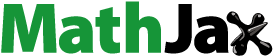
ABSTRACT
The purpose of this study is to evaluate the effect of CIS Building Integrated Photovoltaic as a shading device on building energy performance and PV life cycle. A multi-faceted BIPV energy evaluation approach is created. Energy performance is evaluated in two climate regions, in three scenarios for two transparency ratios of an office building. The case study indicated that when BIPV was used, in mild climates there was 24.82–41.05%, and in hot climates, 35.21–56.18% net energy consumption decreased. The PVs can produce 6.5–9.1 times the amount of energy consumed during manufacturing over the usage period. Finally, it was shown that the CIS technology contributes to sustainable architecture by reducing the integrated design difficulties in front of BIPV.
1. Introduction
Buildings consume a large amount of the energy produced on Earth. In this study, CIS (Copper indium (di)selenide) PV systems as a shading device in the building have been studied as a proposal on a building scale. Shading devices integrated with PV systems have two cohesive functions such as PV as an active system and a shading device as a passive system. The PV system designed as a shading device is BIPV (Building Integrated PV) system. The BIPV system is an integrated design of PV system with a secondary function on the building envelope and this system is a part of the building envelope (Peng, Huang, and Wu Citation2011; Aaditya and Mani Citation2018). It was predicted that shading devices on the building will decrease the cooling load during summer and the PV system will produce electric energy throughout the year and decrease fossil fuel-based energy consumption (buying energy from the grid).
BIPVshading (the BIPV system as a shading device) should be considered as two separate sub-systems: shading and PV. There are shading devices studies as a reference: Shading device type selection is based on direction (Zorer Gedik Citation2002; Grobman, Capeluto, and Austern Citation2016; Elzeyadi Citation2017), the effect of shading devices on building energy (thermal, lighting) loads (Huang, Niu, and Chung Citation2011; Zorer Gedik Citation2001; Ahmad and Reffat Citation2018; Panteli et al. Citation2018; Touma and 2Ouahrani Citation2018).
Many studies evaluated different parameters that affect the efficiency of PV systems (Hwang, Kang, and Kim Citation2011; Wittkopf et al. Citation2012; Kalogirou, Agathokleous, and Panayiotou Citation2013), determining optimum PV module tilt angle (Wang and Chang Citation2010; Mehleri et al. Citation2010; Badescu and Iacobescu Citation2013), building net energy load (Bot et al. Citation2019) and studies on the integration of the PV system (BIPV systems) with a building envelope (Peng, Huang, and Wu Citation2011; Hagemann Citation2004; Biyik et al. Citation2017; Lovati et al. Citation2019) and effect on building net energy load (Vincenzo, Kesten, and Infield Citation2012; Peng et al. Citation2015; Ekoe A Akata, Njomo, and Agrawal Citation2017; Dehwah and Asif Citation2019; Costanzo et al. Citation2018; Vassiliades et al. Citation2018; Braun and Rüther Citation2010; Maghrabie et al. Citation2021).
In BIPV systems used as shading devices, topics and studies could be summarised as follows. The integration of PV material with the shading element was first proposed in 1998, in Korea (Yoo, Lee, and Lee Citation1998). One of the topics studied in this field was BIPVshading evaluation with energy criteria (Vincenzo, Kesten, and Infield Citation2012; Peng et al. Citation2015; Ekoe A Akata, Njomo, and Agrawal Citation2017; Dehwah and Asif Citation2019; Costanzo et al. Citation2018; Vassiliades et al. Citation2018; Braun and Rüther Citation2010; Maghrabie et al. Citation2021; Yoo, Lee, and Lee Citation1998; Yoo and Lee Citation2002). There were optimisation studies about the BIPVshading. Some of them were carried out with the criteria of energy use, PV energy production and daylight illumination (Dino Citation2017; Taveres-Cachat et al. Citation2019). The dynamic BIPVshading (Jayathissa et al. Citation2017) and the movable BIPVshading (Akbari Paydar Citation2020) subjects were studied in the field of optimisation. The effect of BIPVshading on visual comfort (useful daylight illuminance, daylight glare index) with energy criteria was investigated by Mandalaki, Tsoutsos, and Papamanolis (Citation2014). The studies by Mandalaki et al. (Citation2012) and by Fouad, Mohamed, and Shihata (Citation2018) examined the effect of BIPVshading on daylight. The energy effect of the BIPVshading with different tilt angles on the building was analysed by Sun and Yang (Citation2009). Studies to select the most suitable BIPVshading type according to the façade directions are limited to the south façade and a single volume. Different types of BIPVshading were investigated on the south side with the energy efficiency criterion (Mandalaki et al. Citation2012). Various types of shading elements integrated with PV have been investigated with many quantitative (energy) and qualitative (visual-comfort) criteria (Stamatakis, Mandalaki, and Tsoutsos Citation2016). Martinopoulos et al. evaluate in terms of energy consumption and indoor environmental conditions BIPVshading options for an office building (Martinopoulos et al. Citation2018). The life cycle assessment for the photovoltaic integrated shading system was researched by Fouad et al. (Citation2019). Some other studies have been done to evaluate the quality of PV integration into architecture (Custódio et al. Citation2022; Devetaković et al. Citation2020). In their comprehensive review of the BIPV system design, A. K. Shukla, Sudhakar, and Baredar (Citation2016) presented a twelve-step BIPV system design path. However, the step about ‘PV shading devices’ does not include ‘a way to design BIPV shading’. As seen in this review study, it can be said that there is a need for a guiding study on ‘designing BIPV shading’.
Several studies on BIPVshading are similar repeated for different locations (Jung Citation2014; Chang Citation2010; Kim et al. Citation2010). The solar data vary according to the location that is used by the PV system and the shading device design. Since the quality and quantity of incident solar radiation changes according to location, it is hard to make universal assumptions and for that reason, local studies become more important.
The main purpose of this study is to explain the effect of CIS BIPVshading on building energy demand in different climates. In addition, the shadowing effect of this system on the building energy demand is intended to be shown depending on the window-to-wall ratio (WWR). The effect of BIPVshading on building energy consumption is investigated as a whole and separate manner such as the PV function and shading function. Contrary to many studies with a single zone sample, a whole building sample is studied. Since the shading function of the system affects the heating–cooling loads of the building and the zones of the building are in thermal interaction with each other, the building is considered as a whole. One more important point (scope) of this study is working with CIS thin-film PV. CIS is currently regarded as the most innovative technology associated with the serial production of solar modules (Solar Frontier Citation2021). The CIS cells, because of their high efficiency and properties, become more and more popular in BIPV applications (Frydrychowicz–Jastrzębska and Bugała Citation2016). In this study, the most important reasons for choosing CIS technology are the high colour homogeneity (pv Europe Citation2016) and high shadow tolerance (Solar Frontier Citation2021) features that facilitate adaptation to the architecture. CIS technology, with its colour homogeneity, gives PVs the freedom to integrate with architectural design in terms of aesthetics. The shade tolerance feature of CIS technology creates flexibility in positioning the PV and in architectural design against the shading effects of the surroundings. In the meantime, a review article (Zhang et al. Citation2018) on PVs (PVSD) used as shading elements identified eight studies on CIGS (copper indium gallium diselenide, like CIS) PV type and aligned that there is limited knowledge about PVSD. Moreover, a significant feature of the study is the comprehensive analysis and evaluation of energy from the building and PV system aspects of the BIPV system. The main energy indicators such as energy payback time (EPBT) and energy return factor (ERF) were used to evaluate the energy sustainability of the PV system. To sum up, this study takes into consideration the passive systems of the building, energy demand, and energy generated by the photovoltaic, and how they interact in different scenarios. Under energy policies in the World, the importance and request for PV systems are increasing. Therefore, the growing interest in PV systems and the need for local studies are growing the importance of this study. Additionally, unlike other studies, it is shown that the use of per unit BIPVshading area in a building designed according to the climate will add a considerable contribution to decreasing energy consumption. The methodology presented in this study can be used by architects who want to integrate a renewable energy system (PV) into the buildings at the early design phase, and those working in areas such as green buildings, low-energy building, sustainable cities and sustainable buildings. This study is also important for those who want to obtain a green building certificate, as BIPVshading systems can provide points from the parts of green building certification rating systems related to renewable energy and building energy consumption.
2. Methodology
The main purpose of this approach is to evaluate the energy performance of the design with (approximate) ∼100% window-to-wall (transparency) ratio by comparing it with the reference design of an example building with PV shading devices in different climatic regions. The flow diagram of the method is given in .
The flow diagram in is applied to two different climate regions in this study. This flow diagram can be applied to any number of different climate regions and different building types.
Building energy performance is evaluated under three scenarios with reference building and building with ∼100% window-to-wall ratio (building with ∼100% WWR). The building scenario with a window-to- wall ratio of ∼100% is designed with a glass curtain wall system. This scenario is named a ‘building with ∼100% WWR’, taking into account that there are columns close to the glass curtain wall on the perimeter of the building. Three scenarios are neutral position, using a shading device on the building (position with shading device), and using a shading device integrated with the PV system on the building (position with BIPV), respectively. The position with the shading device scenario is the scenario that uses the shading device in the designed BIPV module dimensions. Building scenarios are shown in .
Table 1. The building scenarios.
Softwares accepted in the field (PVsyst and DesignBuilder-EnergyPlus) (Pabasara et al. Citation2019) are used for the implementation of the methodology. Studies were examined to show the accuracy of the results of the PV software used. In these studies, energy generation measurements and simulation results of the PV installations are compared. These validation studies are given in with PV type, measurement period, location and accuracy parameters.
Table 2. The studies on the accuracy of the PV software used.
These studies, which show the differences between the PVsyst software simulation results and the measurements, have been made in different PV types, in various locations, and in measurement periods of up to 12 years. While most are less than 5.0%, their accuracy ranges from 9% to 0.32%. More importantly, in studies with CIS technology, this difference is between 1.2% and 0.47%, so simulation results and measurements are quite close.
2.1. Definition of climate region
Two different cities are chosen in this study to evaluate how the BIPV system effectiveness may change according to the different climate regions. These cities are İstanbul (41° north latitude – 28.8° longittude) and Antalya (36.8° north latitude – 30.7° longitude) in Türkiye. The climate is warm-humid in İstanbul and hot-humid in Antalya. Also, these locations are in a Temperate-Dry summer-Hot summer (Csa) climate according to the Koppen climate classification. Solar radiation annual averages were 1168 kWh/m2 for İstanbul and 1390 kWh/m2 for Antalya (Özkiliç Keleş Citation2008). The average highest temperature in İstanbul is 26.9°C (Aug), the average lowest temperature is 3.1°C (Feb) and the average temperature is 14.4°C (Annual). The average highest temperature in Antalya is 34.1°C (Jul), the average lowest temperature is 6.0°C (Jan) and the average temperature is 18.8°C (Annual) (Turkish State Meteorological Service Citation2019). İstanbul and Antalya global radiation values (Republic of Turkey Ministry of Energy and Natural Resources Citation2023) by month are shown in .
Table 3. The city’s global radiation values (KWh/m2-day) by months (Republic of Turkey Ministry of Energy and Natural Resources Citation2023).
2.2. Case study building
Within the scope of this study, the building type is accepted as an office building considering the working hours of the day. Both a ∼100% WWR office building and a climate-adapted building (determined as a reference building) have been analysed.
It is assumed that the office building is detached and there is no shadow effect of surroundings. To constitute climatic design, building shape ratios presented by Olgyay for 25°–45° north latitude climate regions are adopted (Olgyay Citation1963; Yaşa Citation2013). In İstanbul (range 1/1–2.4 and best 1/1.6) and in Antalya (range 1/1–3 and best 1/1.7), a 1/1.7 aspect ratio which was within recommended range is adopted. To facilitate the comparison of climate scenarios, buildings in İstanbul and Antalya are designed with the same aspect ratio. In addition, reference building design has been made by considering the building bylaw requirements (IBB Citation2007) and TS 825 thermal insulation requirements for buildings (TSI Citation2008). The open-planned office building is designed. For climatic design, the building is located in the east–west direction, with the long edge of the building facing south. Dimensions of the reference building are 15.5 m in width, 26.4 m in length, and 3.6 m in storey height. The building consists of four identical storeys. The gross area of the building is 1636 m2, and the gross volume is 5892 m3.
In reference building, WWR for the north facade is determined according to at least 20% WWR for daylight utilisation in different studies. 50% WWR in the south facade, and 30% WWR in the east and west facades are adopted. Normal floor plans and elevations are given in . Window distribution design on facades is made compliant with interior furnishing depending on window-to-wall ratios. This way, the comfort and efficiency of daylight are increased.
The U values of building envelope constructions are determined according to U value upper limits suggested by TS 825 thermal insulation requirements for building regulation degree-day region (TSI Citation2008). The U values of the building envelope constructions of the buildings located in different regulation degree-day regions are indicated in . Other building constructions are used with default U values. The U values of the internal floor and partition construction of the buildings are 2.93 and 1.64 W/m2K, respectively. Double glass is used in windows. The solar heat gain coefficient (SHGC) of glazing is 0.802 and light transmission is 0.818.
Table 4. The U values of building envelope constructions in İstanbul and Ankara.
In the energy model, the shading devices are modelled with 100% opaque material and their thermal and reflective properties are not considered.
Activity variables that should be defined in the simulation programme and HVAC (Heating, Ventilation, and Cooling) system variables are given in . The variables indicated in belong to open-office space, and variable selections for other spaces are different.
Table 5. The Activity and HVAC system variable selections in the building simulation.
2.3. The design of fixed shading device integrated with PV system module
Due to the shading function of the BIPVshading, the type is selected according to the directions and the BIPVshading is designed as horizontal on the south side and vertically on the east and west sides (Dino Citation2017).
Horizontal BIPVshading module design:
In this article, additional studies that determine the optimum PV angle were examined. SOLAREC (European Union solar power electricity operation) research centre data and the annual tilt angle according to a different study in the literature is between 29 ° and 33° for Turkey’s cities (Kisa Ovali Citation2009; Moral Uğur Citation2006). The annual performance difference between 10° and 30° in Turkey’s conditions will not exceed 15%. In Turkey, considering the summer and winter seasonal average, the optimum PV tilt angle is acceptable as 30° (Özkiliç Keleş Citation2008; Yanardağ Citation2015; Turhan and Çetiner Citation2012). As a result, an optimum PV tilt angle of the scope of this study throughout the year in Turkey (36°–42° northern latitudes) is accepted 30°.
To determine the desired period of sun protection, the outdoor temperature curves of the relevant city are used (Berköz et al. Citation1995). To define the BIPV module length required for protection from the sun, the design day when the solar radiation comes the most oblique in the period for which protection is desired is determined. The design hour, on the other hand, is noon local time, when the directional solar radiation is highest in the horizontal plane.
The width of the BIPV is limited by the window widths (1.5 m) (Fouad, Mohamed, and Shihata Citation2018). The length of the BIPV is found by intersecting the optimum PV tilt angle with the direction of the solar radiation at the elevation angle at the determined day and hour ().
Since the PV size is considered as the active area (real area) in the 3D model of the PV software used and this study evaluates the area calculation, a PV module size is assumed to be 0.75 m × 0.56 m. As an example in , the PV module placement diagram on the horizontal BIPV module in the reference building in İstanbul is shown. In the BIPV system it is assumed that the areas outside the PV modules are completed with a different material.
In , the horizontal BIPV system design information in the building scenarios is shown.
Table 6. The horizontal BIPV system design information designed for building scenarios.
Vertical BIPVshading module design:
The time ranges from morning to noon on the eastern facade (8:30–12:00) and from noon to afternoon on the west facade (12:00–18:00), are the periods for which sun protection is desired. By studying the shadow curve diagram, the sun protection effect ranges of different BIPV system designs are examined and the vertical BIPVshading module layout in is considered. The diagram in shows the width of the BIPV modules, the angle between the facade and the distance parameters between each other. The height (h) of the vertical BIPV is limited by the height of the glass surface.
In this study, the disadvantages of shading are reduced by using CIS technology, which is less affected by shadows (Solar Frontier Citation2021). The vertical BIPV system is designed with the same PV module described in the horizontal BIPV system design. As an example in , vertical BIPV system design and module placement diagrams are shown in the reference building in İstanbul.
In , the vertical BIPV system design information in the building scenarios is shown. Model perspectives of the three scenarios are presented in .
Figure 5. Perspectives of three scenarios from the İstanbul reference building model (neutral position, position with shading device and position with BIPV).

Table 7. The vertical BIPV system design information designed for building scenarios.
2.4. Determining the energy needs and energy production
Building energy needs have been modelled using the dynamic thermal simulation software DesignBuilder, which is actually an interface programme using the EnergyPlus simulation engine. An annual energy analysis of the building scenarios in both İstanbul and Antalya is conducted. Net energy and energy consumption per unit area of conditioned building area are used in the evaluation of the energy analyses. Net energy indicates energy amount from grid after subtracting generated energy amount from consumed energy amount of the building.
PV energy generations in this study are calculated with the PVsyst software package. The building model with BIPV in the dynamic thermal simulation software is also created in the PV programme. The PV systems on the east, south and west facades are designed separately and for this reason, a PV system is formed on each of the 3 facades. PVsyst models of the scenarios with BIPV in Istanbul are shown in .
Figure 6. PVsyst models of the scenarios with BIPV in Istanbul (the reference building on the left and the building with ∼100% WWR on the right).

The used CIS thin film (cells) technology advantages are good low-light behaviour, high shadow tolerance, high-temperature stability and light soaking effect (Solar Frontier Citation2021). It can generate electricity not only in sunny weather but also in diffused light (Raf Citation2021). The nominal conversion efficiency of the modules id equal to 11% for CIS, which is a typical value (Raugei, Bargigli, and Ulgiati Citation2007).
It is considered that BIPV modules shade their own PV modules, in other words, self-shading. To account for the shadows of objects (building and BIPV) in the PV programme, a model of the PV installation and its surroundings is created and the linear shading calculation method is used. In thin-film modules, there is no electrical mismatch loss if the shades affect all cells of each module in the same way. Thus the ‘Linear shadings’ are representative for calculating shading losses in thin film modules. PVsyst uses a PV model that is very compatible with CIS (PVsyst Citation2021). The simulation is based on an hourly data file.
The specifications of the PV system and simulation parameters are shown in .
Table 8. PV system specifications and simulation parameters.
The energy cover factor (CPV) is used to determine the ratio of the amount of energy produced by the PV system to meet the electrical energy consumption of the building. CPV can be calculated with the equation given below (Cellura et al. Citation2012).
(1)
(1) EPV is the annual energy amount (kWh/y) generated by the PV system and ET,e is the annual electrical energy consumption of the building (kWh/y).
2.5. Calculation of the PV system energy performance indicators
Considering the whole life cycle of the PV system, energy is also consumed for the production of system components. To evaluate the energy performance of PV systems, life cycle energy analyses related to manufacturing and operation phases are made.
Energy input (Ein) is the total amount of energy needed during the PV system life cycle. The amount of energy (embodied energy values of PV systems) consumed during the production of PV modules and other system components is taken into account as energy input (Nawaz and Tiwari Citation2006; IEA Citation2006). Within the scope of other system components (BOS), supporting substructure, inverter and cabling are discussed. The gross energy requirement (total primary energy) of a CIS PV module is 2870 MJ/m2 (with frame) (Alsema Citation2012; Knapp and Jester Citation2001) and that of BOS is 700 MJ/m2 (Frankl et al. Citation1998). Energy output (Eout) is the amount of energy generated annually by the PV system. Eout is calculated with PVsyst software.
Energy payback time (EPBT) is the ratio of the total energy consumed during the PV system life cycle (Ein) to the annual energy amount generated during the operation phase (Eout) (IEA Citation2006). The energy return factor (ERF) is the ratio of the amount of energy generated during the PV system operation phase (Eout) to the total amount of energy consumed during the life cycle (Ein) (IEA Citation2006). EPBT (Alsema Citation2012, Citation1998) and ERF (Alsema Citation1998) are calculated with the following equations:
(2)
(2)
(3)
(3)
Within the scope of the study, the lifetime of the PV system (N) is taken into account as 30 years (Knapp and Jester Citation2001; IEA Citation2020). A linear degradation of 0.7%-points per year is assumed in the performance of PV modules over their lifetime (IEA Citation2020). In this study, the grid efficiency value (31%) determined for the Continental European electricity grid is used (IEA Citation2006).
The Performance Ratio (PR) is the ratio of the energy effectively produced, for the energy produced if the system was continuously working at its nominal STC efficiency. The PR is defined in the norm IEC EN 61724 and the PR formula is shown below for a grid-connected system: (PVsyst Citation2021)
(4)
(4) E_Grid is the available energy and PnomPV is the STC-installed power. The PR is used as a performance metric to quantify the overall system losses due to temperature effects, shading and inefficiency of its components (IEA Citation2020).
3. Results
Energy analysis results of the building scenarios for İstanbul and Antalya are given in and , respectively.
İstanbul and Antalya energy results are explained in terms of ‘Reference building’ and ‘Building with ∼100% WWR’. In addition, some energy ratio results are obtained by comparing the scenarios. The details are shared below.
As shown in , using BIPVshading on a reference building in İstanbul, the grid-based net energy consumption of the building decreased 24.82% compared to the neutral state of the building and 11.09% of this ratio is generated with PV system function. In the scenario where only shading devices are used, it can be seen that a 13.73% decrease in net energy consumption is achieved compared to the neutral state.
As shown in , by using BIPVshading on the reference building in Antalya, the grid-based net energy consumption of the building decreased by 35.21% compared to the neutral state of the building, 18.93% of this ratio is generated with shading function, and 16.28% is generated with PV system function.
As shown in , using BIPVshading on a building with ∼100% WWR in İstanbul, the grid-based net energy consumption of the building decreased by 41.05% compared to the neutral state of the building. In the scenario where only shading devices are used, it can be seen that a 27.09% decrease in net energy consumption is achieved compared to the neutral state. When the positions with the shading device and the position with BIPVshading are compared, it is seen that the PV system function decreased the energy consumption of the building by 13.96%.
As shown in , using BIPVshading on a building with ∼100% WWR in Antalya, the grid-based net energy consumption of the building decreased by 56.18% compared to the neutral state of the building, 36.12% of this ratio is generated with shading function, and 20.06% is generated with PV system function.
The performance Ratio is given separately (Eranki and Mani Citation2020) according to the facades of each building scenario. In this way, performance ratios of PV systems can be evaluated separately according to BIPVshading type (horizontal, vertical) and orientation.
The electrical energy consumption of the building, considered in the CPV calculation, consists of cooling, lighting and interior equipment (other) energy needs. The calculation results of the energy indicators and energy cover factor of PV systems are given in .
Table 9. The calculation results of the energy indicators and CPV of PV systems.
4. Discussion
The following inferences were made by comparing building scenarios in terms of climate regions and building envelopes.
When the neutral state of building scenarios in Antalya is compared with the same scenarios in İstanbul, it is seen that the net energy load is higher in Antalya. This is principally due to the higher cooling loads in Antalya than in Istanbul. Net energy loads of BIPV and shading device scenarios in Antalya decreased more than those in Istanbul. One of the reasons for such results is that more electrical energy is produced by the PV system due to the higher sunshine hours and solar radiation values in the hot climate region. In addition, another factor is larger PV areas in BIPV design in Antalya. One more reason is in hot climate regions with a higher cooling load, the shading function has a higher energy consumption decrease. As a result of the comparison of the places in these different latitudes and different climate regions, it has been seen that BIPVshading efficiency decreases from hot climate regions to warm climate regions.
The energy consumption difference between buildings with ∼100% WWR and reference buildings in a neutral state in both cities is dominantly caused by the cooling load. Since the building with ∼100% WWR had higher daylight usage, the lighting load is lower than the reference building. According to the energy analysis of the building scenarios, the share of PV function in the total reduction rate in the grid-based net energy consumption of the building is high in the reference building.
When BIPV is evaluated separately according to system functions, the following results are as follows:
While BIPV system shading function decreased the cooling load in both the reference building and ∼100% WWR building, the PV system function generated energy and decreased fossil fuel-based grid energy consumption of the building. BIPVshading reduces the benefit from sunlight and increases lighting load at certain levels. Additionally, during the heating period, BIPVshading decreases solar heat gain and increases building heating load in some circumstances. When a decrease in cooling load and energy generation by BIPVshading are considered, such an increase in heating load is insignificant. A decrease in building energy load is generated by the shading function during the cooling period of BIPVshading. A decrease in net grid-based energy consumption is the total gain of shading and energy production functions. The shading function affecting cooling load is more effective on (∼100% WWR) buildings that had a higher cooling load. Also, the bigger size of the BIPVshading in ∼100% WWR building is effective on this result.
The shading function had a higher contribution to the total gain provided by BIPVshading. Given the low PV yields according to the current PV technology, the BIPVshading gain will increase as the efficiency of PV technologies increases.
The study is evaluated in terms of BIPV energy gain and the following results are obtained:
In , the energy gain of BIPVshading and only the energy production of the PV function are presented for the unit area. PV energy production indicates the energy amount produced by only the PV system. BIPVshading energy gain (Sun, Lu, and Yang Citation2012) represents energy gain due to decreased cooling load caused by the shading effect of the system and PVs’ energy production. In short, this gain value is the total decrease value provided by the net energy load of the building in the neutral state. Information regarded PV energy production and BIPVshading energy gain are average of all PV or BIPVshading on the east, south, and west facade.
Table 10. PV energy production and BIPVshading energy gain based on building scenario.
A higher energy gain of 1 m2 BIPVshading in a building with ∼100% WWR compared to a reference building is caused by the fact that the shading effect is more effective since the cooling load is higher in a building with ∼100% WWR. However, the ratio of the energy gain from 1 m2 BIPVshading to the total energy load of the building in the neutral state should be considered. This reduction rate is lower in the ∼100% WWR building than in the reference building because the energy load in the ∼100% WWR building is higher.
The rate (ratio) which provides building energy needs with energy gain obtained from 1 m2 BIPVshading is higher in a reference building adapted to climate compared to ∼100% WWR building. It is seen that BIPVshading is more effective in climatic building design (in terms of affecting the building total net energy load). Accordingly, it can be suggested that buildings should be designed and adapted to climate and then equipped with active systems such as PV (BIPV).
The energy cover factor is between 18.1% and 34.6% in scenarios and is a significant share in meeting the electricity needs of buildings.
The PV part of the BIPV system is evaluated with the PV system energy performance indicators.
The EPBT values of the building scenarios are calculated between 3 and 4.9 years. The PV system pays back the energy spent in its manufacturing phase within 30 years of system life. In both cities, the EPBT is longer at ∼100% WWR building compared to the reference building.
It is determined that the PV systems can produce 6.5 to 9.1 (ERF values) times the amount of energy consumed during the manufacturing stages over a 30-year usage period. The ERF value in Antalya is more than the ERF value in İstanbul.
PR is lower in ∼100% WWR building scenarios compared to reference building scenarios. When the same scenarios of İstanbul and Antalya are compared, it is seen that the PR on the south facades of Antalya are less than İstanbul. The PV performance ratios on the east facades (and between the west facades) of İstanbul and Antalya building scenarios in the same building envelope are the same. There is a strong temperature dependence on the PR (Reich et al. Citation2012). Performance ratios lower than 66% may be due to temperature effects and shading.
5. Conclusions
In this paper, the BIPVshading design approach is presented and applied in an office building example in different climates and different building envelopes. Furthermore, it focuses on ‘the impact of BIPVshading on building net energy needs’, ‘PV system life cycle’ and ‘CIS type PV use’. It is a study that has results in line with directly and indirectly many of the United Nations Sustainable Development Goals. Detailed results of the study are listed below.
A unique BIPVshading design approach represented with cases that can be used by BIPVshading designers is presented. The effect of BIPVshading on building energy use is exemplified by different scenarios. In this way, the BIPVshading energy gains that will be most beneficial in the architectural design have been explored.
The study suggests that CIS PV panels can function as shading devices, not only on the roof but also on glazed facades, serving a dual function: generating electricity and shading fenestration. In this way, the building’s surface area where photovoltaics can be used increases.
One of the contributions of this study is to show how effective the PV and shading functions of the BIPVshading system are in different climates and different building envelopes.
In terms of reducing the building energy load, CIS BIPVshading is effective mainly in sunny and warm (hot) climates.
To increase the effect of (per unit area) BIPVshading on the role of reducing the building energy load, it has been observed that the energy load must be reduced by building design. This study leads how BIPVshading can be dealt with in the building design process.
The ratio of 1 m2 BIPVshading energy gain to building energy requirement is a concept presented in this study. This concept, described in the context of the study, shows the extent to which the BIPVshading can be effective in reducing the building's energy load. Also, BIPVshading is the way of displaying the function of PV in a building in subscript format and is offered here.
A multi-faceted BIPV energy evaluation approach that covers the effect of the BIPV system on the building energy need, the effect on the grid energy demand and the PV system life cycle energy analysis is created.
With the energy gain of the BIPV system, the grid electricity demand is reduced. In addition, since the CIS PV system produces more than the energy input, CO2 emissions can also be reduced.
With the effect of the shade tolerance feature of CIS technology, the case study showed successful energy performance, despite the self-shading of BIPVshadings. The shade tolerance feature of CIS technology, and colour homogeneity feature, bring CIS-type PV to the fore by providing flexibility in terms of the freedom of architectural design that integrates with the PV. Most importantly, all this contributes to sustainable architecture by reducing the integrated design difficulties in front of BIPV.
As a result, the use of CIS BIPVshading in the sample office building can sustainably integrate the renewable energy system (PV) into the building with successful energy performance, in addition to providing energy gain in all the scenarios discussed.
In future studies, the BIPVshading design approach can be improved in terms of glare and daylight illuminance level.
Disclosure statement
No potential conflict of interest was reported by the author(s).
References
- Aaditya, G., and M. Mani. 2018. “BIPV: A Real-Time Building Performance Study for a Roof-Integrated Facility.” International Journal of Sustainable Energy 37 (3): 249–267. doi:10.1080/14786451.2016.1261864.
- Ahmad, R. M., and R. M. Reffat. 2018. “A Comparative Study of Various Daylighting Systems in Office Buildings for Improving Energy Efficiency in Egypt.” Journal of Building Engineering 18: 360–376. doi:10.1016/j.jobe.2018.04.002.
- Akbari Paydar, M. 2020. “Optimum Design of Building Integrated PV Module as a Movable Shading Device.” Sustainable Cities and Society 62: 102368. doi:10.1016/j.scs.2020.102368.
- Alsema, E. 1998. “Energy Requirements of Thin-Film Solar Cell Modules – A Review.” Renewable and Sustainable Energy Reviews 2: 387–415. doi:10.1016/S1364-0321(98)00019-7.
- Alsema, E. 2012. “Chapter IV-2 – Energy Payback Time and CO2 Emissions of PV Systems.” In Practical Handbook of Photovoltaics (Second Edition), Fundamentals and Applications, edited by Augustin McEvoy, Tom Markvart, and Luis Castañer, 1097–1117. Waltham, MA, USA: Academic Press.
- Badescu, V., and F. Iacobescu. 2013. “Simple Technological Guidelines for the Implementation of the Romanian National Strategy on PV Systems.” Energy for Sustainable Development 17 (3): 220–227. doi:10.1016/j.esd.2012.12.003.
- Berköz, E., Z. Y. Aygün, G. Kocaaslan, E. Yildiz, F. Ak, M. Küçükdoğu, D. Enarun, R. Ünver, A. K. Yener, and D. Yildiz. 1995. Energy Effective Housing and Settlement Design. [In Turkish.] TÜBİTAK İNTAG Project No: 201, İstanbul.
- Biyik, E., M. Araz, A. Hepbasli, M. Shahrestani, R. Yao, L. Shao, E. Essah, et al. 2017. “A Key Review of Building Integrated Photovoltaic (BIPV) Systems.” Engineering Science and Technology, an International Journal 20 (3): 833–858. doi:10.1016/j.jestch.2017.01.009.
- Bot, K., N. M. M. Ramos, R. M. S. F. Almeida, P. F. Pereira, and C. Monteiro. 2019. “Energy Performance of Buildings with On-site Energy Generation and Storage – An Integrated Assessment Using Dynamic Simulation.” Journal of Building Engineering 24: 100769. doi:10.1016/j.jobe.2019.100769.
- Braun, P., and R. Rüther. 2010. “The Role of Grid-Connected, Building-Integrated Photovoltaic Generation in Commercial Building Energy and Power Loads in a Warm and Sunny Climate.” Energy Conversion and Management 51: 2457–2466. doi:10.1016/j.enconman.2010.04.013.
- Cellura, M., A. Di Gangi, S. Longo, and A. Orioli. 2012. “Photovoltaic Electricity Scenario Analysis in Urban Contests: An Italian Case Study.” Renewable and Sustainable Energy Reviews 16: 2041–2052. doi:10.1016/j.rser.2012.01.032.
- Chang, Y. 2010. “Optimal the Tilt Angles for Photovoltaic Modules in Taiwan.” Electrical Power and Energy Systems 32: 956–964. doi:10.1016/j.ijepes.2010.02.010.
- Chepp, E. D., F. P. Gasparin, and A. Krenzinger. 2021. “Accuracy Investigation in the Modeling of Partially Shaded Photovoltaic Systems.” Solar Energy 223: 182–192. doi:10.1016/j.solener.2021.05.061.
- Costanzo, V., R. Yao, E. Essah, L. Shao, M. Shahrestani, A. C. Oliveira, M. Araz, A. Hepbasli, and E. Biyik. 2018. “A Method of Strategic Evaluation of Energy Performance of Building Integrated Photovoltaic in the Urban Context.” Journal of Cleaner Production 184: 82–91. doi:10.1016/j.jclepro.2018.02.139.
- Custódio, I., T. Quevedo, A. P. Melo, and R. Rüther. 2022. “A Holistic Approach for Assessing Architectural Integration Quality of Solar Photovoltaic Rooftops and Shading Devices.” Solar Energy 237: 432–446. doi:10.1016/j.solener.2022.02.019.
- Dehwah, A. H. A., and M. Asif. 2019. “Assessment of Net Energy Contribution to Buildings by Rooftop Photovoltaic Systems in hot-Humid Climates.” Renewable Energy 131: 1288–1299. doi:10.1016/j.renene.2018.08.031.
- Devetaković, M., D. Djordjević, M. Radojević, A. Krstić-Furundžić, B.-G. Burduhos, G. Martinopoulos, M. Neagoe, and G. Lobaccaro. 2020. “Photovoltaics on Landmark Buildings with Distinctive Geometries.” Applied Sciences 10: 6696. doi:10.3390/app10196696.
- Dino, I. G. 2017. “Evolutionary Optimization of Building Envelope Design with Photovoltaics-Integrated Shading Devices.” LC3 2017: volume 1-proceedings of the joint conference on computing in construction (JC3) July 4−7, 2017, Heraklion, Greece, 457−464.
- Dino, I. G. 2017. “The Performance Evaluation of Solar Control Methods in Buildings: A Multi-Objective Approach.” [In Turkish.] GU Journal of Science Part C 5 (3): 71–87.
- Ekoe A Akata, A. M., D. Njomo, and B. Agrawal. 2017. “Assessment of Building Integrated Photovoltaic (BIPV) for Sustainable Energy Performance in Tropical Regions of Cameroon.” Renewable and Sustainable Energy Reviews 80: 1138–1152. doi:10.1016/j.rser.2017.05.155.
- Elzeyadi, I. 2017. “The Impacts of Dynamic Façade Shading Typologies on Building Energy Performance and Occupant’s Multi-Comfort.” Architectural Science Review 60 (4): 316–324. doi:10.1080/00038628.2017.1337558.
- Eranki, G. A., and M. Mani. 2020. “Integrability Assessment Methodology for Building Integrated Photovoltaics: Concept and Application.” International Journal of Sustainable Energy 39 (4): 362–379. doi:10.1080/14786451.2019.1699559.
- Fouad, M. M., A. G. ElSayed, L. A. Shihata, H. A. Kandil, and E. I. Morgan. 2019. “Life Cycle Assessment for Photovoltaic Integrated Shading System with Different End of Life Phases.” International Journal of Sustainable Energy 38 (9): 821–830. doi:10.1080/14786451.2019.1588272.
- Fouad, M. M., A. H. Mohamed, and L. A. Shihata. 2018. “Modeling and Analysis of Building Attached Photovoltaic Integrated Shading Systems (BAPVIS) Aiming for Zero Energy Buildings in hot Regions.” Journal of Building Engineering 21: 18–27. doi:10.1016/j.jobe.2018.09.017.
- Frankl, P., A. Masini, M. Gamberale, and D. Toccaceli. 1998. “Simplified Life-Cycle Analysis of PV Systems in Buildings—Present Situation and Future Trends.” Progress in Photovoltaics: Research and Applications 6 (2): 137–146. doi:10.1002/(SICI)1099-159X(199803/04)6:2<137::AID-PIP208>3.0.CO;2-N.
- Frydrychowicz–Jastrzębska, G., and A. Bugała. 2016. “CIS, CIGS and CIBS Thin Film Solar Cells and Possibilities of Their Application in BIPV.” Computer Applications in Electrical Engineering 14: 364–377. doi:10.21008/j.1508-4248.2016.0031.
- González-Peña, D., I. García-Ruiz, M. Díez-Mediavilla, M. I. Dieste-Velasco, and C. Alonso-Tristán. 2021. “Photovoltaic Prediction Software: Evaluation with Real Data from Northern Spain.” Applied Sciences 11: 5025. doi:10.3390/app11115025.
- Grobman, Y. J., I. G. Capeluto, and G. Austern. 2016. “External Shading in Buildings: Comparative Analysis of Daylighting Performance in Static and Kinetic Operation Scenarios.” Architectural Science Review 60 (2): 126–136. doi:10.1080/00038628.2016.1266991.
- Hagemann, I. B. 2004. “Examples of Successful Architectural Integration of PV: Germany.” Progress in Photovoltaics: Research and Applications 12: 461–470. doi:10.1002/pip.561.
- Huang, Y., J. Niu, and T. Chung. 2011. “Energy and Carbon Emission Payback Analysis for Energy-Efficient Retrofitting in Buildings—Overhang Shading Option.” Energy and Buildings 44: 94–103. doi:10.1016/j.enbuild.2011.10.027.
- Hwang, T., S. Kang, and J. T. Kim. 2011. “Optimization of the Building Integrated Photovoltaic System in Office Buildings-Focus on the Orientation, Inclined Angle and Installed Area.” Energy and Buildings 46: 92–104. doi:10.1016/j.enbuild.2011.10.041.
- IBB (İstanbul Metropolitan Municipality). 2007. Istanbul Building Bylaws:1512. [In Turkish.] İstanbul: İstanbul Metropolitan Municipality.
- IEA. 2006. Compared Assessment of Selected Environmental Indicators of Photovoltaic Electricity in OECD Cities, Report IEA-PVPS T10-01:2006, International Energy Agency, Photovoltaic power systems programme.
- IEA. 2020. Task 12 PV Sustainability – Methodology Guidelines on Life Cycle Assessment of Photovoltaic, Report IEA-PVPS T12-18:2020, International Energy Agency, Photovoltaic Power Systems Programme, 4th edition.
- Jayathissa, P., M. Luzzatto, J. Schmidli, J. Hofer, Z. Nagy, and A. Schlueter. 2017. “Optimising Building Net Energy Demand with Dynamic BIPV Shading.” Applied Energy 202: 726–735. doi:10.1016/j.apenergy.2017.05.083.
- Jung, S. K. 2014. “Optimal Control Methods for PV-Integrated Shading Devices.” PhD diss., University of Michigan.
- Kalogirou, S. A., R. Agathokleous, and G. Panayiotou. 2013. “On-site PV Characterization and the Effect of Soiling on Their Performance.” Energy 51: 439–446. doi:10.1016/j.energy.2012.12.018.
- Kim, J., S. K. Jung, Y. S. Choi, and J. T. Kim. 2010. “Optimization of Photovoltaic Integrated Shading Devices.” Indoor and Built Environment 19 (1): 114–122. doi:10.1177/1420326X09358139.
- Kisa Ovali, P. 2009. “Forming Ecological Design Criteria Systematics in Terms of Climate Regions in Turkey: Kayaköy Settlement Sample.” [In Turkish.] PhD diss., Trakya University.
- Knapp, K., and T. Jester. 2001. “Empirical Investigation of the Energy Payback Time for Photovoltaic Modules.” Solar Energy 71 (3): 165–172. doi:10.1016/S0038-092X(01)00033-0.
- Lovati, M., G. Salvalai, G. Fratus, L. Maturi, R. Albatici, and D. Moser. 2019. “New Method for the Early Design of BIPV with Electric Storage: A Case Study in Northern Italy.” Sustainable Cities and Society 48: 101400. doi:10.1016/j.scs.2018.12.028.
- Maghrabie, H. M., M. A. Abdelkareem, A. H. Al-Alami, M. Ramadan, E. Mushtaha, T. Wilberforce, and A. G. Olabi. 2021. “State-of-the-Art Technologies for Building-Integrated Photovoltaic Systems.” Buildings 11: 383. doi:10.3390/buildings11090383.
- Malvoni, M., A. Leggieri, G. Maggiotto, P. M. Congedo, and M. G. De Giorgi. 2017. “Long Term Performance, Losses and Efficiency Analysis of a 960 kWP Photovoltaic System in the Mediterranean Climate.” Energy Conversion and Management 145: 169–181. doi:10.1016/j.enconman.2017.04.075.
- Mandalaki, M., T. Tsoutsos, and N. Papamanolis. 2014. “Integrated PV in Shading Systems for Mediterranean Countries: Balance Between Energy Production and Visual Comfort.” Energy and Buildings 77: 445–456. doi:10.1016/j.enbuild.2014.03.046.
- Mandalaki, M., K. Zervas, T. Tsoutsos, and A. Vazakas. 2012. “Assessment of Fixed Shading Devices with Integrated PV for Efficient Energy Use.” Solar Energy 86: 2561–2575. doi:10.1016/j.solener.2012.05.026.
- Martinopoulos, G., A. Serasidou, P. Antoniadou, and A. M. Papadopoulos. 2018. “Building Integrated Shading and Building Applied Photovoltaic System Assessment in the Energy Performance and Thermal Comfort of Office Buildings.” Sustainability 10: 4670. doi:10.3390/su10124670.
- Mehleri, E. D., P. L. Zervas, H. Sarimveis, J. A. Palyvos, and N. C. Markatos. 2010. “Determination of the Optimal Tilt Angle and Orientation for Solar Photovoltaic Arrays.” Renewable Energy 35: 2468–2475. doi:10.1016/j.renene.2010.03.006.
- Mermoud, A., and T. Lejeune. 2010. “Performance Assessment of a Simulation Model for PV Modules of Any Available Technology.” 25th European photovoltaic solar energy conference, 6-10 September, Valencia, Spain.
- Moral Uğur, E. 2006. “A Study About the Integration of Photovoltaics with Building Envelope System Elements.” [In Turkish.] Master's thesis, ITU Graduate School Of Science, Engineering and Technology, İstanbul.
- Nawaz, I., and G. N. Tiwari. 2006. “Embodied Energy Analysis of Photovoltaic (PV) System Based on Macro- and Micro-Level.” Energy Policy 34: 3144–3152. doi:10.1016/j.enpol.2005.06.018.
- Nicolás-Martín, C., P. Eleftheriadis, and D. Santos-Martín. 2020. “Validation and Self-Shading Enhancement for SoL: A Photovoltaic Estimation Model.” Solar Energy 202: 386–408. doi:10.1016/j.solener.2020.03.099.
- Olgyay, V. 1963. Design with Climates. Princeton, NJ: Princeton University Press.
- Özkiliç Keleş, C. 2008. “A Study About Photovoltaic Systems in Point of Energy Efficiency on the Buildings in Turkey.” [In Turkish.] Master's thesis, ITU Graduate School of Science, Engineering and Technology, İstanbul.
- Pabasara, W. M., U. Wijeratne, R. J. Yang, E. Too, and R. Wakefield. 2019. “Design and Development of Distributed Solar PV Systems: Do the Current Tools Work?” Sustainable Cities and Society 45: 553–578. doi:10.1016/j.scs.2018.11.035.
- Panteli, C., A. Kylili, L. Stasiuliene, L. Seduikyte, and P. A. Fokaides. 2018. “A Framework for Building Overhang Design Using Building Information Modeling and Life Cycle Assessment.” Journal of Building Engineering 20: 248–255. doi:10.1016/j.jobe.2018.07.022.
- Peng, C., L. Huang, J. Liu, and Y. Huang. 2015. “Design and Practical Application of an Innovative Net-Zero Energy House with Integrated Photovoltaics: A Case Study from Solar Decathlon China 2013.” Architectural Science Review 58 (2): 144–161. doi:10.1080/00038628.2015.1011075.
- Peng, C., Y. Huang, and Z. Wu. 2011. “Building-Integrated Photovoltaics (BIPV) in Architectural Design in China.” Energy and Buildings 43: 3592–3598. doi:10.1016/j.enbuild.2011.09.032.
- pv Europe. 2016. “AVANCIS Launches New CIS Module SKALA for Solar Façades – Scalable in Size and Color.” Accessed February 17, 2021. https://www.pveurope.eu/solar-modules/avancis-launches-new-cis-module-skala-solar-facades-scalable-size-and-color.
- PVsyst. 2021. SUPPORT (Help). Accessed February 17, 2021. https://www.pvsyst.com/support/.
- Raf. 2021. İltay Enerji – Würth Solar CIS Thin Film Photovoltaic Panels [In Turkish.] Accessed February 16, 2021. https://www.raf.com.tr/urun/iltay-enerji-wurth-solar-cis-ince-film-fotovoltaik-paneller/2813.
- Ramanan, P., K. Kalidasa Murugavel, and A. Karthick. 2019. “Performance Analysis and Energy Metrics of Grid-Connected Photovoltaic Systems.” Energy for Sustainable Development 52: 104–115. doi:10.1016/j.esd.2019.08.001.
- Raugei, M., S. Bargigli, and S. Ulgiati. 2007. “Life Cycle Assessment and Energy pay-Back Time of Advanced Photovoltaic Modules: CdTe and CIS Compared to Poly-Si.” Energy 32: 1310–1318. doi:10.1016/j.energy.2006.10.003.
- Reich, N. H., B. Mueller, A. Armbruster, W. G. J. H. M. Van Sark, K. Kiefer, and C. Reise. 2012. “Performance Ratio Revisited: Is PR > 90% Realistic?” Progress In Photovoltaics: Research And Applications 20: 717–726. doi:10.1002/pip.1219.
- Republic of Turkey Ministry of Energy and Natural Resources. 2023. General Directorate of Energy Affairs GEPA (potential atlas of solar energy). Accessed February 7, 2023. https://gepa.enerji.gov.tr/MyCalculator/.
- Shukla, A. K., K. Sudhakar, and P. Baredar. 2016. “A Comprehensive Review on Design of Building Integrated Photovoltaic System.” Energy and Buildings 128: 99–110. doi:10.1016/j.enbuild.2016.06.077.
- Solar Frontier. 2021. What is CIS?. Accessed February 16, 2021. https://www.solar-frontier.eu/en/cis-technology/what-is-cis/.
- Stamatakis, A., M. Mandalaki, and T. Tsoutsos. 2016. “Multi-Criteria Analysis for PV Integrated in Shading Devices for Mediterranean Region.” Energy and Buildings 117: 128–137. doi:10.1016/j.enbuild.2016.02.007.
- Sun, L., L. Lu, and H. Yang. 2012. “Optimum Design of Shading-Type Building-Integrated Photovoltaic Claddings with Different Surface Azimuth Angles.” Applied Energy 90: 233–240. doi:10.1016/j.apenergy.2011.01.062.
- Sun, L. L., and H. X. Yang. 2009. “Impacts of the Shading-Type Building-Integrated Photovoltaic Claddings on Electricity Generation and Cooling Load Component through Shaded Windows.” Energy and Buildings 42: 455–460. doi:10.1016/j.enbuild.2009.10.014.
- Taveres-Cachat, E., G. Lobaccaro, F. Goia, and G. Chaudhary. 2019. “A Methodology to Improve the Performance of PV Integrated Shading Devices Using Multi-Objective Optimization.” Applied Energy 247: 731–744. doi:10.1016/j.apenergy.2019.04.033.
- Touma, A. A., and D. Ouahrani. 2018. “The Selection of Brise Soleil Shading Optical Properties for Energy Conservation and Glare Removal: A Case Study in Qatar.” Journal of Building Engineering 20: 510–519. doi:10.1016/j.jobe.2018.08.020.
- TSI (Turkish Standards Institution). 2008. TS 825: Thermal Insulation Requirements for Buildings (amendment of 2009 revision). [In Turkish.] Ankara: Turkish Standards Institution.
- Turhan, S., and I. Çetiner. 2012. “Performance Evaluation in Photovoltaic Systems.” [In Turkish.] Paper presented at the 6th national roof and facade symposium Bursa, April 12–13.
- Turkish State Meteorological Service. 2019. Official Statistics. Accessed May 5, 2019. https://mgm.gov.tr/veridegerlendirme/il-ve-ilceler-istatistik.aspx?k=A.
- Vassiliades, C., A. Michael, A. Savvides, and S. Kalogirou. 2018. “Improvement of Passive Behaviour of Existing Buildings Through the Integration of Active Solar Energy Systems.” Energy 163: 1178–1192. doi:10.1016/j.energy.2018.08.148.
- Viduruwan, G., and D. K. A. Induranga. 2021. “Validation of Meteonorm 8 for Energy Estimation of Solar Power Plants in Sri Lanka, Using PVsyst Software.” 3rd International conference on electrical engineering, 24 September, Colombo, Sri Lanka.
- Vincenzo, M. C., D. Kesten, and D. Infield. 2012. “Assessment of PV Shading Device on Building Energy Consumption Taking into Account Site Layout.” Journal of Energy and Power Engineering 6: 346–352.
- Wang, D., and Y. Chang. 2010. “Optimization of Tilt Angle for Photovoltaic Modules Based on the Neural-Genetic Algorithm.” Journal of Nan Kai 7 (1): 57–70.
- Wittkopf, S., S. Valliappan, L. Liu, K. S. Ang, and S. C. J. Cheng. 2012. “Analytical Performance Monitoring of a 142.5 kWp Grid-Connected Rooftop BIPV System in Singapore.” Renewable Energy 47: 9–20. doi:10.1016/j.renene.2012.03.034.
- Wittmer, B., A. Mermoud, and T. Schott. 2015. “Analysis of PV Grid Installations Performance, Comparing Measured Data to Simulation Results to Identify Problems in Operation and Monitoring.” 31st European photovoltaic solar energy conference, 14–18 September, Hamburg, Germany.
- Yanardağ, H. M. 2015. “Evaluation of Energy and Cost Performance of PV Systems on Different Building Forms.” [In Turkish.] Master's thesis, ITU Graduate School Of Science, Engineering And Technology, İstanbul.
- Yaşa, E. 2013. “Evaluation of Effect of Building Form for Different Climate Regions on Micro Climatic Comfort in Terms of Thermal Performance.” [In Turkish.] Paper presented at the environmental design congress Bursa, December 12–13.
- Yoo, S., and E. Lee. 2002. “Efficiency Characteristic of Building Integrated Photovoltaics as a Shading Device.” Building and Environment 37: 615–623. doi:10.1016/S0360-1323(01)00071-3.
- Yoo, S., E. Lee, and J. Lee. 1998. “Building Integrated Photovoltaics: A Korean Case Study.” Solar Energy 64 (4-6): 151–161. doi:10.1016/S0038-092X(98)00115-7.
- Zhang, X., S.-K. Lau, S. S. Y. Lau, and Y. Zhao. 2018. “Photovoltaic Integrated Shading Devices (PVSDs): A Review.” Solar Energy 170: 947–968. doi:10.1016/j.solener.2018.05.067.
- Zorer Gedik, G. 2001. “The Design of Shading Devices in Relation to the Window Orientations.” Paper presented at the livable environments and architecture international congress, Trabzon, July 4–7.
- Zorer Gedik, G. 2002. “An Approach to Designing an Optimal Shading Device.” Architectural Science Review 45 (4): 285–293. doi:10.1080/00038628.2002.9696942.