Abstract
Background
Bronchopulmonary dysplasia (BPD) has a lasting effect on the respiratory function of infants, imposing chronic health burdens. BPD is influenced by various prenatal, postnatal, and genetic factors. This study explored the connection between BPD and home oxygen therapy (HOT), and then we examined the association between HOT and a specific single-nucleotide polymorphism (SNP) in the hyaluronan and proteoglycan link protein 1 (HAPLN1) gene among premature Japanese infants.
Materials and methods
Prenatal and postnatal data from 212 premature infants were collected and analyzed by four SNPs (rs975563, rs10942332, rs179851, and rs4703570) around HAPLN1 using the TaqMan polymerase chain reaction method. The clinical characteristics and genotype frequencies of HAPLN1 were assessed and compared between HOT and non-HOT groups.
Results
Individuals with AA/AC genotypes in the rs4703570 SNP exhibited significantly higher HOT rates at discharge than those with CC homozygotes (odds ratio, 1.20, 95% confidence interval, 1.07–1.35, p = .038). A logistic regression analysis determined that CC homozygotes in the rs4703570 SNP did not show a statistically significant independent association with HOT at discharge.
Conclusions
Although our study did not reveal a correlation between HAPLN1 and the onset of BPD, we observed that individuals with CC homozygosity at the rs4703570 SNP exhibit a reduced risk of HOT.
Introduction
Bronchopulmonary dysplasia (BPD), a serious complication, can occur in premature infants and negatively affect their prognosis and future respiratory function [Citation1,Citation2]. BPD is widely recognized to disrupt the development of alveolar airspaces [Citation3]. BPD management frequently involves respiratory support, such as supplemental oxygen and mechanical ventilation [Citation4,Citation5].
BPD is a multifaceted condition, where its pathophysiology likely stems from a mixture of intrinsic and extrinsic elements. These may encompass genetic predisposition, perinatal inflammation, as well as exposure to detrimental factors like hyperoxia and mechanical ventilation [Citation6–9].
Various investigations are underway regarding the role of hyaluronan in the extracellular matrix of the developing lung [Citation10–12]. Hyaluronan and proteoglycan link protein 1 (HAPLN1), also referred to as cartilage link protein 1, represents a hyaluronan-binding hyaladherin characterized in cartilage proteoglycan preparations [Citation13,Citation14]. HAPLN1 fortifies the coalescence of aggrecan and hyaluronan in ample multimolecular conglomerates, constituting the pericellular matrix of chondrocytes [Citation15,Citation16]. HAPLN1 loss in mice leads to perinatal lethality resulting from chondrodysplasia [Citation17]. However, specific expression of the Hapln1 transgene in cartilage can thwart perinatal mortality and rectify dose-dependent skeletal anomalies in Hapln1-null mice [Citation18]. HAPLN1 might participate in the fibrous remodeling of proliferating human lung fibroblasts during their transformation into myofibroblasts [Citation19]. Recent research indicates the potential efficacy of recombinant human HAPLN1 in reducing alveolar enlargement in adult mouse models of chronic obstructive pulmonary disease [Citation20]. Therefore, it is proposed that moderate regulation of HAPLN1 expression could impact the onset and severity of BPD.
Single-nucleotide polymorphisms (SNPs) around HAPLN1 have been previously reported to be associated with an increased risk of lung cancer [Citation21] and spinal osteophyte formation and disk space narrowing [Citation22].
HAPLN1 appears to play a crucial role as a regulator of cartilage and pericellular matrix homeostasis in chondrocytes and may be associated with BPD development. In this study, we aimed to investigate the relationship between genetic variations in HAPLN1 and the incidence and severity of BPD.
Materials and methods
Study design and patient groups
This study included 212 infants who were born at a gestational age (GA) of <32 weeks and had a birth weight (BW) of <1250 g at four centers (Saitama Medical Center (SMC), Nihon University Itabashi Hospital (NUIH), University of Occupational and Environmental Health (UOEH), and Akita Red Cross Hospital (ARCH)) between 1 April 2014 and 31 March 2022. Infants with congenital anomalies were excluded. Patients were classified into newborns with home oxygen therapy (HOT) at discharge (HOT group, n = 43) and newborns without HOT (non-HOT group, n = 169). Clinical and HAPLN1 genetic polymorphism data between the HOT and non-HOT groups were analyzed.
Patient clinical data
The clinical data analyzed in this study were obtained from the medical records of the patients. Data included maternal age, antenatal steroid therapy, histological chorioamnionitis, mode of delivery, GA, BW, sex, Apgar score at 1 and 5 min, use of surfactant, BPD according to 2016 NICHD definition [Citation23], and HOT at discharge. At each facility, we contemplated implementing HOT if the 24-h average peripheral oxygen saturation could not be sustained at 95% by the corrected 40 weeks. The initiatives for data collection commenced sequentially at each facility on 4 January 2016, and were concluded on 24 April 2022 (SMC), 2 April 2022 (NUIH), 5 April 2022 (UOEH), and 14 April 2022 (ARCH).
Genomic DNA was extracted from the infant’s blood sample using the Wizard® Genomic DNA Purification Kit (Promega, Fitchburg, WI). Four SNPs (rs975563, rs10942332, rs179851, and rs4703570) around HAPLN1 (NM_001884.4) were selected. These SNPs were located in the 5′ flanking region (rs975563), intron 1 (rs10942332), intron 5 (rs179851), and intron 7 (rs4703570). The genomic location was described with reference to GRCh38.p14 (NC_000005.10). The selected SNPs had minor allele frequencies of ≥0.10 in the Japanese population. For genotyping, real-time polymerase chain reaction (PCR) was performed using ABI 7900HT PCR device and TaqMan SNP assays (Applied Biosystems, Foster City, CA).
Statistical analyses
The patients were categorized into two groups based on the number of minor alleles at the same locus: those with one or two minor alleles and those with only the major allele. Descriptive data are expressed as medians (interquartile range) or frequencies (percentage). To compare the clinical characteristics and outcomes between the genotype groups, the Mann–Whitney U-test, Student’s t-test, or Fisher’s exact probability test was employed as appropriate. Multivariate logistic regression was applied to estimate the risk of HOT. All statistical analysis was conducted using EZR, a modified version of R Commander designed for biostatistics, and a graphical user interface for R (version 2.13.0, R Foundation for Statistical Computing, Vienna, Austria). Linkage disequilibrium (r2) between adjacent HAPLN1 SNPs was analyzed using Haploview (version 4.2) [Citation24].
Results
During the study, 212 patients were selected from four distinct medical institutions (). The median GA and BW of the HOT group were significantly lower than those in the non-HOT group (26 ± 1.7 vs. 27 ± 2.2 weeks, p = .015; 774 ± 235 vs. 818 ± 235 g, p = .043). No significant difference was observed in maternal age and Apgar scores at 1 and 5 min between the two groups. The proportion of male infants, maternal use of prenatal steroids, and surfactant administration did not differ significantly between the groups. However, the HOT group had a significantly higher incidence of histologic chorioamnionitis than the non-HOT group (58% vs. 36%, p = .022) ().
Table 1. Demographic and clinical characteristics of 212 patients.
Table 2. Demographic and clinical characteristics of HOT and non-HOT patients.
The frequencies of the four SNPs conformed to the principles of the Hardy–Weinberg equilibrium. A moderate linkage disequilibrium (r2 = 0.40) was found between rs10942332 and rs975563 (). Among the four SNPs, rs4703570 was associated with HOT at discharge (). Our cohort included 97, 77, and 38 patients with AA, AC, and CC genotypes at rs4703570, respectively. The AA/AC group had significantly higher HOT rates at discharge than the CC group (odds ratio, 1.20; 95% confidence interval, 1.07–1.35, p = .038). However, no noticeable variations were found between the AA/AC and CC groups when considering various prenatal and postnatal factors relevant to HOT at discharge ().
Figure 1. Genomic organization and linkage disequilibrium mapping of single-nucleotide polymorphisms (SNPs) in HAPLN1. The upper diagram shows the structure of HAPLN1 and relative physical position of four SNPs. The pairwise linkage disequilibrium (r2) between the SNPs is shown below each SNP combination.
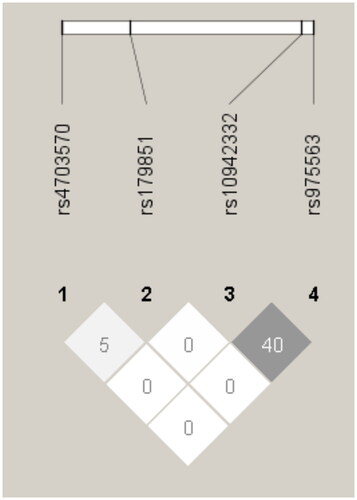
Table 3. Association of each genotype in and near the HAPLN1 gene with home oxygen therapy at discharge.
Table 4. Comparison of patient background and clinical characteristics of different genotypes (AA/AC genotype and CC genotype) of single nucleotide polymorphism (SNP) in intron 7 (rs4703570) of HAPLN1 gene.
A logistic regression analysis was performed to examine the association between the AA/AC group in rs4703570 and use of HOT at discharge. This analysis considered several variables that were significantly different between the groups, including lower GA, sex, and histological chorioamnionitis (). The results revealed that lower GA (odds ratio, 1.20; 95% confidence interval, 1.01–1.43, p = .037) and histological chorioamnionitis (odds ratio, 2.05; 95% confidence interval, 1.20–4.15, p = .043) were significant and independent predictors of HOT at discharge. However, the AA/AC group in rs4703570 did not exhibit a statistically significant independent association with HOT at discharge (odds ratio, 1.67; 95% confidence interval, 0.52–3.230, p = .270).
Table 5. Multivariate logistic regression analysis of BPD risk factors.
Discussion
For this study, data on prenatal and postnatal factors were collected from four healthcare facilities. Multivariate analysis revealed that histological chorioamnionitis independently contributed to the risk of HOT. Recent reviews and studies have increasingly recognized its detrimental effects [Citation25,Citation26]. Furthermore, children with the A allele at rs4703570 have higher risk for requiring HOT at discharge. However, multiple logistic regression analysis did not reveal a significant difference.
Northway et al. introduced the concept of BPD and described it as a condition characterized by dysmorphic microvasculature and alveolar simplification [Citation27]. In 1999, Jobe proposed a new BPD classification involving less airway damage and alveolar septal fibrosis [Citation28]. BPD has multiple underlying causes, and the alteration of lung fibrosis in premature infants is considered a significant contributing factor. According to Lecarpentier et al., both hypoxia and hyperoxia can activate the canonical WNT/β-catenin system and transforming growth factor-β while suppressing peroxisome proliferator-activated receptor-gamma. This impairment of lung maturation leads to the transdifferentiation of lipofibroblasts into myofibroblasts, resulting in pulmonary fibrosis and severe lung complications [Citation29].
Hyaluronan is a polysaccharide or polysaccharide conjugate, and it has been recognized for its role in alveolization, along with heparin, heparan sulfate, and chondroitin sulfate [Citation30,Citation31]. The synthesis of glycosaminoglycans, including hyaluronan, heparan sulfate, and chondroitin sulfate, by lung fibroblasts during lung development was also observed, indicating their involvement in lung epithelial–mesenchymal interactions [Citation32]. Furthermore, Underhill et al. discovered that CD44-positive macrophages, which can take up hyaluronan, regulate its levels during lung development, suggesting a link between hyaluronan and inflammatory cells [Citation33]. However, the relationship between HAPLN1, a hyaluronan-binding protein, and BPD has not been extensively explored. This study suggests a potential association between HAPLN1 and BPD, warranting further in vitro studies and clinical data analysis.
To our understanding, no investigations have delved into the relationship between HAPLN1 polymorphism and BPD. An inadequacy of this research lies in its relatively restricted sample size. Additionally, our current exploration constituted an investigative observation and refrained from executing a power analysis for sample size determination. Moreover, we conjecture that a selection bias might have transpired, wherein neonates with more severe BPD were encompassed in this investigation, consequently amplifying the prevalence of BPD and introducing partiality into the outcomes. Therefore, comprehensive studies on a larger scale are imperative to comprehensively grasp the HAPLN1 genotype-specific impacts on BPD severity.
In conclusion, this multicenter study in premature Japanese infants revealed an association between rs4703570 SNP at intron 7 of HAPLN1 and use of HOT for severe BPD. This study may contribute to the discovery of new diagnostic markers and therapeutic approaches.
Author contributions
This study was conceptualized by FN. AS, HA, AI, EN, NN, SS, SA, AK, and FN obtained blood samples and collected clinical data from the infants, ensuring that proper informed consent was obtained from their families. MH and FN were responsible for processing the blood samples, and MI conducted real-time PCR and performed the statistical procedures. MI prepared the first draft, and all other authors provided substantial input toward developing the final version. YO and FN provided feedback on the methodology. All authors have read and agreed to the published version of the manuscript.
Ethical approval
This retrospective study was conducted under the approval of the ethical committee of Saitama Medical Center (Ethics Approval No. 829), Nihon University Itabashi Hospital (Ethics Approval No. 215-1), University of Occupational and Environmental Health (Ethics Approval No. seH28-01), and Akita Red Cross Hospital (Ethics Approval No. H26-11).
Consent form
Written informed consent was acquired from the parents of the patients.
Disclosure statement
No potential conflict of interest was reported by the author(s).
Data availability statement
All data generated or analyzed during this study are included in this article. Further inquiries can be directed to the corresponding author.
Additional information
Funding
References
- Balany J, Bhandari V. Understanding the impact of infection, inflammation, and their persistence in the pathogenesis of bronchopulmonary dysplasia. Front Med. 2015;2:1. doi: 10.3389/fmed.2015.00090.
- Hirata K, Nishihara M, Shiraishi J, et al. Perinatal factors associated with long-term respiratory sequelae in extremely low birthweight infants. Arch Dis Child Fetal Neonatal Ed. 2015;100(4):F314–6. doi: 10.1136/archdischild-2014-306931.
- Voynow JA. "New" bronchopulmonary dysplasia and chronic lung disease. Paediatr Respir Rev. 2017;24:17–18. doi: 10.1016/j.prrv.2017.06.006.
- Jobe AH. The new bronchopulmonary dysplasia. Curr Opin Pediatr. 2011;23(2):167–172. doi: 10.1097/MOP.0b013e3283423e6b.
- Hennelly M, Greenberg RG, Aleem S. An update on the prevention and management of bronchopulmonary dysplasia. Pediatric Health Med Ther. 2021;12:405–419. doi: 10.2147/PHMT.S287693.
- Hwang JS, Rehan VK. Recent advances in bronchopulmonary dysplasia: pathophysiology, prevention, and treatment. Lung. 2018;196(2):129–138. doi: 10.1007/s00408-018-0084-z.
- Savani RC. Modulators of inflammation in bronchopulmonary dysplasia. Semin Perinatol. 2018;42(7):459–470. doi: 10.1053/j.semperi.2018.09.009.
- Gilfillan M, Bhandari V. Pulmonary phenotypes of bronchopulmonary dysplasia in the preterm infant. Semin Perinatol. 2023;47(6):151810. doi: 10.1016/j.semperi.2023.151810.
- Ito M, Kato S, Saito M, et al. Bronchopulmonary dysplasia in extremely premature infants: a scoping review for identifying risk factors. Biomedicines. 2023;11(2):553. doi: 10.3390/biomedicines11020553.
- Misra S, Hascall VC, Markwald RR, et al. Interactions between hyaluronan and its receptors (CD44, RHAMM) regulate the activities of inflammation and cancer. Front Immunol. 2015;6:201. doi: 10.3389/fimmu.2015.00201.
- Markasz L, Savani RC, Jonzon A, et al. CD44 and RHAMM expression patterns in the human developing lung. Pediatr Res. 2021;89(1):134–142. doi: 10.1038/s41390-020-0873-y.
- Markasz L, Savani RC, Sedin G, et al. The receptor for hyaluronan-mediated motility (RHAMM) expression in neonatal bronchiolar epithelium correlates negatively with lung air content. Early Hum Dev. 2018;127:58–68. doi: 10.1016/j.earlhumdev.2018.10.002.
- Oegema TRJr., Laidlaw J, Hascall VC, et al. The effect of proteoglycans on the formation of fibrils from collagen solutions. Arch Biochem Biophys. 1975;170(2):698–709. doi: 10.1016/0003-9861(75)90167-8.
- Hardingham TE, Muir H. The specific interaction of hyaluronic acid with cartillage proteoglycans. Biochim Biophys Acta. 1972;279(2):401–405. doi: 10.1016/0304-4165(72)90160-2.
- Kwok JC, Carulli D, Fawcett JW. In vitro modeling of perineuronal nets: hyaluronan synthase and link protein are necessary for their formation and integrity. J Neurochem. 2010;114(5):1447–1459. doi: 10.1111/j.1471-4159.2010.06878.x.
- Rauch U, Hirakawa S, Oohashi T, et al. Cartilage link protein interacts with neurocan, which shows hyaluronan binding characteristics different from CD44 and TSG-6. Matrix Biol. 2004;22(8):629–639. doi: 10.1016/j.matbio.2003.11.007.
- Watanabe H, Yamada Y. Mice lacking link protein develop dwarfism and craniofacial abnormalities. Nat Genet. 1999;21(2):225–229. doi: 10.1038/6016.
- Czipri M, Otto JM, Cs-Szabo G, et al. Genetic rescue of chondrodysplasia and the perinatal lethal effect of cartilage link protein deficiency. J Biol Chem. 2003;278(40):39214–39223. doi: 10.1074/jbc.M303329200.
- Evanko SP, Gooden MD, Kang I, et al. A role for HAPLN1 during phenotypic modulation of human lung fibroblasts in vitro. J Histochem Cytochem. 2020;68(11):797–811. doi: 10.1369/0022155420966663.
- Piao Y, Yun SY, Fu Z, et al. Recombinant human HAPLN1 mitigates pulmonary emphysema by increasing TGF-beta receptor I and sirtuins levels in human alveolar epithelial cells. Mol Cells. 2023;46(9):558–572. doi: 10.14348/molcells.2023.0097.
- Jones CC, Bradford Y, Amos CI, et al. Cross-cancer pleiotropic associations with lung cancer risk in African Americans. Cancer Epidemiol Biomarkers Prev. 2019;28(4):715–723. doi: 10.1158/1055-9965.EPI-18-0935.
- Urano T, Narusawa K, Shiraki M, et al. Single-nucleotide polymorphism in the hyaluronan and proteoglycan link protein 1 (HAPLN1) gene is associated with spinal osteophyte formation and disc degeneration in Japanese women. Eur Spine J. 2011;20(4):572–577. doi: 10.1007/s00586-010-1598-0.
- Higgins RD, Jobe AH, Koso-Thomas M, et al. Bronchopulmonary dysplasia: executive summary of a workshop. J Pediatr. 2018;197:300–308. doi: 10.1016/j.jpeds.2018.01.043.
- Barrett JC, Fry B, Maller J, et al. Haploview: analysis and visualization of LD and haplotype maps. Bioinformatics. 2005;21(2):263–265. doi: 10.1093/bioinformatics/bth457.
- Villamor-Martinez E, Alvarez-Fuente M, Ghazi AMT, et al. Association of chorioamnionitis with bronchopulmonary dysplasia among preterm infants: a systematic review, meta-analysis, and metaregression. JAMA Netw Open. 2019;2(11):e1914611. doi: 10.1001/jamanetworkopen.2019.14611.
- Liu PC, Hung YL, Shen CM, et al. Histological chorioamnionitis and its impact on respiratory outcome in very-low-birth-weight preterm infants. Pediatr Neonatol. 2021;62(3):258–264. doi: 10.1016/j.pedneo.2020.11.009.
- Northway WHJr., Rosan RC, Porter DY. Pulmonary disease following respirator therapy of hyaline-membrane disease. Bronchopulmonary dysplasia. N Engl J Med. 1967;276(7):357–368. doi: 10.1056/NEJM196702162760701.
- Jobe AJ. The new BPD: an arrest of lung development. Pediatr Res. 1999;46(6):641–643. doi: 10.1203/00006450-199912000-00007.
- Lecarpentier Y, Gourrier E, Gobert V, et al. Bronchopulmonary dysplasia: crosstalk between PPARgamma, WNT/beta-catenin and TGF-beta pathways; the potential therapeutic role of PPARgamma agonists. Front Pediatr. 2019;7:176. doi: 10.3389/fped.2019.00176.
- Becchetti E, Evangelisti R, Stabellini G, et al. Developmental heterogeneity of mesenchymal glycosaminoglycans (GAG) distribution in chick embryo lung anlagen. Am J Anat. 1988;181(1):33–42. doi: 10.1002/aja.1001810105.
- Smits NC, Shworak NW, Dekhuijzen PN, et al. Heparan sulfates in the lung: structure, diversity, and role in pulmonary emphysema. Anat Rec. 2010;293(6):955–967. doi: 10.1002/ar.20895.
- Caniggia I, Tanswell K, Post M. Temporal and spatial differences in glycosaminoglycan synthesis by fetal lung fibroblasts. Exp Cell Res. 1992;202(2):252–258. doi: 10.1016/0014-4827(92)90072-g.
- Underhill CB, Nguyen HA, Shizari M, et al. CD44 positive macrophages take up hyaluronan during lung development. Dev Biol. 1993;155(2):324–336. doi: 10.1006/dbio.1993.1032.