ABSTRACT
Introduction
Radiological/nuclear accidents, hostile military activity, or terrorist strikes have the potential to expose a large number of civilians and military personnel to high doses of radiation resulting in the development of acute radiation syndrome and delayed effects of exposure. Thus, there is an urgent need for sensitive and specific assays to assess the levels of radiation exposure to individuals. Such radiation exposures are expected to alter primary cellular proteomic processes, resulting in multifaceted biological responses.
Areas covered
This article covers the application of proteomics, a promising and fast developing technology based on quantitative and qualitative measurements of protein molecules for possible rapid measurement of radiation exposure levels. Recent advancements in high-resolution chromatography, mass spectrometry, high-throughput, and bioinformatics have resulted in comprehensive (relative quantitation) and precise (absolute quantitation) approaches for the discovery and accuracy of key protein biomarkers of radiation exposure. Such proteome biomarkers might prove useful for assessing radiation exposure levels as well as for extrapolating the pharmaceutical dose of countermeasures for humans based on efficacy data generated using animal models.
Expert opinion
The field of proteomics promises to be a valuable asset in evaluating levels of radiation exposure and characterizing radiation injury biomarkers.
1. Introduction
Radiological and nuclear injuries as a result of natural calamities, nuclear power plant safety failures/accidents, military conflicts, or terrorist attacks will no doubt need medical interventions [Citation1–3]. Under worst-case scenarios involving nuclear plant disasters and associated core meltdowns, there would no doubt be devastating consequences to human health and to the environment by way of large releases of radioactivity [Citation4,Citation5]. The events associated with the Fukushima Daiichi nuclear reactor disaster illustrate the health hazards posed by accidental radiation exposure [Citation6]. Full and proper radiological exposure management most certainly includes but is not limited to dose assessment and the identification of the individuals requiring medical intervention.
Acute radiation syndromes (ARS) manifest as hematopoietic (H-ARS), gastrointestinal (GI-ARS), and neurovascular syndromes (NV-ARS) after exposures to high doses of radiation delivered at relatively high dose rates. Clinical reports have documented that individuals exposed to the doses of 6–10 Gy will die generally within a few weeks due to GI-ARS. While lower doses of 2–6 Gy are not lethal immediately, they compromise the integrity of the hematopoietic system and if not treated, subject the individual to risk of death within the months following exposure [Citation7,Citation8]. However, some exposed individuals might manifest little signs of ARS (due to lower levels of radiation exposure or perhaps partial-body exposure) but later develop delayed-types of pathologies (e.g. organ system fibrosis, progressive kidney and cardiovascular diseases, cancer, etc.). Such late arising diseases have been documented in follow-up studies of survivors of the atomic bombing of Hiroshima and Nagasaki that ended World War II, as well as the survivors of the Chernobyl nuclear accident [Citation9–11]. These symptoms are known as late effects or delayed effects of acute radiation exposure (DEARE). Accordingly, improved analytics that allow for the identification, validation, and regulatory approval of biomarkers capable of accurately estimating the absorbed dose appears to be the forefront of the effort to better clinically manage individuals/victims of unwanted radiation exposure. Such biomarkers will be helpful for making medical decisions for the treatment of exposed individuals [Citation12,Citation13].
The current clinical strategy for assessing an individual’s radiation exposure is based on the observation of symptoms developed over time [Citation8]. These methods are time-consuming and not practical for real-time, large-scale exposure scenarios. Furthermore, the latent period is a critical time in which therapeutic intervention could help save lives. Therefore, there is an urgent and unmet need to develop biochemical or molecular biomarkers that could help to develop and to implement effective medical countermeasures (MCMs) for radiation injury in heavily exposed individuals, and thus, provide a basis for treatment decisions. An important consideration of pre-clinical biomarker development is that the appearance of clinical symptoms may lag behind the underlying pathophysiological changes. The understanding of exactly when an exposed individual transitions from having curable exposure symptoms to more refractive pathologies remains elusive. Having specific biomarkers of radiation exposure and related dose estimates would perhaps allow tissue-specific injuries to be prognosticated, thus allowing for more efficient triage and clinical interventions [Citation14,Citation15].
Over many decades, attempts have been made to estimate the an individual’s received radiation dose using hematological, biochemical, and cytogenetic parameters [Citation12]. Currently, lymphocyte depletion kinetics, clinical observation, and dicentric chromosome assays are used for post-exposure dose assessment. These assays are highly technical, time-consuming, and labor intensive. Thus, there is an urgent need to identify novel biomarkers that are not only robust and sensitive tofull range and conditions of radiation exposure, but also to employ techniques that are minimally invasive. A biomarker is an objective feature which can be measured and can indicate a pathological, biological, or therapeutic effect [Citation16]. It may exhibit biological processes related to the disease or a step downstream of the early effect. Biomarkers can be grouped into diagnostic markers to triage people and prognostic markers to be used as appropriate therapeutic options. A predictive biomarker may predict a favorable effect or indicate an adverse effect of a specific strategy. A composite biomarker is comprised of several molecules combined in a particular algorithm to achieve a single output. Biomarkers can serve as early detection surrogates for injuries or diseases and can have significant impact on diagnosis and therapy. Biomarkers should be easily detectable and quantifiable and provide important information. Another type of biomarker is a proteomics biomarker, which is a biomarker that is derived from the analysis of proteins or peptides in a biological sample, such as blood, urine, saliva, or tissue. The discipline of proteomics identifies, characterizes, and measures proteins expressed under a variety of conditions. Proteomics biomarkers can reflect the molecular mechanisms and pathways involved in a disease or a response and can provide information on the functional status and dynamics of the biological system. Since the majority of biological and disease processes manifest at the proteome level, proteomics complements to the approaches of genomics and transcriptomics. In brief, there is tremendous interest in proteomics to develop new biomarkers for diagnosis, prognosis, and prediction of diseases, and to accelerate drug development [Citation17,Citation18]. A radiation (injury) biomarker is a type of proteomics biomarker that can be used to assess the exposure, dose, effect, or susceptibility of an individual or a population to ionizing radiation. Radiation biomarkers can help to identify and stratify individuals who have been exposed to radiation, either accidentally or intentionally, and to guide the appropriate medical intervention and treatment. There is a necessity for biomarkers of total-body irradiation (TBI) and partial-body irradiation (PBI), which induce H-ARS and GI-ARS, and also markers of longer-term (delayed and late effects) radiation-induced lesions, such as fibrosis and necrosis [Citation12]. There are several reports of proteomes as radiation injury biomarkers [Citation19–21].
We limit the scope of our discussion to the results of proteome analysis in various animal models using acute lethal doses of different qualities of radiation exposures. Furthermore, emphasis has been given to recent studies using the latest high-throughput proteomic platforms for identifying and validating proteins as biomarkers for radiation injury and radiation MCM effects.
2. Techniques used in proteomic studies
A number of techniques are currently being applied to identify and validate proteomic entities/proteins that might serve as useful biomarkers for radiation injury () [Citation22]. Furthermore, many of these proteins are also being investigated in terms of their modulation by MCM treatment using unirradiated and irradiated animals. Such studies will be useful for converting MCM efficacious doses from animal models to humans for their regulatory approval following the US Food and Drug Administration (US FDA) Animal Rule.
2.1. Conventional proteomics
There are several conventional techniques used in proteomic analysis including gel electrophoresis, mass spectrometry (MS), two-dimensional gel electrophoresis, western blotting, protein microarrays, enzyme-linked immunosorbent assay (ELISA), Luminex, and isotope labeling [Citation23–27]. The latter techniques represent just a few examples of the conventional techniques currently used in proteomics, while many other methods are continually being developed and refined in order to improve our understanding of proteomes and their functions. These techniques are being used in the area of radiation biology for identifying and validating specific proteomes thought to be involved in the fundamental molecular biological processes of radiation injury development and expression.
2.1.1. Western blotting
Western blotting is a widely applied, exceedingly useful laboratory technique used to detect specific proteins within biological samples. It can also be used to detect changes in protein abundance and confirm protein–protein interactions. In brief, the technique involves the separation of proteins by gel electrophoresis; they are then transferred onto a membrane, and subsequently probed with a primary antibody specific to the target protein of interest [Citation28].
2.1.2. Chromatography based techniques
Affinity, size exclusion, and ion exchange chromatography-based proteomic techniques are sets of methods used to separate, identify, and quantify proteins within given samples based on their physicochemical properties.
2.1.2.1. Affinity chromatography
This technique separates proteins based on their specific binding to a ligand immobilized on a chromatography resin [Citation29]. The immobilized ligand can be a small molecule, protein, or nucleic acid that has a high affinity for the target molecule. There are various types of affinity chromatography; protein A chromatography is used to purify antibodies, immobilized metal ion affinity chromatography (IMAC) is used to purify proteins that have histidine tags, lectin chromatography utilizes specific lectins as the immobilized ligand, and DNA affinity chromatography utilizes single-stranded DNA as the immobilized ligand [Citation30].
2.1.2.2. Size-exclusion chromatography
Size exclusion chromatography is a non-denaturing technique that separates proteins based on their size and shape without altering their structure or activity [Citation31]. The stationary phase is typically composed of porous beads made from materials such as dextran, agarose, or polyacrylamide, with pore sizes ranging from 10 to 500 Å. The mobile phase is usually a buffer solution that is optimized for the separation of the target molecules [Citation32].
2.1.2.3. Ion-exchange chromatography
This method separates proteins based on the charged groups on its surface (anionic and cationic) and later on the basis of physiological pH [Citation33]. Ion-exchange chromatography can be performed in two modes: cation-exchange chromatography and anion-exchange chromatography. Cation-exchange chromatography separates negatively charged molecules by attracting them to positively charged stationary phase materials, while anion-exchange chromatography separates positively charged molecules by attracting them to negatively charged stationary phase materials [Citation34].
2.1.3. ELISA
ELISA is a commonly used laboratory technique to detect and quantify specific proteins, antibodies, and other molecules in a sample [Citation35].
2.1.4. Multiplex Luminex
Luminex uses color-coded polystyrene beads (5.6 µm in diameter) that are internally dyed with different proportions of red and infrared fluorophores corresponding to a distinct spectral signature, or bead region. The surface of the bead can be coated with a different capture antibody in order to identify the antigen of interest within the test samples. The beads with bound target proteins are separated by laser excitation and quantitated. Beads coupled with different antibodies, each with a distinct fluorescence signature, are mixed, thus enabling simultaneous detection of multiple target proteins in a single well of a 96-well plate. This high-throughput system allows for rapid multiplexed analyses of panels, and proteomic biomarkers associated with select disease states. This general testing platform, therefore, is of high practical value in a clinical setting [Citation36].
2.1.5. Meso scale discovery (MSD) platform
MSD immunoassays are based on a technology combining electrochemiluminescence and multi-array for detection of multiple proteins in a single sample. This is a sandwich-based assay consisting of a Multi-Spot microplate, where each spot is coated with unique capture antibodies. This assay works on the same principle as ELISA but uses an electrochemiluminescence signal as the detection method, and it has the capability to measure multiple protein analytes in a single well. Unlike ELISA plate readers, MSD instruments provide increased throughput with fast read times. Furthermore, MSD has a wider dynamic range, while Luminex has higher sensitivity and precision [Citation37].
2.1.6. Aptamer-based SOMAscan assay
The oligonucleotide aptamer-based SOMAscan platform of SomaLogic is used for quantification of >1,300 proteins or more [Citation38]. Similar to antibody-based assays, these aptamers are nucleotide sequences to bind specific antigens and, in this way, this assay platform can detect very low abundance proteins within test samples which compares favorably to other immunoassay systems. However, the large SOMAscan platform is limited in terms of providing only relative quantitative information and not absolute concentrations of target molecules of interest.
2.2. Advanced proteomic technology
Advanced proteomic technologies are a set of techniques used to study the complex and dynamic nature of the proteome. These technologies include MS, protein microarrays, high-resolution liquid chromatography, structural proteomics, and top-down proteomics. These advanced proteomic techniques have revolutionized our understanding of the proteome and have enabled the discovery of new biomarkers, drug targets, and therapeutic interventions [Citation22].
2.2.1. Gel-based proteomic techniques
Gel-based proteomic approaches are powerful techniques used to separate, identify, and quantify proteins in complex biological samples. Three commonly used gel-based proteomic approaches are SDS-PAGE (known as two-dimensional polyacrylamide gel electrophoresis), 2D-PAGE, and 2D-DIGE (two-dimensional in gel electrophoresis). They have been widely used in a range of fields, including basic research, clinical diagnosis, and drug discovery. SDS-PAGE is a one-dimensional gel electrophoresis used to separate proteins based on their molecular weight [Citation39]. 2D-PAGE leverages the power of SDS-PAGE by separating proteins based on both their molecular weight and isoelectric point (pI) [Citation40]. 2D-DIGE is a variation of 2D-PAGE that allows for the direct comparison of two protein samples on the same gel [Citation41].
2.2.2. Mass spectrometry (MS)
MS is a powerful analytical technique that can be used to analyze the mass and composition of target molecules within test samples. There are several different types of MS including discovery, targeted, and isotopic labeling. Discovery MS involves the analysis of complex mixtures of molecules, such as proteins or metabolites and the use of high-resolution mass spectrometers, such as Orbitrap or Fourier-transform ion cyclotron resonance (FT-ICR) [Citation42]. Discovery proteomics aims to identify and quantify as many proteins as possible in a given sample, without prior knowledge or selection of the proteins of interest. Targeted MS, on the other hand, is a more focused approach that is used to measure the abundance of a predefined set of proteins of interest using selected reaction monitoring (SRM) or parallel reaction monitoring (PRM) in a sample with the use of triple quadrupole mass spectrometers, which are highly sensitive and selective instruments that can be used to quantify small molecules [Citation43]. The newly developed Olink Proteomics technique falls under the category of targeted proteomics. It is a multiplexed immunoassay-based approach designed to measure protein biomarkers in biological samples, and it uses proximity extension assay (PEA) technology, which combines DNA oligonucleotide pairs linked to antibodies. When the target proteins bind to their respective antibody pairs, the oligonucleotides are brought into proximity, enabling their hybridization and subsequent amplification for quantification. The technique allows for the simultaneous measurement of multiple protein biomarkers in a highly sensitive and specific manner [Citation44]. Isotopic labeling aims to compare the relative abundance of proteins between different samples or conditions using stable isotopes to label the peptides or proteins, and is a technique that is used to track the movement of atoms or molecules through metabolic pathways. Isotopes are atoms that have the same number of protons but different numbers of neutrons and can be used to label molecules so that they can be tracked using MS. There are several types of isotopic labeling including stable isotopic labeling, in which stable isotopes are used to label molecules, and radioactive isotopic labeling, in which radioactive isotopes are used to label molecules [Citation45]. Data-independent acquisition (DIA) is a relatively recent enhancement in the field of MS-based proteomics. This innovative approach enables thorough and unbiased data collection by generating fragment ion spectra for every precursor ion within a predefined mass range. It can be seamlessly integrated into targeted proteomics workflows, allowing for the simultaneous quantification of multiple proteins of interest. DIA is a versatile MS approach that encompasses different strategies for acquiring comprehensive data. SWATH is known for its comprehensive coverage [Citation46], PRM is specialized for targeted quantification, and MSX [Citation25,Citation47,Citation48] enhances throughput by simultaneously fragmenting multiple precursor ions. These approaches diverge in how they segment the mass range into smaller windows for fragmentation and in their methods for capturing the resulting fragment ion spectra. The choice of strategy depends on the specific research goals, whether they involve comprehensive proteome analysis, precise quantification of specific proteins, or high-throughput screening. The usage of DIA in combination with stable isotope labeling is quite rare and is typically applied in specialized scenarios like single-cell proteomics [Citation49]. However, there are some examples of its use in proteomics research. For example, a study by Wang et al. [Citation50] used DIA with stable isotope labeling to quantify protein expression in human plasma. Its remarkable versatility, which encompasses both identification and quantification capabilities, positions DIA as an invaluable asset in the toolkit of proteomic research [Citation51].
2.2.3. Protein microarray
Protein microarrays have proven to be useful tools for high-throughput analysis of protein–protein interactions, protein–DNA interactions, and protein-small molecule interactions. There are different types of protein microarrays, including reverse phase, analytical, and functional microarrays. Reverse phase protein microarrays (RPPAs) allow for the quantitative analysis of protein expression levels in biological samples. This technique involves immobilizing samples of lysed cells or tissues onto a microarray and probing them with a panel of specific antibodies against proteins of interest. The antibodies are then detected using fluorescent or chemiluminescent signals, and the resulting data is used to quantitatively measure protein expression levels [Citation52]. Analytical protein microarrays are used for the detection and quantification of specific proteins or protein modifications. These microarrays are typically made by printing specific antibodies or protein-binding molecules onto a solid support, such as a glass slide or a membrane. The protein of interest is then applied to the array, and any bound protein is detected using a variety of labeling or detection techniques, such as fluorescence or chemiluminescence [Citation53]. Functional protein microarrays are used to study protein–protein interactions or protein-small molecule interactions. These microarrays typically contain a library of purified proteins, which are printed onto solid supports. The arrays are then probed with fluorescently labeled target proteins or small molecules, and any bindings are detected using fluorescence imaging. Functional protein microarrays can be used to identify novel protein interactions or to screen large libraries of compounds for their ability to bind to specific proteins [Citation53].
2.2.4. Quantitative proteomics
Various methods have been developed for quantitative proteomics, enabling the comparison of protein expression levels between different samples. Label-free methods and labeling methods using MS1 and MS2-based quantification are two categories of MS-based quantification methods for proteomic analysis. These include label-based approaches like stable isotope labeling with amino acids in cell culture (SILAC) or isobaric tags for relative and absolute quantitation (iTRAQ/TMT), ICAT as well as label-free methods based on spectral counting, or intensity-based quantification. Label-free methods do not use any chemical or isotopic labels to mark the peptides or proteins in different samples, but rely on the intensity or count of the peptide signals in the MS spectrum to estimate their relative abundance [Citation54]. Labeling methods use chemical or isotopic labels to mark the peptides or proteins in different samples, which can be distinguished by their mass difference in the MS spectrum and are used to quantify their relative abundance [Citation55]. MS1-based quantification methods use the intensity of the peptide precursor ions in the survey scan (MS1) to measure their relative abundance. MS2-based quantification methods use the intensity or count of the peptide fragment ions in the tandem mass spectrometry scan (MS2) to measure their relative abundance.
Label-Free Methods: Label-free quantification methods in proteomics rely on the relative abundance of peptides or proteins in different samples without the use of chemical or isotopic labels. These methods compare the intensities or spectral counts of peptides between samples to determine relative protein abundance. Common label-free techniques include spectral counting and various intensity-based methods that rely on quantifying ion intensities, such as spectral index or precursor ion intensity, which essentially involves extracted ion chromatograms. These techniques leverage MS’s capability to measure ions for quantification purposes. Label-free quantification is advantageous for its simplicity, cost-effectiveness, and suitability for a wide range of experimental designs [Citation54]. Labeling methods: iTRAQ/TMT, (isobaric tags for relative and absolute quantitation/tandem mass tag). TMT and iTRAQ are indeed isobaric labeling techniques. The reporters are isobaric and have the same mass, but they differ in their fragmentation patterns and can be used to quantify peptide abundance. They label peptides with tags that have the same nominal mass but different isotopic compositions. The reporters, however, have different masses and are used for quantification [Citation56]. iTRAQ and TMT differ in some aspects, such as the number of tags available, the structure of the tags, and the fragmentation behavior of the tags [Citation55]. The labeled peptides are then combined and analyzed by MS, allowing for the relative quantitation of multiple samples in a single experiment [Citation57]. ICAT is a method of quantitative proteomics that involves labeling proteins with isotopically labeled cysteine-containing tags. The labeled proteins are then digested with a protease and analyzed by MS, allowing for the quantitation of proteins between two samples [Citation58]. SILAC is a metabolic labeling method that involves incorporating isotopically labeled amino acids into proteins during cell culture [Citation59].
2.3. High-throughput proteomics
High-throughput proteomic technologies refer to a range of techniques that enable the analysis of large numbers of proteins in a high-throughput manner. These technologies are critical for large-scale proteomic studies, such as those aimed at establishing basic, molecular processes of disease development, as well as for drug discovery or biomarker identification. Some of the key high-throughput proteomic technologies include MS, protein microarrays, tandem affinity purification, chemical cross-linking, and quantitative proteomics.
2.4. Bioinformatics analysis
Bioinformatics plays an essential role in the analysis and interpretation of proteomic data. For full and proper analyses, massive amounts of data generated by proteomic technologies, such as MS and protein microarrays, require sophisticated computational tools and algorithms to extract meaningful information. Some key bioinformatics analysis steps in proteomic technology include the following processes:
2.4.1. Multivariate analyses
Multivariate analyses in proteomics are statistical methods that can handle and interpret data with multiple variables such as protein abundance, expression, modification, interaction, etc. Multivariate analyses can reveal complex patterns and relationships among proteins and can also reduce the dimensionality and sparsity of proteomic data, improve the accuracy and specificity of protein identification and quantification, and facilitate the biological interpretation and functional annotation of proteomic data. Multivariate techniques such as Support Vector Machines (SVM), Partial Least Squares (PLS), or Elastic Net Regression are commonly employed for biomarker identification and validation [Citation60].
2.4.2. Database searching
Proteomic data is typically analyzed by searching against a protein database to identify proteins and their post-translational modifications (PTMs). This step involves the use of some key software tools and databases such as Mascot [Citation61], Uniport [Citation62], Proteome Discoverer [Citation63], PRIDE [Citation64], Skyline [Citation65], or MaxQuant [Citation63] to match experimental spectra to theoretical spectra generated from protein sequences.
2.4.3. Protein quantification
Proteomic technologies such as iTRAQ, ICAT, and SILAC generate quantitative data, which require specialized software tools to analyze. These tools typically involve normalization, statistical analysis, and visualization of the data [Citation63].
2.4.4. Post-translational (PTM) analysis
PTMs play important roles in protein function and regulation, and proteomic technologies can be used to identify and quantify PTMs. Bioinformatics analysis of PTMs typically involves the use of specialized algorithms and databases, such as PhosphoSitePlus or UniProt, to identify modified peptides and assign specific PTMs [Citation62,Citation63].
2.4.5. Functional analysis
Proteomic data can be used to infer protein function and identify potential biological pathways or networks. Functional analysis typically involves the use of specialized tools, such as gene ontology (GO) analysis or pathway analysis, to identify over-represented functional categories or enriched pathways. These include PANTHER [Citation66], Gene Ontology (GO) analysis [Citation67], and David database [Citation68].
2.4.6. Integration with other data types
Proteomic data can be integrated with other types of omics data, such as transcriptomic or genomic data, to provide a more comprehensive view of biological systems. Bioinformatics tools, such as ingenuity pathway analysis (IPA) or Cytoscape, can be used to integrate and visualize different types of omics data.
3. Radiation-induced altered proteomic profiles
A sizable number of studies have been reported demonstrating radiation-induced alterations of proteomes in body fluids and organs within both small and large animals subjected to different modes, levels, and qualities of ionizing radiation [Citation12,Citation69]. Recent studies have used state of the art omic platforms such as DIGE, protein microarray, NMR-spectroscopy, MS, and X-ray crystallography to demonstrate the proteomic changes in serum, plasma, and various tissue samples collected from irradiated and unirradiated animals ().
Table 1. Proteomic studies for radiation biomarkers using various analysis platforms and different animal models.
Table 2. Studies for cytokines/growth factors using MCMs in mice using various analysis platforms.
Table 3. Studies for cytokines/growth factors using MCMs in minipigs, canines, and NHPs using various analysis platforms.
Table 4. Proteomic studies in murine models using radiation MCMs for ARS and various platforms for analysis.
Table 5. Proteomic studies in an NHP model using radiation MCMs for ARS and the NanoUPLC-MS/MS platform.
3.1. Induction of growth factors and other cytokines by radiation exposure
Studies from several institutions have reported induction of a large number of cytokines and growth factors in response to radiation exposure in different animal models with or without countermeasure use [Citation70,Citation71]. Though the majority of studies have used western blot, chromatography, ELISA, and multiplex Luminex for measuring radiation-induced responses of cytokines and growth factors, there are also reports using the MSD MULTI-ARRAY 96-well plate HT platform [Citation70,Citation72].
3.1.1. Animal models of radiation injury and related proteome responses
Small animal murine model(s): In an earlier study, it has been shown that exposure to ionizing radiation induced significant levels of G-CSF and interleukin-6 (IL-6) in mice receiving 9.2 Gy 60Co gamma-radiation. Maximal levels of G-CSF were observed in peripheral blood of mice 8 h post-irradiation, and IL-6 levels were highest at 12 h post-irradiation [Citation73]. Further, it was shown that the administration of specific antibodies to G-CSF significantly enhanced mortality in acutely irradiated mice. These reported findings clearly suggest that acute radiation exposure induces G-CSF and IL-6, and that neutralizing antibodies to G-CSF aggravates the injury caused by ionizing radiation to select vital organ systems of the body such as the hematopoietic and GI organ systems. This study also suggests that the irradiation-induced G-CSF may play an important role in injury recovery as well. In this context, it is important to note that recombinant products of G-CSF (Neupogen and Neulasta) have already been approved by the US FDA for mitigating acute, potentially lethal radiation injuries [Citation74–77]. Two additional related agents, granulocyte-macrophage colony stimulating factor (GM-CSF, Leukine) and Nplate (thrombopoietin (TPO) analog), have also been approved by the US FDA for comparable radiation injuries [Citation78–81]. Furthermore, studies have demonstrated induction of other cytokine- or inflammatory-like agents (e.g. C-reactive proteins) in various murine models of radiation exposure injuries under a variety of experimental conditions [Citation12,Citation82–95]. For example, the immunological effector molecule, IL-18, has been shown to be induced (modulated) in rodents in response to total body-gamma ray exposures as evidenced by elevated levels of IL-18 in both blood and urine samples [Citation88,Citation96,Citation97].
While the majority of these studies have involved total-body exposures using gamma rays (60Co gamma rays), the effects of partial-body exposures have employed mainly small animal research platforms (SARRP) or linear accelerators (LINAC) for the experimental irradiations. One research group, for example, has conducted several studies using CD2F1 and BALB/c male mice using both types of irradiation platforms (i.e. 60Co gamma and SARRP). These studies have reported not only on the modulation of G-CSF following irradiation, but also on the growth arrest of targeted cells and temporally related DNA damage-α (GADD45α), serum amyloid A (SAA), TPO, erythropoietin (EPO), and FMS-related tyrosine kinase 3 ligand (FLT-3 L) responses [Citation91,Citation92,Citation95,Citation98,Citation99]. Interestingly, under equivalent radiation exposures/doses, 60Co gamma (megavoltage) appears less injurious than SARRP X-ray (orthovoltage) [Citation100].
The latter observations appear to suggest the influence of the ‘quality’ of ionizing radiation being employed in these studies. To this point, there are a few studies that have used mixed-field radiation (neutrons and gamma fields generated by a TRIGA reactor). One mixed-field study was performed over a broad dose range (1.5–6 Gy), with dose rates of either 0.6 or 1.9 Gy/min, and two different proportions of neutrons and gammas (either 67% neutrons plus 33% gammas or 30% neutrons plus 70% gammas) using B6D2F1 mice. Blood was collected 1, 2, 4, and 7 d after TBI [Citation86]. Irradiation-induced proteins were measured using the MSD’s high-throughput multi-array platform and ELISA. Results demonstrated dose- and time-dependent changes in FLT-3 L, IL-5, IL-10, IL-12, IL-18, G-CSF, GM-CSF, TPO, EPO, SAA, lipopolysaccharide binding protein (LPSBP), surface plasma neutrophil (CD45) and lymphocyte (CD27) markers, ratio of CD45 to CD27, and procalcitonin (PCT). It is important to note that regarding the above comments concerning ‘radiation quality’ effects, higher levels of IL-18, G-CSF and GM-CSF, SAA, and PCT were observed in animals irradiated with 67% neutrons + 33% gamma compared to those irradiated with 30% neutrons + 70% gamma. Comparisons were also made with another study conducted with TBI using gamma-rays (60Co gamma ray source) under similar conditions [Citation85]. Results from a 60Co gamma study demonstrated significant dose- and time-dependent changes with all cytokines listed above. No differences were observed in cytokines between different dose-rate groups. Furthermore, no differences were observed between two sexes. Again, as suggested earlier, radiation-quality comparisons demonstrated that equivalent doses of gamma rays and mixed-field radiation do not produce equivalent biological effects. H-ARS occurs at lower doses of mixed-field radiation, accompanied by increased mortality. Cytokines in the 60Cogamma study with 3 Gy were equivalent to the mixed field study with 1.5 Gy.
Large animal/minipig models: These porcine models have also been used sparingly for select radiobiological studies, including a cytokine study of the induction pattern of IL-18 following acute irradiation [Citation101]. Gottingen minipigs (males) were subjected to 1.6 or 1.78 Gy of 60Co gamma rays. Prior to or following the exposures, blood samples were collected periodically (hours to days), and serum samples were prepared and analyzed by ELISA. Modulatory patterns, specifically elevated blood levels of IL-18, were noted at various time points relative to the radiation exposure [Citation101].
Large animal/rhesus nonhuman primate models: Nonhuman primates (NHPs) have been extensively used of late to explore the types and patterns of injury-induction, along with the associated proteomic responses under a range of doses of radiation exposure conditions. The use of these NHP models has provided clear demonstration of specific induction patterns of select cytokines of interest (e.g. G-CSF, IL-6, IL-8, IL-18, etc.) that have been implicated in acute injury and/or repair processes within the hematopoietic and GI systems over specific post-exposure periods [Citation102–106]. These cytokine assays were conducted using mainly multiplex Luminex testing platforms. Of note, the kinetic induction patterns of these cytokines appeared quite distinct. In yet another study of NHPs acutely exposed to a range of gamma rays (1.0–8.5 Gy TBI at 0.55 Gy/min), a number of plasma proteins of interest (e.g. CRP, SAA, IL-6, and FLT-3 L) were analyzed over a short-time course following exposure (i.e. at 0.24, 1, 2, 3, 4, and 7 days post-irradiation) and were found to be significantly altered. This observation gave rise to the working hypotheses that such dynamic, quantitative changes of these blood plasma proteins may function as prognostic indicators of the time course and severity of ARS [Citation90]. Further, the combination of response patterns of multiple proteins may provide still greater accuracy for early, acute radiation injury assessment than would any single protein response pattern.
Results of subsequent studies utilizing NHPs tend to support our ‘working hypothesis’ (i.e. induction patterns of select plasma proteins are sufficiently distinct as to provide a prognostic indicator of acute radiation injury). Specifically, these follow-up investigations showed that cytokine induction patterns generally followed similar trends over a range of radiation doses, apart from a few instances (e.g. deviant IL-7 and MCP-1 response patterns), where certain radiation dose groups deviated from the other radiation dose groups [Citation102].
In another supporting study that compared the induction of a large number of cytokine- or cytokine-like plasma proteins (FLT-3 L, CD14, TPO, EPO, IL-12 p40, AMY2A, CRP, SAA, G-CSF, GM-CSF, PCT, LBP, CD177, CD20, and CD27) in NHPs under both full and minimal supportive care, a consistent, temporal modulation of many of these blood plasma proteins was observed [Citation72].
Ancillary cytokine studies have utilized NHPs and have focused on primary, irradiation-induced pulmonary injuries and subsequent repair responses. In one study, the levels of some 24 cytokines were measured in serially collected plasma samples (as well as the terminal sampling of lung tissues) of NHPs subjected to intense (11.5 Gy) doses of PBI (thoracic). Of the cytokines assayed, those that were shown to be significantly altered as a result of whole-thoracic-lung irradiation (WTLI) include seven plasma cytokines (interferon gamma-induced protein 10 (IP-10), MCP-1, IL-12, IL-15, IL-16, IL-7 and IL-6) along with seven of the assayed cytokines (i.e. IP-10, MCP-1, MIP-1α, TARC (thymus- and activation-regulated chemokine), IL-17, TNF-β and IL-6) in lung tissue. Of note was the finding that the terminal plasma concentrations of IP-10, TARC, IL-12, IL-15 and IL-6 were significantly correlated with their levels in the lung tissue [Citation107].
3.2. Proteomic analysis using other high-throughput platforms
During the last 10 years, there have been numerous reports using different animal models, particularly murine and NHPs, and various proteomic platforms (e.g. LC-MS and 2D-DIGE) to study the radiation exposure-induced proteomic changes in serum/plasma or other tissue samples ( and 3). In general, all of these studies provide foundational support for both the identification and validation of biomarkers for radiation injury-related studies.
Figure 2. Schematic of two-dimensional differential in-gel electrophoresis and animal study, sample preparation, and subsequent data analysis.
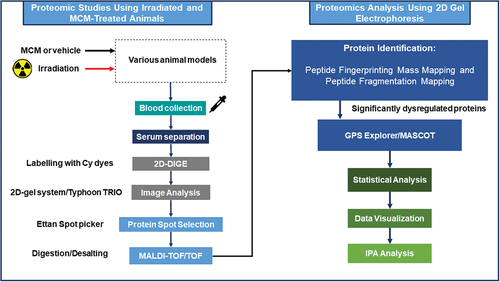
Using a C57BL/6J mouse model of radiation injury, proteomic analyses were carried out using LC-MS/MS of GI (upper ileum) tissues collected from animals during early periods (1, 3, 6 d) following acute TBI (8, 10, 12, 14 Gy) [Citation108]. Time- and dose-dependent protein changes were revealed with select upstream, regulatory proteins of key canonical pathways being altered by the acute exposures [Citation108]. Using the same strain of mice subjected to WTLI, and with proteomic analyses focused on the early, post-exposure (1, 3, 6 d) responses of pulmonary tissues, several response trends were observed in the proteome alterations, including protein changes greater than 10-fold, proteins consistently up- or down-regulated at all time points and radiation dose, and time and dose-dependent proteomic changes [Citation109].
Still further advanced proteomic studies using small rodent mouse models of radiation injury have been reported, including a report demonstrating marked alterations of proteomic profiles (with splenic tissues) seen under steady state to those profiles observed during early post-exposure. For example, the upregulation of the Wnt signaling pathway and actin-cytoskeleton linked proteins in mediating radiation injury responses in spleens of CD2F1 mice subjected to TBI was revealed by MS, reverse transcription-polymerase chain reaction (RT-PCR), and western blot analyses of sampled tissues [Citation110]. Comparable studies have been conducted and have reported major shifts of proteomic profiles of select organs/tissues of acutely irradiated animals. For example, 2D-DIGE followed by MS and IPA demonstrated a change in the expression of proteins in jejunum and serum samples [Citation111,Citation112]. IPA revealed a network of associated proteins including β2-glycoprotein 1, α1-acid glycoprotein 1, α2-macroglobulin, complement C3, mannose-binding protein C, and major urinary protein 6 that are involved in cellular movement, immune cell trafficking, and inflammatory response. These could be translated as biomarkers of radiation injury. A study with CBA/CaJ mice and137Cs TBI at a dose of 0–3 Gy using plasma samples and 2D-DIGE/MS at 2 and 7 d post-irradiation was conducted [Citation113]. Although different proteins (mostly involved in inflammatory responses) were detected in exposed mice, alterations in expression-levels of clusterin, gelsolin, kininogen, and alpha-2-HS-glycoproteins were found at both times. Still another study compared the expression of five proteomic biomarkers (FLT-3 L, MMP9 (matrix metalloproteinase 9), SAA, pentraxin 3 (PTX3), and fibrinogen (FGB)) across females of five murine strains (C57BL6, BALB/c, C3H/HeJ, CD2F1, and CD-1) to test a multivariate dose prediction model based on a single C57BL/6 strain [Citation114]. Significant differences were found between irradiation-induced expression of FLT-3 L, MMP9, SAA, PTX3, and FGB among the five strains. This study suggests that strain-specific differences exist for biomarker expression and the use of multiple biomarkers for dose prediction strengthens the predictive accuracy of a model. There are additional studies using murine models that serve to further strengthen the predictive features of our working model [Citation115–120].
A study to identify candidate radiation-responsive protein biomarkers in human lymphocytes in vivo using humanized NOD SCID gamma (Hu-NSG) mouse model has been reported. Human CD45+ cells were collected from the Hu-NSG mouse spleen 3 d after X-ray exposure (0–2 Gy, 88 cGy/min) and changes were analyzed by MS. A total of 46 proteins were differentially expressed in response to X-ray exposure [Citation121]. The combination of FDXR, ACTN1, and DDB2 showed the lowest mean absolute errors (≤0.13 Gy) and highest coefficients of determination (R2 = 0.96).
A Sprague Dawley rat model of radiation injuries that arise relatively early (1–7 d) following acute, but relatively low ionizing radiation exposures (60Co gamma rays, 1–5 Gy TBI) has demonstrated significant shifts using advanced analytics (i.e. tandem mass tag labeling, in combination with LC-MS/MS) in the blood concentrations of a select number of plasma proteins, namely alpha-2-macroglobulin, chromogranin-A, and glutathione peroxidase [Citation122]. In another related report using comparable analytics [Citation123], significant shifts of urinary proteins were noted following either TBI or partial-body (LKI - localized kidney irradiation) exposures of large rodents (WAG/RIJICmer rats). Notably, urinary protein profiles changed markedly, but selectively following the two exposure protocols (TBI and LKI). Following TBI, fetuin-B, beta-glucuronidase, tissue kallikrein, chondroitin sulfate proteoglycan NG2, and vitamin D-dependent calcium binding protein were detected, while following LKI exposures, RNA binding protein 19, major urinary protein-1, WD repeat and FYVE domain containing protein 3, sorting nexin-8, neuron navigator, Dapper homolog 3, ankycorbin, and aquaporin. Urine protein (Up) and creatinine (Uc) ratios (Up/Uc) and urinary albumin abundance decreased in both TBI and LKI groups. As such, these reported observations suggest that the urine proteome may be useful in determining organ-specific (kidney) injury.
Minipigs have been added recently to the array of mammalian species currently being used to evaluate the role played by the proteome in manifesting acute radiation injuries using advanced analytics [Citation124–126]. A recently applied, novel aptamer-based proteomic assay has been used to investigate changes to the blood proteome after TBI across four different mammalian species including mice, minipigs, NHPs, and humans. Plasma samples, both unirradiated and irradiated either in situ or in vitro, were collected and assayed using the aptamer-based SomaLogic SomaScan assay to evaluate changes in the expression of 1,310 protein analytes. Detectable values were obtained for all 1,310 proteins, and the HIST1H1C protein was shown to be radiation responsive in humans, NHPs, and murine species.
There are several studies using rhesus macaque NHPs and TBI or PBI models for studying proteomic changes in response to irradiation. Urine and plasma samples from a study with NHPs exposed to high, lethal doses of gamma irradiation (6.7 and 7.4 Gy doses delivered at relatively high exposure dose rates, 0.6 Gy/min) have been analyzed using high-resolution MS analysis [Citation127,Citation128]. A total of 2,346 and 663 proteins were identified in urine and plasma, respectively, and out of these proteins, 414 were found to be in common and significant. A total of 33 proteins from plasma and 46 from urine were found to be significantly altered in abundance. Furthermore, 10 proteins were found to significantly differentiate between both dose and time points in plasma and urine from the same animals [Citation127,Citation128].
In yet another study employing an advanced analytic procedure of NanoUPLC-MS/MS global molecular profiling of radiation-induced proteomic changes in blood samples collected from acutely irradiated NHPs (7.2 Gy), it was found that a total of 63, 69, 48, and 53 proteins were significantly dysregulated at 24, 36, 48, and 96 h post-irradiation, respectively [Citation20]. This study also revealed alterations of a host of type-specific proteins (e.g. proteins related to hemostasis, innate immunity, protein metabolism, etc.), as well as signs of generalized dysregulation of basic cell/tissue networks.
Advanced proteomic analytics have been applied to studies investigating the pathophysiology of radiation injury within NHPs following PBI. As an example, one such study involved high dose PBI (12 Gy, 6 MV LINAC-derived photons at 0.8 Gy min) but with 2.5% marrow sparing. Proteome analyses (using LC-MS/MS) of various blood and tissue samples collected over a 3-week period were conducted using LC-MS/MS [Citation129–132]. A number of trends were observed, including pronounced qualitative/quantitative changes as well as changes that were consistently up- or down-regulated at all time points and dose levels investigated.
In jejunum, 3,245 unique proteins were quantified out of more 3,700 identified proteins. A total of 289 quantified proteins demonstrated consistent and significant responses across at least three time points, with 263 up- and 26 down-regulated [Citation132]. In the kidney, 3,432 unique proteins were identified. A total of 265 proteins demonstrated significant responses across at least three time points, of which 230 were up- and 35 were down-regulated [Citation130]. In the lung, 3,101 unique proteins were identified and a total of 252 showed consistent response, of which 215 were up- and 37 were down-regulated [Citation131]. Investigation with plasma samples suggested that CRP, SAA1, and CASS4 (Cas scaffolding protein family member 4) are the most promising proteins as biomarkers for radiation injury [Citation129]. In brief, significant pathway and biological process alterations were observed after PBI.
3.3. Multi-omics approach using NHP model
While several studies have reported putative metabolite, protein, or miRNA-based biomarkers for predicting radiation exposure, few have used a multi-omics approach in large animal models for reproducibility and possible clinical translation [Citation12,Citation19,Citation133–135]. Such multi-omics studies have used TBI as well as PBI models.
NHPs with different doses of TBI (60Co gamma ray) have been studied to delineate a multi-omics (proteomics and metabolomics) serum probability index. For example, in a recent report, blood plasma (serum) samples were collected from both male and female NHPs prior to acute irradiation during the initial period following exposure (1, 2, and 6 d post-irradiation) and were analyzed for select metabolites, lipids, and proteins of interest using MS analytics [Citation102]. A logistic regression model was used to develop a four-analyte panel that serves to identify unirradiated and irradiated animals within a given population with a high degree of predictability [Citation19]. Remarkably, the panel appeared to be highly efficacious in identifying radiation-exposed animals across all six radiation exposure levels (6 to 8.5 Gy) during early periods of testing (e.g. 24 h to 6 d post-exposure). The panel of putative biomarkers consisted of Acetyl-carnitine, PC (16:0/22:6), Serpin Family A9, and Suberylglycine [Citation19]. The panel’s protein biomarkers were validated with western blots of serum samples. Additional studies using other multi-analyte panels for specific dose assessments have also been reported, providing additional evidence of the utility of the ‘multi-omics’ approach. In sum, this study demonstrated the potential usefulness of a combinatorial molecular characterization. In particular, the use of multi-analyte panels of potential biomarkers for the development of minimally invasive assays for radiation dose assessments.
In addition to the above-mentioned studies that have focused on proteomic changes with TBI, there are additional, but related reports that have examined the effects of PBI. These reports certainly include but are not limited to attempts to better characterize the pathogenesis of select types of radiation-induced injuries using multi-omics approaches (proteomics and metabolomics) [Citation135,Citation136]. For example, the proteome and metabolome of left and right heart ventricles were quantitatively profiled in NHPs following 12 Gy PBI and with 2.5% bone marrow sparing over a time period of 3 weeks [Citation135]. Global proteome profiling identified >2,200 unique proteins, with 220 and 286 in the left and right ventricles, respectively. Targeted metabolomics analyzed a total of 229 metabolites, showing significant responses compared to baseline levels. Furthermore, pathway analyses using metabolomic and proteomic data demonstrated dysregulation of energy metabolism, inflammation, and myocardial functions, along with dysregulation of the retinoid homeostasis pathway. Related PBI-induced deregulatory effects (proteome and metabolome) within mesenteric lymph nodes of NHPs were also noted using multi-omic analytics [Citation136].
4. Proteome biomarkers and MCMs
There have been extended efforts to make use of cytokine-induction patterns following acute irradiation as potential biomarkers of radiation injuries, as well as bioindicators of the effectiveness of given agents designed to thwart such injuries. Several recent studies have utilized advanced, high-throughput analytics for these proteomic/MCM-based studies. This is an important area of MCM research, as the US FDA requires efficacy-based biomarkers for any given MCM to be approved under the Animal Rule. Further, such biomarkers are considered critical for drug dose conversions from animals to humans. Studies presented in list various MCMs, and these studies have separate groups without an MCM as a control demonstrating the effects of irradiation. Irradiated animal samples have been compared with samples derived from unirradiated animals to investigate the effects of radiation exposure.
4.1. Induction of G-CSF and other cytokines by MCMs and their role in radioprotection
Related to this research effort, a large number of MCMs have been shown to influence induction of cytokines and growth factors in unirradiated and irradiated murine and NHP models. Few studies have been conducted in minipig and canine models as well. For the sake of brevity, we highlight below the major findings of various reports that have used principally either small rodents, canines or NHP models of acute radiation injuries and related MCM radioprotective or mitigative actions.
Using a murine model, a number of potential useful MCMs have been extensively assessed for their radioprotective/mitigative/therapeutic actions and have shown that these radiation injury countering effects are mediated, at least in part, by their capacity to induce high blood and tissues levels of protective G-CSF. By blocking G-CSF induction (via infusion of specific antibody to G-CSF), the cytokine’s reparative effects of acute radiation injury can be effectively ablated. These MCMs are gamma-tocotrienol (GT3) [Citation137,Citation138], delta-tocotrienol (DT3) [Citation36], tocopherol succinate (TS) [Citation139,Citation140], entolimod (CBLB502) [Citation104,Citation105,Citation141], and 5-androstenediol (5-AED) [Citation142–144]. Again, all these agents have been shown to have the capacity to induce (during early post-exposure periods) high levels of G-CSF in a dose- and time-dependent manner.
A large number of MCMs have been used for studying cytokines/growth factors and related molecules using irradiated and/or unirradiated murine [Citation145] (), minipig [Citation146], and canine [Citation104] models (). Most notably these MCMs include agents such as entolimod (a polypeptide derived from the Salmonella filament protein flagellin and ligand for toll-like receptor 5 (TLR-5, CBLB502)) [Citation104,Citation105,Citation141,Citation147,Citation148], CBLB613 (naturally occurring mycoplasma-derived lipopeptide ligand for TLR-2/6) [Citation84,Citation149], CBLB612 (another synthetic lipopeptide that mimicking the structure of naturally occurring mycoplasma bacterial lipopeptide) [Citation82], BIO 300 (genistein) [Citation150,Citation151], 5-androstenediol (5-AED) [Citation143,Citation144], gamma-tocotrienol (GT3) [Citation103,Citation137,Citation138,Citation152–158], PEGylated IL-11 (BBT-059, a long acting PEGylated IL-11 analog created using site-specific PEGylation technology and is modified with a single branched 40 kDa-PEG at a cysteine residue (PEG-*179C) added to the C-terminus of the protein) [Citation159,Citation160], thrombopoietin mimetic (JNJ-26366821, a TPO mimetic with no sequence homology to endogenous TPO) [Citation161,Citation162], PLX-R18 Cells (3D-expanded placenta-derived stromal cell product) [Citation163,Citation164], tocopherol succinate (TS) [Citation70,Citation140,Citation165–168], delta-tocotrienol (DT3) [Citation36], captopril [Citation146,Citation169], and AEOL 10,150 (a superoxide dismutase) [Citation107,Citation170].
NHPs have been deemed by select investigators/regulatory agencies as ‘the gold standard’ of animal models for drug development, specifically for drugs being developed following the FDA Animal Rule [Citation171]. Several promising MCMs under development have been used for cytokine assessment using the NHP model. These MCMs include but are not limited to the following agents: entolimod [Citation104,Citation105,Citation141,Citation147], gamma tocotrienol [Citation103], and AEOL 10,150 [Citation107,Citation170]. The highlights of these studies are listed in .
4.2. Proteomic analysis with MCMs using advanced high-throughput platforms
There are several studies in murine and NHP models using LC-MS and 2D-DIGE and various MCMs to identify proteomes that can be used as biomarkers for efficacy assessments and drug dose conversions while developing new MCMs following the FDA’s Animal Rule guidelines () [Citation172].
Figure 3. Experimental design for biomarker discovery of radiation exposure and MCM treatments using nanoUPLC-MS/MS.
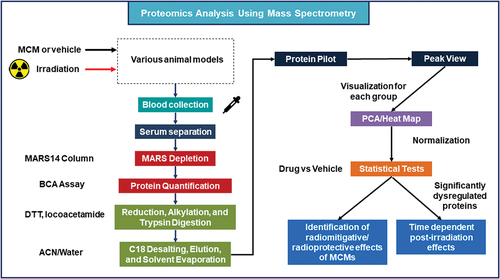
A recent report, for example, used 2D-DIGE/MS as the major analytic tool to demonstrate the modulating/mitigating effect(s) of one such MCM, GT3, on acute radiation-induced shifts within proteomes of both hematopoietic and gastrointestinal systems of acutely irradiated mice [Citation89]. Specifically, these analyses demonstrated a change in the expression of 18 proteins in response to TBI in blood plasma (serum) samples of the irradiated animals. Further, these changes were reversed with prophylactic treatment of GT3 [Citation112]. Significant expression changes in β2-glycoprotein 1, α1-acid glycoprotein 1, α2-macroglobulin, complement C3, mannose-binding protein C, and major urinary protein 6 were of particular interest after TBI, and were reversed with GT3 treatment. In another study with jejunum samples with the same mice strain, jejunal lysate from irradiated mice was compared to that of GT3-treated/irradiated mice, and 65 proteins were differentially expressed [Citation111].
There is a report for the analysis of protein expression in spleen tissue of male CD2F1 mice treated with GT3 with 7 Gy 60Co gamma TBI (). Additional MCM studies have employed advanced analytics to evaluate proteomic shifts induced by acute irradiation or by the administration of a given MCM (e.g. TS [Citation111,Citation167,Citation173], G-003 M [Citation174], melatonin [Citation175], Ex-Rad [Citation20,Citation176–180], BIO 300 [Citation21,Citation150,Citation181–186], etc.) under test. The major findings of these limited studies are listed in .
5. Challenges and limitations
Proteins, along with carbohydrates, lipids, and nucleic acids serve as key, fundamentally important structural and functional elements of all cells and tissues. These vitally important molecules often change dynamically over time, while undergoing selective modifications during basic life processes, such as during cell reproduction, differentiation, maturation, aging, etc. As noted here in this review, changing patterns of protein expression often accompany a variety of externally driven micro-environmental events (e.g. acute ionizing radiation exposures) or progression of given diseases, or in response to various drug treatments. Hence, it is abundantly clear that measurements of such proteomic shifts or changes, induced or otherwise, within cells, body fluids, or tissues are of fundamental importance for biomedicine. More specifically, and in terms of the primary subject matter under discussion here, it would seem prudent, if not critical to extend and perhaps expand the research effort to identify, develop, and validate reliable and sensitive biomarkers for acute injury elicited by ionizing radiation. As mentioned above, these putative, proteome-based biomarkers are urgently needed to improve radiation dose assessments and MCM drug dose conversions from animal models to humans. These drug dose conversions present a number of substantial analytical and clinical challenges that need to be met and overcome prior to making use of any new, putatively predictive proteome-based biomarker of MCM efficacy from animal models to clinical use. For example, challenging variables such as sample collection, processing, and storage conditions of collected samples prior to analysis may influence downstream proteomic analyses and biomarker detection. These variables need to be accounted for in any/all work related to biomarker identification and validation. The selection of proteome analysis platforms and data processing as well as tools used for computational analysis are critical for discrimination between samples obtained from the radiation exposed and unexposed study subjects. Similarly, the above factors are also important for discrimination between samples collected from the MCM-treated and untreated study subjects. The hope here is that future studies will identify and validate specific proteomes that serve as predictive/prognostic biomarkers for specific types and conditions of ionizing radiation exposures and related injuries stemming from exposures.
Though there are advancements in the area of proteomic biomarkers, the clinical translation has proved challenging. Some of such obstructions include assays failing to meet clinical precision or the absence of comprehensive evidence that changes in these biomarkers lead to crucial health results. Although there is a vast amount of raw data concerning noted changes within the proteome following ionizing radiation exposures, the sorting, analyses and assembly of such data in order to develop useful algorithms of acute ionizing radiation-related injuries largely remains unaccomplished. In this regard, the field of bioinformatics is progressing rapidly, especially for the analyses of proteomic data and perhaps for the advent of new and improved biomedical tools to assess ionizing radiation exposures, related injuries, and the safety and efficacy of given MCMs under development.
6. Conclusion
New and improved methods for the assessment of populations at risk following unwanted exposures (i.e. accidental or intentional) to ionizing radiation is currently deemed a high priority issue by federal/state emergency response planners responsible for developing and promulgating medical contingency plans of any/all mass casualty situations. The effort to identify and to develop accurate, sensitive, and reliable biomarkers of ionizing radiation-associated exposures and/or consequent injuries are integral to this research effort to improve the effectiveness of current medical emergency plans for any given nuclear/radiological exposure contingency. As illustrated here in this brief review, there appears to be substantial utility in pursuing the use of advanced, radiation-related proteomics as a primary vehicle in attempting to achieve the overall goal of developing radiation/injury-specific biomarkers with improved prognostic, diagnostic, or predictive features.
The reality is that the tools we have available for either radiation exposure estimates or for injury assessments are not only labor intensive but time-consuming and often unreliable. For example, the utility of dose estimates based on physical dosimetry is limited. By contrast, current biodosimetric procedures in general are reasonably sensitive and accurate, but often are technically difficult to perform and inordinately constrained. Due to space limitation, we restricted the focus of this article to acute radiation effects and related issues. Unfortunately, we have not included more expanded descriptions of other types of radiobiological studies involving different radiation qualities and varying doses and dose-rates, e.g. extraterrestrial/space-related research or low-dose and/or protracted exposure-related studies [Citation187–191]. Several studies have demonstrated proteomic changes compared with samples collected from pre-exposure or sham irradiated versus post-irradiated animals in patterns that are dependent on radiation quality, dose, and time post-exposure [Citation12,Citation21].
Cytokines/growth factor-associated proteomes are most frequently detected and validated post-irradiation in animal models of ARS. Proteomics is a powerful approach to investigate the changes in these essential, growth regulatory proteins prior to the onset of clinical symptoms of radiation injury. Improvements in the techniques of proteome identification, associated databases, and bioinformatics have facilitated the development of proteome panels for FDA qualification and for the potential clinical use of the technology in the future. The fully FDA-qualified biomarker(s) of radiation exposure and injury are currently needed; however, there are no such biomarkers available. Further, at this moment, the recent proteomic developments highlight a number of research needs in order to move the field forward: first, there is a need to standardized basic protocols for proteome investigation; second, to validate putatively useful, recently identified targeted biomarkers; and third, to conduct studies with samples from both genders to rule out gender bias as well as additional studies with different age cohorts.
The plasma samples would be the samples of choice for measuring proteomes to determine the effects of radiation exposure. One of the challenges with plasma samples is the high abundance of proteins. More than 95% of the serum proteome is of ∼20 high abundance proteins. These proteins interfere with the detection of low abundance proteins.
7. Expert opinion
With time, proteomics has developed into a diverse scientific discipline of quantitative precision and sensitivity measurements of basic biological events. Advanced proteomic platforms now allow for a high-throughput of samples in addition to a wide variety of studies ranging from single-cell analyses to large populations of individuals [Citation192,Citation193]. New technological advancements may constitute significant steps toward moving proteomics into specialized clinical diagnostic applications [Citation194]. While protein identification based on biochemical techniques is qualitative, MS-based protein identification is highly computational by nature. MS is one of the more powerful technologies currently available to biomedical researchers for analyses of the proteome, with the combined analytics of LC-MS/MS lying at the center of current approaches being applied in the science of proteomics. Accordingly, well-established proteomic laboratories are generally equipped with LC-MS/MS systems that support various flow regimes. In regard to the latter, it is expected that in due course, micro-flow LC-MS/MS will replace nano-LC-MS/MS for large scale proteome analyses. It is also envisioned that ‘sub-proteome’ analyses will become more common, as will the analytics of relatively high abundance post-translational modifications (e.g. phosphorylation) of proteins become more amendable and accessible. The analysis of protein PTM is challenging. For PTMs such as phosphorylation and glycosylation, newer chromatographic techniques with higher resolving powers have been shown to be beneficial in the separation and subsequent analyses of such protein PTMs. The resolving capability of LC separations usually increases with flow rate but sensitivity of the measurement trends in the opposite direction [Citation195]. In brief, micro-flow LC-MS/MS can be useful for select types of proteomic analyses, e.g. body fluids, glycoproteomes and phosphoproteomic profiling of cell lines, etc. Isomeric phosphopeptides tend to be better resolved using micro-flow LC-MS/MS rather than with nano-flow LC-MS/MS, but with a significant loss in sensitivity.
Earlier studies using the NHP model have demonstrated that multi-omics approaches can be effective in identifying putative, early arising markers of radiation exposure and related injury in blood plasma/serum [Citation19,Citation110,Citation196–210]. These omic-based NHP studies designed to assess the pathophysiological effects of acute irradiation included the use of advanced, state-of-the-art MS technology. Additional animal (rodent)-based work has been reported using comparable discovery designed approaches. However, as informative as these studies might be, they are limited in value by the fact that they do not address certain response variables, most notably the influence of gender. Further, there are no follow-up studies using large animal models in which the observed change(s) of interest, i.e. putative biomarkers, have been validated relative to the major endpoints of interest, namely survival, structure/function of primary radiosensitive organs, as well as the capacity of the biomarker to differentiate exposed from unexposed individuals within the ‘at risk’ population. Biomarkers tied to measures of effectiveness of most MCMs currently under study remain insufficiently validated as well. Still further, newly proposed NHP work to validate multi-omics biomarker panels in compliance with FDA guidelines remains to be conducted and/or reported. In brief, there is presently an acute shortage of effective and reliable biomarkers available to help in the identification of individuals at risk of developing acute radiation-induced illness. Hence, there is a critical need to develop and to have available (i.e. in the emergency medical kit for nuclear/radiological exposure contingencies) well-validated biomarkers of radiation exposure and related consequent injury. Regardless of how daunting this research and development may appear; the application of high-throughput techniques will most certainly aid and abet the identification and validation of reliable and efficient biomarkers for both detection and clinical management of at-risk populations in case of radiological/nuclear emergencies.
It is important to validate biomarkers in humans. The biomarker research community may launch clinical studies on cohorts of patients exposed to therapeutic doses of radiation, such as malignancies, inflammatory diseases, or other clinical conditions to test the biofluids (serum or urine samples) for markers identified in preclinical studies. Such studies with clinical doses of radiation may not be optimal for ARS situations but such studies will increase the confidence for success in this area. Finally, on a positive note, we point out that recent developments in the area of bioinformatics are highly encouraging, making the identification and validation of the radiation exposure targeted proteome quite feasible.
Article highlights
Biomarkers are important for assessing radiation exposure doses and also for medical countermeasure (MCM) dose conversions from animals to humans for regulatory approval following the US FDA Animal Rule.
Research and development of such MCMs includes identification of radiation injury biomarkers.
Fully FDA-qualified biomarker(s) of acute radiation injury is needed, and there is no such biomarker at this moment.
Recent studies using irradiated small and large animal models suggest that proteomic changes within biofluids may prove to be useful for the identification and validation of specific biomarkers for both radiation exposure and consequent injury.
High-throughput techniques have identified large numbers of potential biomarkers within the proteome that show promise as biomarkers for radiation exposure.
Some proteome biomarkers for MCMs have been demonstrated across various species including humans and appear promising in terms of identifying MCM-specific biomarkers.
Declaration of interest
The authors have no relevant affiliations or financial involvement with any organization or entity with a financial interest in or financial conflict with the subject matter or materials discussed in the manuscript. This includes employment, consultancies, honoraria, stock ownership or options, expert testimony, grants or patents received or pending, or royalties.
Reviewer disclosures
Peer reviewers on this manuscript have received an honorarium from Expert Review of Proteomics for their review work but have no other relevant financial relationships to disclose.
Author contributions
VK Singh and TM Seed performed literature searches, drafted the manuscript, revised, and finalized for publication. M Srivastava assisted in the collection and writing of methodology section.
Acknowledgments
The opinions or assertions contained herein are the private views of the authors and are not necessarily those of the Uniformed Services University of the Health Sciences, or the Department of Defense. The mention of specific therapeutic agents does not constitute endorsement by the U.S. Department of Defense, and trade names are used only for the purpose of clarification. We are thankful to Ms. Alana Carpenter for editing the manuscript and Mr. Stephen Y. Wise for literature searches. We apologize to those having contributed substantially to the topics discussed herein that we were unable to cite because of space constraints.
Additional information
Funding
References
- Gale RP, Armitage JO, Hashmi SK. Emergency response to radiological and nuclear accidents and incidents. Br J Haematol. 2021;192(6):968–972. doi: 10.1111/bjh.16138
- Gale RP, Baranov A. If the unlikely becomes likely: medical response to nuclear accidents. Bull At Sci. 2011;67(2):10–18. doi: 10.1177/0096340211399849
- Gusev IA, Guskova AK, Mettler FA. Medical management of radiation accidents. Boca Raton (FL): CRC Press; 2001.
- Goans RE, Holloway EC, Berger ME, et al. Early dose assessment following severe radiation accidents. Health Phys. 1997;72:513–518. doi: 10.1097/00004032-199704000-00001
- Gorin NC, Fliedner TM, Gourmelon P, et al. Consensus conference on European preparedness for haematological and other medical management of mass radiation accidents. Ann Hematol. 2006;85:671–679. doi: 10.1007/s00277-006-0153-x
- Ohnishi T. The disaster at Japan’s Fukushima-Daiichi nuclear power plant after the March 11, 2011 earthquake and tsunami, and the resulting spread of radioisotope contamination. Radiat Res. 2012;177:1–14. doi: 10.1667/RR2830.1
- Dainiak N, Waselenko JK, Armitage JO, et al. The hematologist and radiation casualties. Hematol Am Soc Hematol Educ Prog. 2003;2003:473–496. doi: 10.1182/asheducation-2003.1.473
- Waselenko JK, MacVittie TJ, Blakely WF, et al. Medical management of the acute radiation syndrome: recommendations of the Strategic National Stockpile radiation working group. Ann Intern Med. 2004;140:1037–1051. doi: 10.7326/0003-4819-140-12-200406150-00015
- Jargin SV. Chernobyl-related cancer and precancerous lesions: incidence increase vs. late diagnostics. Dose Resp. 2014;12:404–414. doi: 10.2203/dose-response.13-039.Jargin
- Williams JP, McBride WH. After the bomb drops: a new look at radiation-induced multiple organ dysfunction syndrome (MODS). Int J Radiat Biol. 2011;87(8):851–868. doi: 10.3109/09553002.2011.560996
- Ozasa K, Cullings HM, Ohishi W, et al. Epidemiological studies of atomic bomb radiation at the radiation effects research foundation. Int J Radiat Biol. 2019;95(7):879–891. doi: 10.1080/09553002.2019.1569778
- Singh VK, Newman VL, Romaine PL, et al. Use of biomarkers for assessing radiation injury and efficacy of countermeasures. Expert Rev Mol Diagn. 2016;16:65–81. doi: 10.1586/14737159.2016.1121102
- Singh VK, Simas M, Pollard H. Biomarkers for acute radiation syndrome: challenges for developing radiation countermeasures following animal rule. Expert Rev Mol Diagn. 2018;18(11):921–924. doi: 10.1080/14737159.2018.1533404
- Dainiak N. Hematologic consequences of exposure to ionizing radiation. Exp Hematol. 2002;30(6):513–528. doi: 10.1016/S0301-472X(02)00802-0
- Dainiak N. Rationale and recommendations for treatment of radiation injury with cytokines. Health Phys. 2010;98(6):838–842. doi: 10.1097/HP.0b013e3181b3fce5
- U.S. Food and Drug Administration. Guidance document: product development under the animal Rule. 2015 [cited 2022 Oct 20]. Available from: http://www.fda.gov/downloads/drugs/guidancecomplianceregulatoryinformation/guidances/ucm399217.pdf
- Deutsch EW, Omenn GS, Sun Z, et al. Advances and utility of the human plasma proteome. J Proteome Res. 2021;20(12):5241–5263. doi: 10.1021/acs.jproteome.1c00657
- Moaddel R, Ubaida-Mohien C, Tanaka T, et al. Proteomics in aging research: a roadmap to clinical, translational research. Aging Cell. 2021;20(4):e13325. doi: 10.1111/acel.13325
- Cheema AK, Li Y, Moulton J, et al. Identification of novel biomarkers for acute radiation injury using multiomics approach and nonhuman primate model. Int J Radiat Oncol Biol Phys. 2022;114:310–320. doi: 10.1016/j.ijrobp.2022.05.046
- Carpenter AD, Li Y, Janocha BL, et al. Analysis of the proteomic profile in serum of irradiated nonhuman primates treated with Ex-Rad, a radiation medical countermeasure. J Proteome Res. 2023;22(4):1116–1126. doi: 10.1021/acs.jproteome.2c00458
- Girgis M, Li Y, Ma J, et al. Comparative proteomic analysis of serum from nonhuman primates administered BIO 300: a promising radiation countermeasure. Sci Rep. 2020;10(1):19343. doi: 10.1038/s41598-020-76494-4
- Aslam B, Basit M, Nisar MA, et al. Proteomics: technologies and their applications. J Chromatogr Sci. 2017;55(2):182–196. doi: 10.1093/chromsci/bmw167
- Gygi SP, Rochon Y, Franza BR, et al. Correlation between protein and mRNA abundance in yeast. Mol Cell Biol. 1999;19(3):1720–1730. doi: 10.1128/MCB.19.3.1720
- Kuster B, Schirle M, Mallick P, et al. Scoring proteomes with proteotypic peptide probes. Nat Rev Mol Cell Biol. 2005;6(7):577–583. doi: 10.1038/nrm1683
- Domon B, Aebersold R. Mass spectrometry and protein analysis. Science. 2006;312(5771):212–217. doi: 10.1126/science.1124619
- Zhu H, Snyder M. Protein arrays and microarrays. Curr Opin Chem Biol. 2001;5(1):40–45. doi: 10.1016/S1367-5931(00)00170-8
- Gavin AC, Maeda K, Kuhner S. Recent advances in charting protein-protein interaction: mass spectrometry-based approaches. Curr Opin Biotechnol. 2011;22:42–49. doi: 10.1016/j.copbio.2010.09.007
- Song YL, Johnson CB. Western blotting: methods and application. Methods Mol Biol. 2009;528:23–44.
- Hage DS, Anguizola JA, Bi C, et al. Pharmaceutical and biomedical applications of affinity chromatography: recent trends and developments. J Pharm Biomed Anal. 2012;69:93–105. doi: 10.1016/j.jpba.2012.01.004
- Block H, Maertens B, Spriestersbach A, et al. Immobilized-metal affinity chromatography (IMAC): a review. Methods Enzymol. 2014;463:439–73. doi: 10.1016/S0076-6879(09)63027-5
- Voedisch B, Thie H. Size exclusion chromatography. In: Kontermann R Dübel S, editors. Antibody engineering. Berlin (Heildberg): Springer; 2010. p. 607–612. doi: 10.1007/978-3-642-01144-3_38
- Some D, Amartely H, Tsadok A, et al. Characterization of proteins by size-exclusion chromatography coupled to multi-angle light scattering (SEC-MALS). J Vis Exp. 2019;148(148):| e59615. doi: 10.3791/59615
- Jungbauer A, Hahn R. Ion-exchange chromatography. Methods Enzymol. 2009;463:349–371.
- Abdulrahman A, Ghanem A. Recent advances in chromatographic purification of plasmid DNA for gene therapy and DNA vaccines: a review. Anal Chim Acta. 2018;1025:41–57. doi: 10.1016/j.aca.2018.04.001
- Hayrapetyan H, Tran T, Tellez-Corrales E, et al. Enzyme-linked immunosorbent assay: types and applications. Methods Mol Biol. 2023;2612:1–17.
- Singh VK, Wise SY, Scott JR, et al. Radioprotective efficacy of delta-tocotrienol, a vitamin E isoform, is mediated through granulocyte colony-stimulating factor. Life Sci. 2014;98(2):113–122. doi: 10.1016/j.lfs.2014.01.065
- Chowdhury F, Williams A, Johnson P. Validation and comparison of two multiplex technologies, Luminex and mesoscale discovery, for human cytokine profiling. J Immunol Methods. 2009;340:55–64. doi: 10.1016/j.jim.2008.10.002
- Raffield LM, Dang H, Pratte KA, et al. Comparison of proteomic assessment methods in multiple cohort studies. Proteomics. 2020;20(12):e1900278. doi: 10.1002/pmic.201900278
- Timms JF, Cramer R. Difference gel electrophoresis. Proteomics. 2008;8(23–24):4886–4897. doi: 10.1002/pmic.200800298
- Ohlendieck K. Comparative DIGE proteomics. Methods Mol Biol. 2018;1664:17–24.
- Arentz G, Weiland F, Oehler MK, et al. State of the art of 2D DIGE. Proteomics Clin Appl. 2015;9(3–4):277–288. doi: 10.1002/prca.201400119
- Michalski A, Cox J, Mann M. More than 100,000 detectable peptide species elute in single shotgun proteomics runs but the majority is inaccessible to data-dependent LC-MS/MS. J Proteome Res. 2011;10:1785–1793. doi: 10.1021/pr101060v
- Bantscheff M, Schirle M, Sweetman G, et al. Quantitative mass spectrometry in proteomics: a critical review. Anal Bioanal Chem. 2007;389(4):1017–1031. doi: 10.1007/s00216-007-1486-6
- Lundberg M, Thorsen SB, Assarsson E, et al. Multiplexed homogeneous proximity ligation assays for high-throughput protein biomarker research in serological material. Mol & Cell Proteomics. 2011;10(4):M110 004978. doi: 10.1074/mcp.M110.004978
- Ong SE, Blagoev B, Kratchmarova I, et al. Stable isotope labeling by amino acids in cell culture, SILAC, as a simple and accurate approach to expression proteomics. Mol Cell Proteomics. 2002;1(5):376–386. doi: 10.1074/mcp.M200025-MCP200
- Collins BC, Hunter CL, Liu Y, et al. Multi-laboratory assessment of reproducibility, qualitative and quantitative performance of SWATH-mass spectrometry. Nat Commun. 2017;8(1):291. doi: 10.1038/s41467-017-00249-5
- Egertson JD, Kuehn A, Merrihew GE, et al. Multiplexed MS/MS for improved data-independent acquisition. Nat Methods. 2013;10(8):744–746. doi: 10.1038/nmeth.2528
- Peterson AC, Russell JD, Bailey DJ, et al. Parallel reaction monitoring for high resolution and high mass accuracy quantitative, targeted proteomics. Mol & Cell Proteomics. 2012;11(11):1475–1488. doi: 10.1074/mcp.O112.020131
- Specht H, Slavov N. Transformative opportunities for single-cell proteomics. J Proteome Res. 2018;17(8):2565–2571. doi: 10.1021/acs.jproteome.8b00257
- Wang W, Zhou H, Lin H, et al. Quantification of proteins and metabolites by mass spectrometry without isotopic labeling or spiked standards. Anal Chem. 2003;75(18):4818–4826. doi: 10.1021/ac026468x
- Gillet LC, Navarro P, Tate S, et al. Targeted data extraction of the MS/MS spectra generated by data-independent acquisition: a new concept for consistent and accurate proteome analysis. Mol & Cell Proteomics. 2012;11(6):O111 016717. doi: 10.1074/mcp.O111.016717
- Liotta LA, Kohn EC, Petricoin EF. Clinical proteomics: personalized molecular medicine. JAMA. 2001;286(18):2211–2214. doi: 10.1001/jama.286.18.2211
- Ramachandran N, Hainsworth E, Bhullar B, et al. Self-assembling protein microarrays. Science. 2004;305(5680):86–90. doi: 10.1126/science.1097639
- Zhao L, Cong X, Zhai L, et al. Comparative evaluation of label-free quantification strategies. J Proteomics. 2020;215:103669. doi: 10.1016/j.jprot.2020.103669
- Pappireddi N, Martin L, Wuhr M. A review on quantitative Multiplexed proteomics. Chembiochem. 2019;20(10):1210–1224. doi: 10.1002/cbic.201800650
- Thompson A, Schafer J, Kuhn K, et al. Tandem mass tags: a novel quantification strategy for comparative analysis of complex protein mixtures by MS/MS. Anal Chem. 2003;75(8):1895–1904. doi: 10.1021/ac0262560
- Ross PL, Huang YN, Marchese JN, et al. Multiplexed protein quantitation in Saccharomyces cerevisiae using amine-reactive isobaric tagging reagents. Mol & Cell Proteomics. 2004;3(12):1154–1169. doi: 10.1074/mcp.M400129-MCP200
- Gygi SP, Rist B, Gerber SA, et al. Quantitative analysis of complex protein mixtures using isotope-coded affinity tags. Nat Biotechnol. 1999;17(10):994–999. doi: 10.1038/13690
- Deng J, Erdjument-Bromage H, Neubert TA. Quantitative comparison of proteomes using SILAC. Curr Protoc Protein Sci. 2019;95:e74. doi: 10.1002/cpps.74
- Lualdi M, Fasano M. Statistical analysis of proteomics data: a review on feature selection. J Proteomics. 2019;198:18–26. doi: 10.1016/j.jprot.2018.12.004
- Vihinen M. Bioinformatics in proteomics. Biomol Eng. 2001;18(5):241–248. doi: 10.1016/S1389-0344(01)00099-5
- MacDougall A, Volynkin V, Saidi R, et al. UniRule: a unified rule resource for automatic annotation in the UniProt knowledgebase. Bioinformatics. 2021;36(22–23):5562. doi: 10.1093/bioinformatics/btaa663
- Palomba A, Abbondio M, Fiorito G, et al. Comparative evaluation of MaxQuant and proteome discoverer MS1-based protein quantification tools. J Proteome Res. 2021;20(7):3497–3507. doi: 10.1021/acs.jproteome.1c00143
- Perez-Riverol Y, Csordas A, Bai J, et al. The PRIDE database and related tools and resources in 2019: improving support for quantification data. Nucleic Acids Res. 2019;47:D442–D50. doi: 10.1093/nar/gky1106
- MacLean B, Tomazela DM, Shulman N, et al. Skyline: an open source document editor for creating and analyzing targeted proteomics experiments. Bioinformatics. 2010;26(7):966–968. doi: 10.1093/bioinformatics/btq054
- Mi H, Muruganujan A, Ebert D, et al. PANTHER version 14: more genomes, a new PANTHER GO-slim and improvements in enrichment analysis tools. Nucleic Acids Res. 2019;47:D419–D26. doi: 10.1093/nar/gky1038
- Ashburner M, Ball CA, Blake JA, et al. Gene ontology: tool for the unification of biology. The gene ontology Consortium. Nat Genet. 2000;25(1):25–29. doi: 10.1038/75556
- Huang da W, Sherman BT, Lempicki RA. Systematic and integrative analysis of large gene lists using DAVID bioinformatics resources. Nat Protoc. 2009;4:44–57. doi: 10.1038/nprot.2008.211
- Shakyawar SK, Mishra NK, Vellichirammal NN, et al. A review of radiation-induced alterations of multi-omic profiles, radiation injury biomarkers, and countermeasures. Radiat Res. 2023;199: doi: 10.1667/RADE-21-00187.1
- Singh VK, Newman VL, Seed TM. Colony-stimulating factors for the treatment of the hematopoietic component of the acute radiation syndrome (H-ARS): a review. Cytokine. 2015;71(1):22–37. doi: 10.1016/j.cyto.2014.08.003
- Singh VK, Yadav VS. Role of cytokines and growth factors in radioprotection. Exp Mol Pathol. 2005;78(2):156–169. doi: 10.1016/j.yexmp.2004.10.003
- Ossetrova NI, Blakely WF, Nagy V, et al. Non-human primate total-body irradiation model with limited and full medical supportive care including filgrastim for biodosimetry and injury assessment. Radiat Prot Dosimetry. 2016;172(1–3):174–191. doi: 10.1093/rpd/ncw176
- Singh VK, Fatanmi OO, Singh PK, et al. Role of radiation-induced granulocyte colony-stimulating factor in recovery from whole body gamma-irradiation. Cytokine. 2012;58(3):406–414. doi: 10.1016/j.cyto.2012.03.011
- Singh VK, Seed TM. Radiation countermeasures for hematopoietic acute radiation syndrome: growth factors, cytokines and beyond. Int J Radiat Biol. 2021;97:1526–1547.
- Farese AM, MacVittie TJ. Filgrastim for the treatment of hematopoietic acute radiation syndrome. Drugs Today (Barc). 2015;51:537–548. doi: 10.1358/dot.2015.51.9.2386730
- Hankey KG, Farese AM, Blaauw EC, et al. Pegfilgrastim improves survival of lethally irradiated nonhuman primates. Radiat Res. 2015;183(6):643–655. doi: 10.1667/RR13940.1
- Farese AM, Cohen MV, Katz BP, et al. Filgrastim improves survival in lethally irradiated nonhuman primates. Radiat Res. 2013;179(1):89–100. doi: 10.1667/RR3049.1
- Zhong Y, Pouliot M, Downey AM, et al. Efficacy of delayed administration of sargramostim up to 120 hours post exposure in a nonhuman primate total body radiation model. Int J Radiat Biol. 2021;97:S100–S16. doi: 10.1080/09553002.2019.1673499
- Wong K, Chang PY, Fielden M, et al. Pharmacodynamics of romiplostim alone and in combination with pegfilgrastim on acute radiation-induced thrombocytopenia and neutropenia in non-human primates. Int J Radiat Biol. 2020;96(1):155–166. doi: 10.1080/09553002.2019.1625488
- Clayton NP, Khan-Malek RC, Dangler CA, et al. Sargramostim (rhu GM-CSF) improves survival of non-human primates with severe bone marrow suppression after acute, high-dose, whole-body irradiation. Radiat Res. 2021;195:191–199. doi: 10.1667/RADE-20-00131.1
- Bunin DI, Javitz HS, Gahagen J, et al. Survival and hematologic benefits of romiplostim after acute radiation exposure supported FDA approval under the animal rule. Int J Radiat Oncol Biol Phys. 2023;117(3):705–717. in press. doi: 10.1016/j.ijrobp.2023.05.008
- Shakhov AN, Singh VK, Bone F, et al. Prevention and mitigation of acute radiation syndrome in mice by synthetic lipopeptide agonists of Toll-like receptor 2 (TLR2). PLoS One. 2012;7(3):e33044. doi: 10.1371/journal.pone.0033044
- Singh VK, Christensen J, Fatanmi OO, et al. Myeloid progenitors: a radiation countermeasure that is effective when initiated days after irradiation. Radiat Res. 2012;177(6):781–791. doi: 10.1667/RR2894.1
- Singh VK, Ducey EJ, Fatanmi OO, et al. CBLB613: a TLR 2/6 agonist, natural lipopeptide of Mycoplasma arginini, as a novel radiation countermeasure. Radiat Res. 2012;177(5):628–642. doi: 10.1667/RR2657.1
- Ossetrova NI, Stanton P, Krasnopolsky K, et al. Comparison of biodosimetry biomarkers for radiation dose and injury assessment after mixed-field (neutron and gamma) and pure gamma radiation in the mouse total-body irradiation model. Health Phys. 2018;115:743–759. doi: 10.1097/HP.0000000000000939
- Ossetrova NI, Stanton P, Krasnopolsky K, et al. Biomarkers for radiation biodosimetry and injury assessment after mixed-field (neutron and gamma) radiation in the mouse total-body irradiation model. Health Phys. 2018;115:727–742. doi: 10.1097/HP.0000000000000938
- Kiang JG, Smith JT, Hegge SR, et al. Circulating cytokine/chemokine concentrations respond to ionizing radiation doses but not radiation dose rates: granulocyte-colony stimulating factor and interleukin-18. Radiat Res. 2018;189(6):634–643. doi: 10.1667/RR14966.1
- Xiao M, Bolduc DL, Li X, et al. Urine interleukin-18 (IL-18) as a biomarker of total-body irradiation: a preliminary study in nonhuman primates. Radiat Res. 2017;188(3):325–334. doi: 10.1667/RR14768.1
- Gulani J, Koch A, Chappell MG, et al. Cercopithecine herpesvirus 9 (simian varicella virus) infection after total-body irradiation in a rhesus macaque (Macaca mulatta). Comp Med. 2016;66:150–153.
- Ossetrova NI, Sandgren DJ, Blakely WF. Protein biomarkers for enhancement of radiation dose and injury assessment in nonhuman primate total-body irradiation model. Radiat Prot Dosimetry. 2014;159(1–4):61–76. doi: 10.1093/rpd/ncu165
- Ossetrova NI, Condliffe DP, Ney PH, et al. Early-response biomarkers for assessment of radiation exposure in a mouse total-body irradiation model. Health Phys. 2014;106:772–786. doi: 10.1097/HP.0000000000000094
- Blakely WF, Sandgren DJ, Nagy V, et al. Further biodosimetry investigations using murine partial-body irradiation model. Radiat Prot Dosimetry. 2014;159(1–4):46–51. doi: 10.1093/rpd/ncu127
- Prasanna PG, Blakely WF, Bertho JM, et al. Synopsis of partial-body radiation diagnostic biomarkers and medical management of radiation injury workshop. Radiat Res. 2010;173:245–253. doi: 10.1667/RR1993.1
- Blakely WF, Ossetrova NI, Whitnall MH, et al. Multiple parameter radiation injury assessment using a nonhuman primate radiation model-biodosimetry applications. Health Phys. 2010;98:153–159. doi: 10.1097/HP.0b013e3181b0306d
- Ossetrova NI, Blakely WF. Multiple blood-proteins approach for early-response exposure assessment using an in vivo murine radiation model. Int J Radiat Biol. 2009;85(10):837–850. doi: 10.3109/09553000903154799
- Ha CT, Li X, Fu D, et al. Circulating IL-18 binding protein (IL-18BP) and IL-18 as dual biomarkers of total-body irradiation in mice. Radiat Res. 2016;185(4):375–383. doi: 10.1667/RR14238.1
- Li X, Cui W, Hull L, et al. IL-18 binding protein (IL-18BP) as a novel radiation countermeasure after radiation exposure in mice. Sci Rep. 2020;10(1):18674. doi: 10.1038/s41598-020-75675-5
- Ossetrova NI, Sandgren DJ, Gallego S, et al. Combined approach of hematological biomarkers and plasma protein SAA for improvement of radiation dose assessment triage in biodosimetry applications. Health Phys. 2010;98:204–208. doi: 10.1097/HP.0b013e3181abaabf
- Ossetrova NI, Ney PH, Condliffe DP, et al. Acute radiation syndrome severity score system in mouse total-body irradiation model. Health Phys. 2016;111:134–144. doi: 10.1097/HP.0000000000000499
- Bell BL, Vercellino J, Brodin NP, et al. Orthovoltage X-rays exhibit increased efficacy compared with γ-rays in preclinical irradiation. Cancer Res. 2022;82:2678–2691. doi: 10.1158/0008-5472.CAN-22-0656
- Ha CT, Li XH, Fu D, et al. Circulating interleukin-18 as a biomarker of total-body radiation exposure in mice, minipigs, and nonhuman primates (NHP). PLoS One. 2014;9(10):e109249. doi: 10.1371/journal.pone.0109249
- Singh VK, Fatanmi OO, Wise SY, et al. Determination of lethality curve for cobalt-60 gamma-radiation source in rhesus macaques using subject-based supportive care. Radiat Res. 2022;198:599–614. doi: 10.1667/RADE-22-00101.1
- Singh VK, Kulkarni S, Fatanmi OO, et al. Radioprotective efficacy of gamma-tocotrienol in nonhuman primates. Radiat Res. 2016;185:285–298. doi: 10.1667/RR14127.1
- Krivokrysenko VI, Shakhov AN, Singh VK, et al. Identification of granulocyte colony-stimulating factor and interleukin-6 as candidate biomarkers of CBLB502 efficacy as a medical radiation countermeasure. J Pharmacol Exp Ther. 2012;343:497–508. doi: 10.1124/jpet.112.196071
- Krivokrysenko VI, Toshkov IA, Gleiberman AS, et al. The Toll-like receptor 5 agonist Entolimod mitigates lethal acute radiation syndrome in non-human primates. PLoS One. 2015;10:e0135388. doi: 10.1371/journal.pone.0135388
- Singh VK, Carpenter AD, Janocha BL, et al. Radiosensitivity of rhesus nonhuman primates: consideration of sex, supportive care, body weight and age at time of exposure. Expert Opin Drug Discov. 2023;18:797–814. doi: 10.1080/17460441.2023.2205123
- Cui W, Hankey KG, Zhang P, et al. Identifying circulating and lung tissue cytokines associated with thoracic irradiation and AEOL 10150 treatment in a nonhuman primate model. Radiat Res. 2020;194(1):81. doi: 10.1667/RR14310.1
- Huang W, Yu J, Jones JW, et al. Proteomic evaluation of the acute radiation syndrome of the gastrointestinal tract in a murine total-body irradiation model. Health Phys. 2019;116:516–528. doi: 10.1097/HP.0000000000000951
- Huang W, Yu J, Jones JW, et al. Acute proteomic changes in the lung after WTLI in a mouse model: identification of potential initiating events for delayed effects of acute radiation exposure. Health Phys. 2019;116:503–515. doi: 10.1097/HP.0000000000000956
- Cheema AK, Byrum SD, Sharma NK, et al. Proteomic changes in mouse spleen after radiation-induced injury and its modulation by gamma-tocotrienol. Radiat Res. 2018;190(5):449–463. doi: 10.1667/RR15008.1
- Rosen E, Fatanmi OO, Wise SY, et al. Tocol prophylaxis for total-body irradiation: a proteomic analysis in murine model. Health Phys. 2020;119:12–20. doi: 10.1097/HP.0000000000001221
- Rosen E, Fatanmi OO, Wise SY, et al. Gamma-tocotrienol, a radiation countermeasure, reverses proteomic changes in serum following total-body gamma irradiation in mice. Sci Rep. 2022;12(1):3387. doi: 10.1038/s41598-022-07266-5
- Rithidech KN, Honikel L, Rieger R, et al. Protein-expression profiles in mouse blood-plasma following acute whole-body exposure to (137)Cs gamma rays. Int J Radiat Biol. 2009;85:432–447. doi: 10.1080/09553000902820390
- Sproull M, Shankavaram U, Camphausen K. Comparison of proteomic biodosimetry biomarkers across five different murine strains. Radiat Res. 2019;192(6):640–648. doi: 10.1667/RR15442.1
- Zhang J, Han X, Zhao Y, et al. Mouse serum protects against total body irradiation-induced hematopoietic system injury by improving the systemic environment after radiation. Free Radic Biol Med. 2019;131:382–392. doi: 10.1016/j.freeradbiomed.2018.12.021
- Yi L, Hu N, Mu H, et al. Identification of cofilin-1 and destrin as potential early-warning biomarkers for gamma radiation in mouse liver tissues. Health Phys. 2019;116:749–759. doi: 10.1097/HP.0000000000001012
- Jin H, Jeon S, Kang GY, et al. Identification of radiation response genes and proteins from mouse pulmonary tissues after high-dose per fraction irradiation of limited lung volumes. Int J Radiat Biol. 2017;93(2):184–193. doi: 10.1080/09553002.2017.1235297
- Kulkarni S, Koller A, Mani KM, et al. Identifying urinary and serum exosome biomarkers for radiation exposure using a data dependent acquisition and SWATH-MS combined workflow. Int J Radiat Oncol Biol Phys. 2016;96(3):566–577. doi: 10.1016/j.ijrobp.2016.06.008
- Shukla S, Shankavaram UT, Nguyen P, et al. Radiation-induced alteration of the brain proteome: understanding the role of the sirtuin 2 deacetylase in a murine model. J Proteome Res. 2015;14(10):4104–4117. doi: 10.1021/acs.jproteome.5b00083
- Lin RX, Zhao HB, Li CR, et al. Proteomic analysis of ionizing radiation-induced proteins at the subcellular level. J Proteome Res. 2009;8(1):390–399. doi: 10.1021/pr800699w
- Lee Y, Pujol Canadell M, Shuryak I, et al. Candidate protein markers for radiation biodosimetry in the hematopoietically humanized mouse model. Sci Rep. 2018;8(1):13557. doi: 10.1038/s41598-018-31740-8
- Sun JL, Li S, Lu X, et al. Identification of the differentially expressed protein biomarkers in rat blood plasma in response to gamma irradiation. Int J Radiat Biol. 2020;96(6):748–758. doi: 10.1080/09553002.2020.1739775
- Sharma M, Halligan BD, Wakim BT, et al. The urine proteome for radiation biodosimetry: effect of total body vs. local kidney irradiation. Health Phys. 2010;98:186–195. doi: 10.1097/HP.0b013e3181b17cbd
- Sproull M, Nishita D, Chang P, et al. Comparison of proteomic expression profiles after radiation exposure across four different species. Radiat Res. 2022;197(4):315–323. doi: 10.1667/RADE-21-00182.1
- Sproull M, Shankavaram U, Camphausen K. Novel murine biomarkers of radiation exposure using an aptamer-based proteomic technology. Front Pharmacol. 2021;12:633131. doi: 10.3389/fphar.2021.633131
- Sproull M, Kawai T, Krauze A, et al. Prediction of total-body and partial-body exposures to radiation using plasma proteomic expression profiles. Radiat Res. 2022;198(6):573–581. doi: 10.1667/RADE-22-00074.1
- Byrum SD, Burdine MS, Orr L, et al. Time- and radiation-dose dependent changes in the plasma proteome after total body irradiation of non-human primates: implications for biomarker selection. PLoS One. 2017;12(3):e0174771. doi: 10.1371/journal.pone.0174771
- Byrum SD, Burdine MS, Orr L, et al. A quantitative proteomic analysis of urine from gamma-irradiated nonhuman primates. J Proteomics Bioinform. 2016;9:005. doi: 10.4172/jpb.S10-005
- Huang W, Yu J, Liu T, et al. Proteomics of non-human primate plasma after partial-body radiation with minimal bone marrow sparing. Health Phys. 2020;119:621–632. doi: 10.1097/HP.0000000000001350
- Huang W, Yu J, Farese AM, et al. Acute proteomic changes in non-human primate kidney after partial-body radiation with minimal bone marrow sparing. Health Phys. 2021;121:345–351. doi: 10.1097/HP.0000000000001475
- Huang W, Yu J, Liu T, et al. Acute proteomic changes in lung after radiation: toward identifying initiating events of delayed effects of acute radiation exposure in non-human primate after partial body irradiation with minimal bone marrow sparing. Health Phys. 2021;121:384–394. doi: 10.1097/HP.0000000000001476
- Huang W, Yu J, Liu T, et al. Proteomic evaluation of the natural history of the acute radiation syndrome of the gastrointestinal tract in a non-human primate model of partial-body irradiation with minimal bone marrow sparing includes dysregulation of the retinoid pathway. Health Phys. 2020;119:604–620. doi: 10.1097/HP.0000000000001351
- Pannkuk EL, Fornace AJ Jr., Laiakis EC. Metabolomic applications in radiation biodosimetry: exploring radiation effects through small molecules. Int J Radiat Biol. 2017;93:1151–1176.
- Singh VK, Seed TM, Cheema AK. Metabolomics-based predictive biomarkers of radiation injury and countermeasure efficacy: Current status and future perspectives. Expert Rev Mol Diagn. 2021;21(7):641–654. doi: 10.1080/14737159.2021.1933448
- Zalesak-Kravec S, Huang W, Wang P, et al. Multi-omic analysis of non-human primate heart after partial-body radiation with minimal bone marrow sparing. Health Phys. 2021;121:352–371. doi: 10.1097/HP.0000000000001478
- Muller L, Huang W, Jones JW, et al. Complementary lipidomic, proteomic, and mass spectrometry imaging approach to the characterization of the acute effects of radiation in the non-human primate mesenteric lymph node after partial-body irradiation with minimal bone marrow sparing. Health Phys. 2021;121:372–383. doi: 10.1097/HP.0000000000001470
- Kulkarni S, Singh PK, Ghosh SP, et al. Granulocyte colony-stimulating factor antibody abrogates radioprotective efficacy of gamma-tocotrienol, a promising radiation countermeasure. Cytokine. 2013;62(2):278–285. doi: 10.1016/j.cyto.2013.03.009
- Singh VK, Hauer-Jensen M. Gamma-tocotrienol as a promising countermeasure for acute radiation syndrome: Current status. Int J Mol Sci. 2016;17:e663.
- Singh PK, Wise SY, Ducey EJ, et al. Radioprotective efficacy of tocopherol succinate is mediated through granulocyte-colony stimulating factor. Cytokine. 2011;56(2):411–421. doi: 10.1016/j.cyto.2011.08.016
- Singh VK, Brown DS, Kao TC. Alpha-tocopherol succinate protects mice from gamma-radiation by induction of granulocyte-colony stimulating factor. Int J Radiat Biol. 2010;86(1):12–21. doi: 10.3109/09553000903264515
- Burdelya LG, Krivokrysenko VI, Tallant TC, et al. An agonist of Toll-like receptor 5 has radioprotective activity in mouse and primate models. Science. 2008;320:226–230. doi: 10.1126/science.1154986
- Grace MB, Singh VK, Rhee JG, et al. 5-AED enhances survival of irradiated mice in a G-CSF-dependent manner, stimulates innate immune cell function, reduces radiation-induced DNA damage and induces genes that modulate cell cycle progression and apoptosis. J Radiat Res. 2012;53(6):840–853. doi: 10.1093/jrr/rrs060
- Singh VK, Shafran RL, Inal CE, et al. Effects of whole-body gamma irradiation and 5-androstenediol administration on serum G-CSF. Immunopharmacol Immunotoxicol. 2005;27:521–534. doi: 10.1080/08923970500416707
- Singh VK, Grace MB, Jacobsen KO, et al. Administration of 5-androstenediol to mice: pharmacokinetics and cytokine gene expression. Exp Mol Pathol. 2008;84:178–188. doi: 10.1016/j.yexmp.2007.12.001
- Singh VK, Newman VL, Berg AN, et al. Animal models for acute radiation syndrome drug discovery. Expert Opin Drug Discov. 2015;10(5):497–517. doi: 10.1517/17460441.2015.1023290
- Rittase WB, McCart EA, Muir JM, et al. Effects of captopril against radiation injuries in the Gottingen minipig model of hematopoietic-acute radiation syndrome. PLoS One. 2021;16:e0256208. doi: 10.1371/journal.pone.0256208
- Singh VK, Seed TM. Entolimod as a radiation countermeasure for acute radiation syndrome. Drug Discov Today. 2021;26(1):17–30. doi: 10.1016/j.drudis.2020.10.003
- Singh VK, Pollard HB. Patents for Toll-like receptor ligands as radiation countermeasures for acute radiation syndrome. Expert Opin Ther Pat. 2015;25(10):1085–1092. doi: 10.1517/13543776.2015.1064900
- Singh VK, Hanlon BK, Santiago PT, et al. A review of radiation countermeasures focusing on injury-specific medicinals and regulatory approval status: part III. Countermeasures under early stages of development along with ‘standard of care’ medicinal and procedures not requiring regulatory approval for use. Int J Radiat Biol. 2017;93(9):885–906. doi: 10.1080/09553002.2017.1332440
- Singh VK, Seed TM. BIO 300: a promising radiation countermeasure under advanced development for acute radiation syndrome and the delayed effects of acute radiation exposure. Expert Opin Investig Drugs. 2020;29(5):429–441. doi: 10.1080/13543784.2020.1757648
- Singh VK, Grace MB, Parekh VI, et al. Effects of genistein administration on cytokine induction in whole-body gamma irradiated mice. Int Immunopharmacol. 2009;9:1401–1410. doi: 10.1016/j.intimp.2009.08.012
- Baliarsingh S, Beg ZH, Ahmad J. The therapeutic impacts of tocotrienols in type 2 diabetic patients with hyperlipidemia. Atherosclerosis. 2005;182(2):367–374. doi: 10.1016/j.atherosclerosis.2005.02.020
- Qureshi AA, Burger WC, Peterson DM, et al. The structure of an inhibitor of cholesterol biosynthesis isolated from barley. J Biol Chem. 1986;261(23):10544–10550. doi: 10.1016/S0021-9258(18)67419-8
- Singh VK, Seed TM. Development of gamma-tocotrienol as a radiation medical countermeasure for the acute radiation syndrome: Current status and future perspectives. Expert Opin Investig Drugs. 2023;32(1):25–35. doi: 10.1080/13543784.2023.2169127
- Ghosh SP, Kulkarni S, Hieber K, et al. Gamma-tocotrienol, a tocol antioxidant as a potent radioprotector. Int J Radiat Biol. 2009;85(7):598–606. doi: 10.1080/09553000902985128.
- Kulkarni SS, Cary LH, Gambles K, et al. Gamma-tocotrienol, a radiation prophylaxis agent, induces high levels of granulocyte colony-stimulating factor. Int Immunopharmacol. 2012;14(4):495–503. doi: 10.1016/j.intimp.2012.09.001
- Singh VK, Wise SY, Fatanmi OO, et al. Progenitors mobilized by gamma-tocotrienol as an effective radiation countermeasure. PLoS One. 2014;9(11):e114078. doi: 10.1371/journal.pone.0114078
- Singh VK, Fatanmi OO, Verma A, et al. Progenitor cell mobilization by gamma-tocotrienol: a promising radiation countermeasure. Health Phys. 2016;111:85–92. doi: 10.1097/HP.0000000000000458
- Lee HT, Park SW, Kim M, et al. Interleukin-11 protects against renal ischemia and reperfusion injury. Am J Physiol Renal Physiol. 2012;303(8):F1216–24. doi: 10.1152/ajprenal.00220.2012
- Kumar VP, Biswas S, Sharma NK, et al. Pegylated IL-11 (BBT-059): a novel radiation countermeasure for hematopoietic acute radiation syndrome. Health Phys. 2018;115:65–76. doi: 10.1097/HP.0000000000000841
- Cwirla SE, Balasubramanian P, Duffin DJ, et al. Peptide agonist of the thrombopoietin receptor as potent as the natural cytokine. Science. 1997;276(5319):1696–1699. doi: 10.1126/science.276.5319.1696
- Kumar VP, Holmes-Hampton GP, Biswas S, et al. Mitigation of total body irradiation-induced mortality and hematopoietic injury of mice by a thrombopoietin mimetic (JNJ-26366821). Sci Rep. 2022;12(1):3485. doi: 10.1038/s41598-022-07426-7
- Ofir R. Prophylactic administration of placenta-derived PLX-R18 stromal cells mitigates H-ARS death and promotes BM and peripheral blood lineage recovery in a murine model. In: Radiation injury treatment network. Washington DC (USA); 2019.
- Kumar VP, Biswas S, Holmes-Hampton GP, et al. Pre-administration of PLX-R18 cells protects mice from radiation-induced hematopoietic failure and lethality. Genes (Basel). 2022;13(10):1756. doi: 10.3390/genes13101756
- Singh VK, Brown DS, Kao TC. Tocopherol succinate: a promising radiation countermeasure. Int Immunopharmacol. 2009;9(12):1423–1430. doi: 10.1016/j.intimp.2009.08.020
- Singh VK, Parekh VI, Brown DS, et al. Tocopherol succinate: modulation of antioxidant enzymes and oncogene expression, and hematopoietic recovery. Int J Radiat Oncol Biol Phys. 2011;79:571–578. doi: 10.1016/j.ijrobp.2010.08.019
- Singh VK, Singh PK, Wise SY, et al. Radioprotective properties of tocopherol succinate against ionizing radiation in mice. J Radiat Res. 2013;54(2):210–220. doi: 10.1093/jrr/rrs088
- Singh VK, Romaine PL, Newman VL, et al. Tocols induce G-CSF and mobilise progenitors that mitigate radiation injury. Radiat Prot Dosimetry. 2014;162(1–2):83–87. doi: 10.1093/rpd/ncu223
- Barshishat-Kupper M, Mungunsukh O, Tipton AJ, et al. Captopril modulates hypoxia-inducible factors and erythropoietin responses in a murine model of total body irradiation. Exp Hematol. 2011;39(3):293–304. doi: 10.1016/j.exphem.2010.12.002
- Orrell RW. AEOL-10150 (aeolus). Curr Opin Invest Drugs. 2006;7(1):70–80.
- Singh VK, Olabisi AO. Nonhuman primates as models for the discovery and development of radiation countermeasures. Expert Opin Drug Discov. 2017;12:695–709.
- U.S. Food and Drug Administration. Animal Rule information. 2022. [cited 2022 Oct 20]. Available from: http://www.fda.gov/EmergencyPreparedness/Counterterrorism/MedicalCountermeasures/MCMRegulatoryScience/ucm391604.htm
- Singh VK, Beattie LA, Seed TM. Vitamin E: tocopherols and tocotrienols as potential radiation countermeasures. J Radiat Res. 2013;54:973–988. doi: 10.1093/jrr/rrt048
- Bajaj S, Alam SI, Ahmad B, et al. Combination of podophyllotoxin and rutin modulate radiation induced alterations of jejunal proteome in mice. Int J Radiat Biol. 2020;96(7):1–39. doi: 10.1080/09553002.2020.1741721
- Wang Q, Wang Y, Du L, et al. Quantitative proteomic analysis of the effects of melatonin treatment for mice suffered from small intestinal damage induced by gamma-ray radiation. Int J Radiat Biol. 2021;97:1206–1216. doi: 10.1080/09553002.2021.1956006
- Singh VK, Garcia M, Seed TM. A review of radiation countermeasures focusing on injury-specific medicinals and regulatory approval status: part II. Countermeasures for limited indications, internalized radionuclides, emesis, late effects, and agents demonstrating efficacy in large animals with or without FDA IND status. Int J Radiat Biol. 2017;93(9):870–884. doi: 10.1080/09553002.2017.1338782
- Singh VK, Newman VL, Romaine PL, et al. Radiation countermeasure agents: an update (2011 - 2014). Expert Opin Ther Pat. 2014;24:1229–1255. doi: 10.1517/13543776.2014.964684
- Kang AD, Cosenza SC, Bonagura M, et al. ON01210.Na (Ex-RAD(R)) mitigates radiation damage through activation of the AKT pathway. PLoS One. 2013;8:e58355. doi: 10.1371/journal.pone.0058355
- Suman S, Datta K, Doiron K, et al. Radioprotective effects of on 01210.Na upon oral administration. J Radiat Res. 2012;53:368–376. doi: 10.1269/jrr.11191
- Suman S, Maniar M, Fornace AJ Jr., et al. Administration of on 01210.Na after exposure to ionizing radiation protects bone marrow cells by attenuating DNA damage response. Radiat Oncol. 2012;7:6. doi: 10.1186/1748-717X-7-6
- Singh VK, Fatanmi OO, Wise SY, et al. A novel oral formulation of BIO 300 confers prophylactic radioprotection from acute radiation syndrome in mice. Int J Radiat Biol. 2022;98(5):958–967. doi: 10.1080/09553002.2021.1981556
- Singh VK, Serebrenik AA, Fatanmi OO, et al. The radioprotectant, BIO 300, protects the lungs from total-body irradiation injury in C57L/J mice. Radiat Res. 2023;199(3):294–300. doi: 10.1667/RADE-22-00142.1
- Landauer MR, Srinivasan V, Seed TM. Genistein treatment protects mice from ionizing radiation injury. J Appl Toxicol. 2003;23(6):379–385. doi: 10.1002/jat.904
- Davis TA, Clarke TK, Mog SR, et al. Subcutaneous administration of genistein prior to lethal irradiation supports multilineage, hematopoietic progenitor cell recovery and survival. Int J Radiat Biol. 2007;83(3):141–151. doi: 10.1080/09553000601132642
- Li Y, Girgis M, Jayatilake M, et al. Pharmacokinetic and metabolomic studies with a BIO 300 oral powder formulation in nonhuman primates. Sci Rep. 2022;12(1):13475. doi: 10.1038/s41598-022-17807-7
- Cheema AK, Mehta KY, Santiago PT, et al. Pharmacokinetic and metabolomic studies with BIO 300, a nanosuspension of genistein, in a nonhuman primate model. Int J Mol Sci. 2019;20:1231. doi: 10.3390/ijms20051231.
- Laiakis EC, Pinheiro M, Nguyen T, et al. Quantitative proteomic analytic approaches to identify metabolic changes in the medial prefrontal cortex of rats exposed to space radiation. Front Physiol. 2022;13:971282. doi: 10.3389/fphys.2022.971282
- Mo W, Xu W, Hong M, et al. Proteomic and miRNA profiling of radon-induced skin damage in mice: FASN regulated by miRnas. J Radiat Res. 2022;63(5):706–718. doi: 10.1093/jrr/rrac037
- Langen B, Vorontsov E, Spetz J, et al. Age and sex effects across the blood proteome after ionizing radiation exposure can bias biomarker screening and risk assessment. Sci Rep. 2022;12(1):7000. doi: 10.1038/s41598-022-10271-3
- Rithidech KN, Tungjai M, Jangiam W, et al. Proteomic profiling of hematopoietic stem/progenitor cells after a whole body exposure of CBA/CaJ mice to titanium ((48)Ti) ions. Proteomes. 2015;3:132–159. doi: 10.3390/proteomes3030132
- Li H, He Y, Di C, et al. Comparative analysis of the serum proteome for biomarker discovery to reveal hepatotoxicity induced by iron ion radiation in mice. Life Sci. 2016;167:57–66. doi: 10.1016/j.lfs.2016.10.029
- Biron DG, Loxdale HD, Ponton F, et al. Population proteomics: an emerging discipline to study metapopulation ecology. Proteomics. 2006;6(6):1712–1715. doi: 10.1002/pmic.200500423
- Nedelkov D, Kiernan UA, Niederkofler EE, et al. Population proteomics: the concept, attributes, and potential for cancer biomarker research. Mol & Cell Proteomics. 2006;5(10):1811–1818. doi: 10.1074/mcp.R600006-MCP200
- Bian Y, Gao C, Kuster B. On the potential of micro-flow LC-MS/MS in proteomics. Expert Rev Proteomics. 2022;19(3):153–164. doi: 10.1080/14789450.2022.2134780
- Lenco J, Vajrychova M, Pimkova K, et al. Conventional-flow liquid chromatography-mass spectrometry for exploratory bottom-up proteomic analyses. Anal Chem. 2018;90:5381–5389. doi: 10.1021/acs.analchem.8b00525
- Pannkuk EL, Laiakis EC, Singh VK, et al. Lipidomic signatures of nonhuman primates with radiation-induced hematopoietic syndrome. Sci Rep. 2017;7:9777.
- Pannkuk EL, Laiakis EC, Fornace AJ Jr., et al. A metabolomic serum signature from nonhuman primates treated with a radiation countermeasure, gamma-tocotrienol, and exposed to ionizing radiation. Health Phys. 2018;115:3–11. doi: 10.1097/HP.0000000000000776
- Coy SL, Krylov EV, Schneider BB, et al. Detection of radiation-exposure biomarkers by differential mobility prefiltered mass spectrometry (DMS-MS). Int J Mass Spectrom. 2010;291:108–117. doi: 10.1016/j.ijms.2010.01.013
- Johnson CH, Patterson AD, Krausz KW, et al. Radiation metabolomics. 5. Identification of urinary biomarkers of ionizing radiation exposure in nonhuman primates by mass spectrometry-based metabolomics. Radiat Res. 2012;178(4):328–340. doi: 10.1667/RR2950.1
- Li HH, Tyburski JB, Wang YW, et al. Modulation of fatty acid and bile acid metabolism by peroxisome proliferator-activated receptor alpha protects against alcoholic liver disease. Alcohol Clin Exp Res. 2014;38:1520–1531. doi: 10.1111/acer.12424
- Johnson CH, Manna SK, Krausz KW, et al. Global metabolomics reveals urinary biomarkers of breast cancer in a mcf-7 xenograft mouse model. Metabolites. 2013;3(3):658–672. doi: 10.3390/metabo3030658
- Wang C, Yang J, Nie J. Plasma phospholipid metabolic profiling and biomarkers of rats following radiation exposure based on liquid chromatography-mass spectrometry technique. Biomed Chromatogr. 2009;23(10):1079–1085. doi: 10.1002/bmc.1226
- Zhang Y, Zhou X, Li C, et al. Assessment of early triage for acute radiation injury in rat model based on urinary amino acid target analysis. Mol Biosyst. 2014;10(6):1441–1449. doi: 10.1039/C3MB70526A
- Liu H, Wang Z, Zhang X, et al. Selection of candidate radiation biomarkers in the serum of rats exposed to gamma-rays by GC/TOFMS-based metabolomics. Radiat Prot Dosimetry. 2013;154(1):9–17. doi: 10.1093/rpd/ncs138
- Golla S, Golla JP, Krausz KW, et al. Metabolomic analysis of mice exposed to gamma radiation reveals a systemic understanding of total-body exposure. Radiat Res. 2017;187(5):612–629. doi: 10.1667/RR14592.1
- Coy SL, Cheema AK, Tyburski JB, et al. Radiation metabolomics and its potential in biodosimetry. Int J Radiat Biol. 2011;87(8):802–823. doi: 10.3109/09553002.2011.556177
- Tyburski JB, Patterson AD, Krausz KW, et al. Radiation metabolomics. 2. Dose- and time-dependent urinary excretion of deaminated purines and pyrimidines after sublethal gamma-radiation exposure in mice. Radiat Res. 2009;172(1):42–57. doi: 10.1667/RR1703.1
- Mak TD, Tyburski JB, Krausz KW, et al. Exposure to ionizing radiation reveals global dose- and time-dependent changes in the urinary metabolome of rat. Metabolomics. 2015;11(5):1082–1094. doi: 10.1007/s11306-014-0765-4
- Dissmore T, DeMarco AG, Jayatilake M, et al. Longitudinal metabolic alterations in plasma of rats exposed to low doses of high linear energy transfer radiation. J Environ Sci Health C Toxicol Carcinog. 2021;39:219–233. doi: 10.1080/26896583.2020.1865027
- Cheema AK, Mehta KY, Fatanmi OO, et al. A metabolomic and lipidomic serum signature from nonhuman primates administered with a promising radiation countermeasure, gamma-tocotrienol. Int J Mol Sci. 2018;19:79. doi: 10.3390/ijms19010079