuclear factor-kappaB component RelB. Am J Pathol. 2007;170(3):855–864. doi:10.2353/ajpath.2007.060391.
- Quintana FJ, Sherr DH. Aryl hydrocarbon receptor control of adaptive immunity. Pharmacol Rev. 2013;65(4):1148–1161. doi:10.1124/pr.113.007823.
- Rahman Z, Dwivedi DK, Jena GB. Ethanol-induced gastric ulcer in rats and intervention of tert-butylhydroquinone: involvement of Nrf2/HO-1 signalling pathway. Hum Exp Toxicol. 2020;39(4):547–562. doi:10.1177/0960327119895559.
- Zhu J, Luo L, Tian L, et al. Aryl hydrocarbon receptor promotes IL-10 expression in inflammatory macrophages through Src-STAT3 signaling pathway. Front Immunol. 2018;9:2033. doi:10.3389/fimmu.2018.02033.
- Patil AS, Singh AD, Mahajan UB, et al. Protective effect of omeprazole and lansoprazole on β-receptor stimulated myocardial infarction in wistar rats. Mol Cell Biochem. 2019;456(1-2):105–113. doi:10.1007/s11010-019-03494-y.
- Badr AM, El-Orabi NF, Ali RA. The implication of the crosstalk of Nrf2 with NOXs, and HMGB1 in ethanol-induced gastric ulcer: potential protective effect is afforded by raspberry ketone. PLoS One. 2019;14(8):e0220548. doi:10.1371/journal.pone.0220548.
- Hashioka S, Klegeris A, McGeer PL. Proton pump inhibitors reduce interferon-γ-induced neurotoxicity and STAT3 phosphorylation of human astrocytes. Glia. 2011;59(5):833–840. doi:10.1002/glia.21157.
- Shivanna B, Chu C, Welty SE, et al. Omeprazole attenuates hyperoxic injury in H441 cells via the aryl hydrocarbon receptor. Free Radic Biol Med. 2011;51(10):1910–1917. doi:10.1016/j.freeradbiomed.2011.08.013.
- Yang X, Dong WB, Lei XP, et al. Resveratrol suppresses hyperoxia-induced nucleocytoplasmic shuttling of SIRT1 and ROS production in PBMC from preterm infants in vitro. J Matern Fetal Neonatal Med. 2018;31(9):1142–1150.
- Hansmann G, Sallmon H, Roehr CC, European Pediatric Pulmonary Vascular Disease Network (EPPVDN)., et al. Pulmonary hypertension in bronchopulmonary dysplasia. Pediatr Res. 2021;89(3):446–455. doi:10.1038/s41390-020-0993-4.
- Rakshasbhuvankar AA, Simmer K, Patole SK, et al. Enteral vitamin a for reducing severity of bronchopulmonary dysplasia: a randomized trial. Pediatrics. 2021;147(1):e2020009985. doi:10.1542/peds.2020-009985.
- Filippone M, Nardo D, Bonadies L, et al. Update on postnatal corticosteroids to prevent or treat bronchopulmonary dysplasia. Am J Perinatol. 2019;36(S 02):S58–S62. Epub 2019 Jun 25. doi:10.1055/s-0039-1691802.
- Ofman G, Caballero MT, Alvarez Paggi D, et al. The discovery BPD (D-BPD) program: study protocol of a prospective translational multicenter collaborative study to investigate determinants of chronic lung disease in very low birth weight infants. BMC Pediatr. 2019;19(1):227. doi:10.1186/s12887-019-1610-8.
- Day CL, Ryan RM. Bronchopulmonary dysplasia: new becomes old again!. Pediatr Res. 2017;81(1-2):210–213. doi:10.1038/pr.2016.201.
- Sahni M, Mowes AK. Bronchopulmonary dysplasia. Treasure Island (FL): StatPearls Publishing; 2021.
- Savani RC. Modulators of inflammation in bronchopulmonary dysplasia. Semin Perinatol. 2018;42(7):459–470. doi:10.1053/j.semperi.2018.09.009.
- Papagianis PC, Pillow JJ, Moss TJ. Bronchopulmonary dysplasia: pathophysiology and potential anti-inflammatory therapies. Paediatr Respir Rev. 2019;30:34–41. doi:10.1016/j.prrv.2018.07.007.
- Yeh TF, Chen CM, Wu SY, et al. Intratracheal administration of budesonide/surfactant to prevent bronchopulmonary dysplasia. Am J Respir Crit Care Med. 2016;193(1):86–95. doi:10.1164/rccm.201505-0861OC.
- Zhao W, Ma L, Cai C, et al. Caffeine inhibits NLRP3 inflammasome activation by suppressing MAPK/NF-κB and A2aR signaling in LPS-Induced THP-1 macrophages. Int J Biol Sci. 2019;15(8):1571–1581. doi:10.7150/ijbs.34211.
- Richter J, Jimenez J, Nagatomo T, et al. Proton-pump inhibitor omeprazole attenuates hyperoxia induced lung injury. J Transl Med. 2016;14(1):247. doi:10.1186/s12967-016-1009-3.
- Busbee PB, Rouse M, Nagarkatti M, et al. Use of natural AhR ligands as potential therapeutic modalities against inflammatory disorders. Nutr Rev. 2013;71(6):353–369. doi:10.1111/nure.12024.
- Bessede A, Gargaro M, Pallotta MT, et al. Aryl hydrocarbon receptor control of a disease tolerance defence pathway. Nature. 2014;511(7508):184–190. doi:10.1038/nature13323.
- Meyer BK, Perdew GH. Characterization of the AhR-hsp90-XAP2 core complex and the role of the immunophilin-related protein XAP2 in AhR stabilization. Biochemistry. 1999;38(28):8907–8917. doi:10.1021/bi982223w.
- Furue M, Hashimoto-Hachiya A, Tsuji G. Aryl hydrocarbon receptor in atopic dermatitis and psoriasis. Int J Mol Sci. 2019;20(21):5424. doi:10.3390/ijms20215424.
- Kondraganti SR, Jiang W, Jaiswal AK, et al. Persistent induction of hepatic and pulmonary phase II enzymes by 3-methylcholanthrene in rats. Toxicol Sci. 2008;102(2):337–344. doi:10.1093/toxsci/kfn007.