ABSTRACT
Toxicological studies were performed to examine silver nanoparticle (AgNP, size: 14.4 ± 2.5 nm) transformation within three different test media and consequent effects on embryos of whitefish (Coregonus lavaretus) and roach (Rutilus rutilus). The test media, namely ASTM very hard water, ISO standard dilution medium, and natural lake water differed predominantly in ionic strength. Total silver was determined using inductively coupled plasma mass spectrometry (ICP-MS), while AgNPs were characterized by transmission electron microscopy and single particle ICP-MS. Silver species distributions were estimated via thermodynamic speciation calculations. Data demonstrated that increased AgNP dissolution accompanied by decreasing ionic strength of the test medium did not occur as noted in other studies. Further, other physicochemical parameters including AgNP size and metallic species distribution did not markedly affect AgNP-induced toxicity. Irrespective of the test medium, C. lavaretus were more sensitive to AgNP exposure (median lethal concentration after 8 weeks: 0.51–0.73 mg/L) compared to R. rutilus, where adverse effects were only observed at 5 mg/L in natural lake water. In addition, AgNP-induced toxicity was lower in the two standard test media compared to natural lake water. Currently, there are no apparent studies assessing simultaneously the sensitivity of C. lavaretus and R. rutilus to AgNP exposure. Therefore, the aim of this study was to (1) investigate AgNP-induced toxicity in C. lavaretus and R. rutilus cohabiting in the same aquatic environment and (2) the role played by test media in the observed effects of AgNPs on these aquatic species.
Introduction
Silver nanoparticles (AgNPs) are broadly applied in various products such as paints, food packaging, or textiles, predominantly attributed to antimicrobial properties (Kaweeteerawat et al. Citation2017; Monteiro et al. Citation2009). However, the wide range of applications inevitably resulted in a discharge of AgNPs into the environment (Lekamge et al. Citation2019; Nowack and Bucheli Citation2007). Measuring AgNPs in complex environmental samples is still a challenge. Several investigators reported concentrations of ≤1500 ng/L in WWTP influents (Li et al. Citation2013; Vogt et al. Citation2019) and a removal efficiency of WWTPs being ≥95% (Li et al. Citation2013, Citation2016). The release of AgNPs to aquatic environments is mainly accompanied by a rapid sedimentation (Blaser et al. Citation2008; Stebounova, Guio, and Grassian Citation2010) resulting in a steady accumulation of AgNP or their transformation products in sediments, despite their low discharge rate (Gottschalk et al. Citation2009; Vogt et al. Citation2019).
Fish embryos of several species are in contact with sediments for their entire embryogenesis and may be exposed to AgNPs during several critical periods in their life cycle (Gottschalk, Sun, and Nowack Citation2013; Kamler Citation1992a). Embryos of fish species developing in potentially AgNP enriched sediments for extended durations, such as Coregonus lavaretus or Rutilus rutilus (developmental time approximately ≥2 month and 9 days, respectively; Cingi, Keinänen, and Vuorinen Citation2010; Kortet et al. Citation2004) are therefore at a higher risk compared to fish species with a short embryogenesis such as the most frequently used test organism zebrafish (Danio rerio; developmental time ≤ 3 days). These former species are, however, of high ecological relevance for temperate environments as C. lavaretus and Coregonus clupeaformis exhibit similar ecological niches and are widely distributed throughout northern Europe, northern USA, Canada, and Russia (Lelek Citation1987; Scott and Crossman Citation1973). Rutilus rutilus is commonly found in most parts of Europe and Asia (Kottelat and Freyhof Citation2007). Therefore, assessing the risk of AgNPs using fish species relevant for temperate environments is needed.
The dissolution of AgNPs to Ag+ is believed to be a major source of AgNP-mediated toxicity (Boyle and Goss Citation2018; Cunningham et al. Citation2013; Kim, Kim, and Lee Citation2011) and is dependent on particle specific factors such as size, shape, or coating (Cunningham et al. Citation2013; George et al. Citation2012; Ma et al. Citation2012), but also characteristics of the surrounding medium like pH (Liu and Hurt Citation2010), dissolved organic matter (Chappell et al. Citation2011; Zhang et al. Citation2009), dissolved oxygen (Liu and Hurt Citation2010), temperature (Kittler et al. Citation2010; Liu and Hurt Citation2010; Walters et al. Citation2016), or ionic strength (Hawkins et al. Citation2015; Metreveli et al. Citation2016; Stebounova, Guio, and Grassian Citation2010; Yang et al. Citation2012). Several investigators reported that an increased ionic strength of the surrounding test medium reduced thickness of the diffuse double layer of AgNPs, thus, promoting particle-particle interactions and finally AgNP agglomeration (Bae et al. Citation2013; Badawy et al. Citation2010; Chambers et al. Citation2014; Metreveli et al. Citation2016; Römer et al. Citation2013). An associated decrease in total surface area reduced dissolution of AgNPs and highly toxic Ag+ ions were released at a lower rate (Dobias and Bernier-Latmani Citation2013; Zook et al. Citation2012). At the same time, the presence of anions in the test medium including chloride, sulfide, or sulfate leads to the formation of thermodynamically favored silver salts with extremely low solubility products such as AgCl or Ag2S. These silver salts exhibit a lower toxicity compared to Ag+ and AgNPs (Levard et al. Citation2013). Therefore, the applied test medium is considered to be critical when estimating and comparing AgNP-induced effects in lab studies.
The aim of this study was to assess how the chemical composition of various test media influenced AgNP toxicity for embryos of C. lavaretus and R. rutilus as evidenced by mortality, developmental delay, and deformations during embryogenesis. Two standard test media recommended for toxicological studies ASTM very hard water and ISO standard dilution medium (ASTM Citation2007; ISO Citation1992) were employed. In addition, natural water from a subalpine lake (Lake Mondsee) was utilized as a third test medium differing predominantly in chemical composition and ionic strength. The interaction of AgNPs with C. lavaretus embryos in test media was monitored in a separate study in terms of size and potential AgNP dissolution over the course of 1 week using inductively coupled plasma mass spectrometry (ICP-MS), single-particle ICP-MS (spICP-MS) with a prototype data acqusition system that provides microsecond time resolution (Mozhayeva and Engelhard Citation2019, Citation2020; Strenge and Engelhard Citation2016) and transmission electron microscopy (TEM).
Materials and methods
Ethics statement
The Research Department for Limnology, Mondsee, is a registered cultivation and rearing facility for fish (BMWFW-66.008/0001-WF/V/3b/2017 and BMWFW-66.008/0009-WF/II/3b/2014). Cultivation and rearing of fish were in accordance with national legislations. All experiments were approved by the animal welfare committee of the University of Innsbruck (Austria).
Test species
R. rutilus and C. lavaretus were obtained from commercial catches during the spawning season from Lake Mondsee on May 3, 2016 and on November 24, 2016, respectively, using gill nets (C. lavaretus) and trap nets (R. rutilus). The fertilization of eggs was conducted on the same day. For C. lavaretus, eggs from eight females and milt from five males were used, whereas for R. rutilus, eggs from four females were fertilized with milt from six males. Eggs and milt were only taken from adult fish showing no signs of diseases. Eggs of apparently low quality were not employed for the test. The adult fish handling and fertilization processes were conducted according to the national animal welfare legislation and followed established aquaculture practices (Wanzenböck CitationIn press).
Test media
Three different test media were utilized, namely ASTM very hard water (ASTM; ASTM Citation2007; Mg2+ 48.5 mg/L, Ca2+ 55.9 mg/L, Na+ 105.1 mg/L, K+ 8.4 mg/L, SO42- 325.5 mg/L, Cl− 7.7 mg/L), ISO standard dilution medium (ISO; ISO Citation1992; Mg2+ 12.2 mg/L, Ca2+ 80.2 mg/L, Na+ 17.2 mg/L, K+ 2.9 mg/L, SO42- 48.1 mg/L, Cl− 144.4 mg/L), and natural water from Lake Mondsee (LM; Mg2+ 8.9 mg/L, Ca2+ 55.2 mg/L, Na+ 6.7 mg/L, K+ 1.3 mg/L, NO3− 0.6 mg/L, SO42- 5.7 mg/L, Cl− 10.2 mg/L). The standard test media (ASTM hard water and ISO) are recommended by OECD for testing effects of chemicals on Daphnia and fish (OECD Citation1992, Citation2004, Citation2012). ASTM hard water and ISO possess a similar ionic strength; therefore, ASTM very hard water was selected to obtain different ionic strengths in all three test media (Table A1). ASTM and ISO were prepared using MilliQ water and the required chemicals (Table A 2). The pH of the test media was not adjusted.
Lake Mondsee water was pumped out of the lake at 12 m depth, filtered using a sand filter, and disinfected by UV radiation and ozone treatment. Finally, Lake Mondsee water was cleaned using micro-glass fiber filtration (MGC; pore size: 1.2 µm; Munktell & Filtrak GmbH, Bärenstein, Germany) followed by an additional disinfection step via heating utilizing a microwave (900 W for 7:30 min; R-93ST-AA, Sharp Electronics GmbH, Wien, Austria). These cleaning steps were accomplished to exclude biological factors in the test medium, such as microorganisms; and thus, effects of AgNPs might solely be related to the chemical composition of the test medium. The above-mentioned chemical composition of Lake Mondsee water in terms of Mg2+, Ca2+, Na+, K+, NO3−, SO42-, and Cl− was measured using ion chromatography (Dionex ICS-1100 RFIC system, Thermo Scientific Dionex, USA; Table A 2). Again, no pH adjustment was done. Lake Mondsee was part of an extensive field study during which the concentration of AgNP and total silver was determined in the lake (Vogt et al. Citation2019). Data demonstrated that AgNP concentrations in the lake water were below the limit of detection (LOD; Vogt et al. Citation2019). Each medium was prepared 1 day before use and saturated with oxygen by aeration overnight at 5.2 ± 0.3°C and 12.8 ± 1°C for C. lavaretus and R. rutilus, respectively.
AgNP characterization
AgNPs, namely NM-300 K, were provided by the Organization for Economic Co-operation and Development (OECD) Sponsorship Program. AgNPs featured a spherical shape with an average particles size (± standard deviation (SD) of the mean) of 15 ± 0.1 nm according to the manufacturer’s TEM data (for further information see Klein et al. Citation2011). The stabilization agent NM-300 K-DIS (4% polyoxyethylene glycerol trioleate and 4% polyoxyethylene (20) sorbitan mono-laurate) was an additional component of the AgNP stock dispersion (10.16% w/w AgNP) to avoid changes in the physicochemical state of AgNPs. Nanoparticles were extracted from their test medium via cloud point extraction as described by Hartmann, Hutterer, and Schuster (Citation2013). These solutions were placed into a centrifuge tube containing a custome made substrate which held a copper mesh grid in place at the bottom of the tube. AgNP were then seperated from the remaining solution via centrifugation. TEM images of the copper mesh grid were taken to verify the AgNP size using a Talos F200X (FEI, Hillsboro, USA) at 200 kV. The size of 591 primary particles was determined using ImageJ (V 1.50i), by converting their two dimensional projected surface area onto the images to ideal spherically shaped particles. Their mode ± SD of the particle size distribution function was calculated using Origin Pro (Version 9.0) by fitting a log-normal function resulting in an AgNP size of 14.4 ± 2.5 nm ().
Exposure of C. lavaretus and R. rutilus embryos
Exposure of C. lavaretus and R. rutilus mainly followed the OECD test guideline 236 (Citation2013) with some adaptations due to the sensitivity of C. lavaretus embryos. Newly fertilized eggs (age < 2 hr post fertilization) of both species were exposed to nominal AgNP concentrations of Control (0), 0.1, 0.5, 1, 2 or 5 mg/L. The concentrations were selected based upon a pretest using the same conditions. These concentrations were also within the range of ecotoxicological studies with fish (Cho et al. Citation2013; Laban et al. Citation2010; Muth-Köhne et al. Citation2013), thus enabling a direct comparison of C. lavaretus and R. rutilus with other fish species such as D. rerio, Pimephales promelas, and Oryzias latipes. To account for different developmental periods for embryogenesis of C. lavaretus and R. rutilus embryos, two exposure times to AgNPs were utilized (8 weeks and 9 days, respectively). The experimental duration was selected to ensure that the experiments ended shortly before hatching. This enabled determination of the presence of AgNPs in the chorion and fish embryos via optical dark field microscopy. The influence of the stabilization agent NM-300 K DIS was also tested in ASTM, ISO, and Lake Mondsee water to test for potential toxicological effects on embryos of both fish species using a concentration equal to the amount of NM-300 K DIS available in the highest tested AgNP concentration (5 mg/L AgNPs). All AgNP stock dispersions and NM-300 K DIS stock solutions were treated in an ultrasonic bath (Sonorex RK 102 Transistor, Bandelin electronic GmbH & Co. KG, Berlin, Germany) at 35 kHz for 10 min before usage.
C. lavaretus and R. rutilus embryos were exposed in new 24-well plates (CytoOne, untreated, polystyrene, Starlab International GmbH, Hamburg, Germany) filled with 3 mL respective test media in darkness at 5.2 ± 0.3 and 12.8 ± 1°C, respectively. All well plates were pre-conditioned for 24 hr using the corresponding test solution. Prior to transferring the newly fertilized eggs to the well plates, approximately 100 eggs per treatment were kept in petri dishes (material: polystyrene) filled with test solution containing the respective test medium and AgNPs/ NM-300 K DIS for a maximum of 4 hr to sort out over-, undersized, or deformed eggs. On each well plate, 20 wells were used for respective test solutions, whereas the remaining 4 wells were filled with the respective control medium (ASTM, ISO, or Lake Mondsee water) as an internal plate control as recommended by OECD test guideline 236 (Citation2013). One egg was transferred to each well using a plastic Pasteur pipette. The tests were conducted in a triplicate design, with one well plate representing one replicate (20 individuals per replicate).
The test medium was replaced weekly by exchanging 2/3 of the test solutions, thus ensuring that the highly sensitive eggs were fully covered by the test solution at all times. Prior to the exchange procedure, the test solutions were mixed in all well plates to ensure a homogenous distribution of AgNPs/ NM-300 K DIS within the well. New AgNP dispersions and NM-300 K DIS stock solutions were prepared for every medium change. On the day of medium exchange, pH and electrical conductivity were measured in freshly prepared control medium and in a collective control sample of the old medium to obtain sufficient volume (Hach HQ40d, Hach Lange GmbH, Vienna, Austria). Temperature and concentrations of dissolved oxygen were monitored every 10 min for the whole test duration using microfiber optic oxygen and temperature sensors (Oxy-4 ST, Precision Sensing GmbH, Regensburg, Germany). For each test medium, a random well plate was selected and both parameters were determined in one internal plate control that also contained a fish embryo. A new random well was selected after each medium exchange. Temperature, oxygen concentration, and pH were stable throughout the test, whereas electrical conductivity increased within 1 week (Table A 1). Since the ionic composition of Lake Mondsee water was not fully available, ionic strength was calculated from the electrical conductivity using the approximate calculation provided by Maier and Grohmann (Citation1977) for all test media, rather than using the exact calculation (OECD Citation2017; for comparison see Table A 3).
To calculate the median lethal concentration after 9 days (LC50-9d), R. rutilus embryos were observed daily for signs of lethality including coagulation and lack of heartbeat, as well as additional signs of lethality, including lack of somite formation and non-detachment of the tail (OECD Citation2013). In addition, adverse effects during embryogenesis such as edema or phenotypic defects of the head, tail, or yolk sac were tracked to calculate the median effect concentration (EC50-9day). Previous tests with daily observations of C. lavaretus embryos resulted in high control mortality (>80%), which might be attributed to high sensitivity of C. lavaretus to temperature changes during handling (Cingi, Keinänen, and Vuorinen Citation2010). To reduce stress, C. lavaretus embryos were observed weekly for 8 weeks for the above mentioned endpoints to calculate the LC50-8 week and EC50-8 week. During the time of eye-pigmentation, embryos of both species were monitored daily to precisely record the day of reaching the eye-point stage. This day was defined as a measure for developmental delay and may be determined accurately due to the transparent chorion of C. lavaretus and R. rutilus. All endpoints were analyzed using a dissecting microscope (Motic SMZ-168, Motic Deutschland GmbH, Wetzlar, Germany). Replicates were excluded from the analysis, if mortality exceeded 25% in the internal plate control as recommended by OECD test guideline 236 (Citation2013): one replicate of each ASTM 0.1 mg/L and 0.5 mg/L, ISO 2 mg/L, and Lake Mondsee water 0.5 mg/L.
AgNP uptake by embryos
Histological analyses of the whole eggs were conducted at the end of each test to evaluate whether AgNPs were able to pass the chorion. Whole eggs of each treatment were washed three times with phosphate buffered saline (PBS; pH 7.4) and stored in polypropylene tubes (Greiner Bio-One, Kremsmünster, Austria) filled with neutral buffered formalin solution (NBF; 4% formalin, 4 g/L NaH2PO4 · H2O, and 6.5 g/L Na2HPO4) at 4°C in darkness. Prior to histological analysis, all eggs were washed three times with NBF to remove excessive formaldehyde. Dehydration and infiltration of the samples was performed stepwise with (i) 30% (w/v) sucrose solution, (ii) a 1:1 mixture of 30% sucrose and frozen section medium (v/v; Richard-Allan Scientific™ Neg-50™, San Diego, USA), and finally (iii) in pure frozen section medium. Each step was performed overnight at 4°C. Liquid nitrogen-cooled 2,2-dimethylbutane (Sigma-Aldrich, Darmstadt, Germany) was employed for the final embedding. Sections of 5 to 10 µm were cut with a cryomicrotome (Leica Microsystems, CM1900, Nussloch, Germany) at −20°C and collected on adhesion microscope slides (Polysine, Thermo Fisher Scientific, Braunschweig, Germany). Residuals of the frozen section medium were removed, and sections mounted with DPX mounting medium (Merck, Darmstadt, Germany) and cover-slipped. Histological analysis was performed using a light microscope (Zeiss Axioskop, Jena, Germany) under darkfield illumination with a mercury vapor lamp (HBO 50 W, Osram, Berlin, Germany) as a light source.
Nanoparticle stability
Time-dependent AgNP concentrations and particle size changes were evaluated in a separate experiment at 0.1 mg/L (nominal concentration) after 0, 24, 96, and 168 hr for all three media. The same test conditions were applied as in the fish embryo test and well plates contained C. lavaretus embryos to also consider AgNP interactions with the embryos. The test lasted for 7 days and no medium exchange was performed. In order to avoid mortality and poor health conditions of fish embryos in the AgNP stability experiment, C. lavaretus embryos were raised under optimal culture conditions in Zuger jars to achieve optimal health conditions (Wanzenböck CitationIn press) and later used for this study at the eye-point stage. At this stage, the fish larvae surpassed several critical developmental stages (based on previous research at the Research Department for Limnology, Mondsee but also Kamler Citation1992a), thus ensuring comparable interactions of the embryos with AgNPs in each of the applied media. For MilliQ water, these measurements were only done after 0 hr without fish embryos and the AgNP size was additionally determined after 168 hr. The time point 0 hr for all media included the time elapsed during the preparation of the respective test medium (< 10 min). A concentration of 0.1 mg/L was selected, because higher concentrations would have required a dilution of the samples prior to the measurements, thus implementing an additional factor that might have biased the results. Twenty new 24-well plates were prepared for each test medium and collective samples from 2 mL aliquots were taken for each time point and test medium from as many wells as needed for the respective measurement. Each well was only used once for sampling and the volume and number of wells needed for each measurement are described below.
TEM measurements
The AgNP sizes of primary particles were measured by TEM as described earlier. For each medium and time point, 2 mL aliquots were collected from 20 wells into a polypropylene vessel (= 40 mL, n = 2), and frozen and stored at −20°C. Primary particles 108–591 were analyzed by TEM per treatment, irrespective of whether a particle was part of an agglomerate or present as an individual particle. As a consequence, a decrease in particle size over time indicates dissolution. Determining the size of agglomerates was not performed by TEM, because sample-preparation-induced particle agglomeration during the evaporation process of the solvent on the copper mesh grid could not be excluded. While storage effects (i.e. freezing after sampling and thawing before the measurements) may introduce analytical errors, transportation of samples from the culturing site was necessary and unavoidable.
spICP-MS measurements
The size of AgNPs in suspensions was assessed also via spICP-MS. Here, 2 mL aliquots were collected from five wells (= 10 mL, n = 1), frozen using liquid nitrogen and stored at −20°C using polypropylene cryo vials. A model iCAP Qc (Thermo Fisher Scientific, Bremen, Germany) ICP-MS instrument with a single quadrupole mass analyzer was used for spICP-MS measurements. In short, the data acquisition for spICP-MS was performed with a prototype DAQ (continuous 5 µs time resolution; Strenge and Engelhard Citation2016). After careful optimization (Mozhayeva and Engelhard Citation2017; Mozhayeva, Strenge, and Engelhard Citation2017), particle characterization was performed on a particle-by-particle level with a particle size LOD of 10.5 nm in the medium samples. Particle number LOD was set to 10 particles per measurement, according to Poisson statistics with false positives and false negatives probability set to 5% (Currie Citation1972). Data processing was performed by extracting nanoparticle ion cloud events from the raw data, as described by Strenge and Engelhard (Citation2016). Before spICP-MS measurement, water samples were thawed in the fridge at 4°C overnight. Prior to the analysis, samples were allowed to reach room temperature and shaken for 15 min. The samples were diluted with double distilled water immediately before spICP-MS measurements. The size calibration was done with 20, 40, and 60 nm citrate-coated AgNPs (Nanocomposix, San Diego, CA, USA) with the sizes taken from the specification sheets from the manufacturer´s TEM data. The experimental conditions are presented in Table A 4.
Dissolution-measurement
Potential dissolution of AgNPs was assessed in the nanoparticle stability study by applying centrifugal ultrafiltration (50 kDa; Amicon® Ultra-15, Sigma-Aldrich, Vienna, Austria) at 2934 g for 30 min (Eppendorf 5430 R, fixed angle rotor: Eppendorf F-35-6-30, Vienna, Austria), a technique that was used in several studies (Jung et al. Citation2018; Kreyling et al. Citation2014; Stefanic et al. Citation2019) to separate AgNPs or small particles of approximately <5–6 nm from their dissolved fraction. For each time point and medium, 2 mL aliquots were collected from five wells (= 10 mL; n = 1 for total silver and n = 2 for dissolved silver measurements) to assess total silver in the unfiltered samples and the filtrate and ultimately the amount of Ag+ released from AgNP. Total silver includes AgNP, all silver species (e.g. Ag+ and silver salts), and bulk silver. Both, the unfiltered samples and the filtrates were stabilized using HNO3 (69%, Suprapur®, Carl Roth, Germany) to reach pH 1–2, and frozen and stored in polypropylene vessels at −20°C.
Total silver concentration was then measured by ICP-MS to quantify the dissolution rate of AgNPs. The Ag+ recovery after filtration was verified at 10 and 50 µg/L Ag+ in MilliQ water using AgNO3. Ag+ in the test solutions was stabilized by using HNO3 (69%, Suprapur®, Carl Roth, Germany) to reach pH 1–2. Again, the total silver concentration was measured in the initial solution and the filtrate via ICP-MS to assess Ag+ recovery after filtration. Prior to each measurement, samples were gently thawed at 4°C for 2 days, taken out of the fridge, and shaken for 30 min.
The digestion procedure of AgNPs was conducted with nitric acid (70%, Analytical Reagent Grade, Fisher Scientific, Loughborough, UK) for 90 min. The digested samples were diluted 100 times to obtain the concentration that are compatible with the ICP-MS instrument (2.85% (w/v) HNO3). Samples that only contained Ag+ were analyzed without the digestion procedure after a 10-fold double distilled water dilution. The calibration of the instrument was conducted on the same day with a series of 7 silver standards from 10 ng/L to 10 µg/L in 2.5% (w/v) HNO3. The matrix-matched blank samples (2.5% (w/v) HNO3) were measured at least after each fifth sample. Indium was used as an internal standard to compensate for matrix interferences. Limits of detection (LOD; 3.29× SD of the blank (SDBL)) and limits of quantification (LOQ; 10× SDBL) for total silver in the samples (taking into the account the respective dilution) were determined to be 100.9 ng/L and 336.4 ng/L, respectively.
Speciation calculation
In order to estimate the distribution of thermodynamically expected Ag(I) species in media applied in this study, the speciation calculation was done for Ag using Visual MINTEQ software version 3.0 (Gustafsson Citation2017). The calculations were performed for Ag(I) concentration range of 0.5–1000 µg/L considering the CO2 equilibrium between air and aqueous phase. The presence of NM-300 K DIS in solutions was not considered. The calculations were conducted for input solutions containing only dissolved species without AgNPs (Table A 5). The activity coefficients were calculated by extended Debye-Hückel equation (Gustafsson Citation2017). The number of iterations for model calculations was set to 5000. The kinetics of Ag(I) species formation was not considered by model calculations. Interactions of silver species with organic metabolites, nitrogenous compounds, or potentially remaining NOM in the water of Lake Mondsee water after filtration were not incorporated into the model, as no measurements were done to determine their presence.
Statistical analysis
The software R, version 3.3.2 for Windows (R Core Team Citation2016) and respective extension packages (Ritz et al. Citation2015; Wickham Citation2009) were used for statistical analyses and dose-response modeling. LC50s and EC50s with corresponding 95% confidence intervals (CI) were calculated by fitting dose–response models and selecting the best-fitted model based on Akaike’s information criterion and visual judgment. Statistical difference was assumed when CIs did not overlap. Embryos being exposed to the stabilization agent NM-300 K DIS were not considered for LC50s and EC50s calculations. Statistical requirements for parametric testing were not met for data of the eye-point stage for both species. Therefore, Kruskal-Wallis rank sum test followed by Mann-Whitney U-test was employed for each treatment versus the control treatment (no AgNP, no NM-300 K DIS) also applying a Bonferroni adjusted alpha-threshold. Statistical requirements for parametric testing were also not met to determine the statistical difference between AgNP sizes of primary particles in different media for each time point via TEM. Again, Kruskal-Wallis rank sum test followed by Mann-Whitney U-test was used applying a Bonferroni adjusted alpha-threshold.
Results
Lethal and sublethal effects of AgNPs
The sensitivity of C. lavaretus embryos was markedly dependent on the test medium during the 8 weeks of exposure and toxicity in Lake Mondsee water was significantly higher compared to ASTM based on non-overlapping CIs (LC50-8 week: ASTM 0.73 mg/L, CI 0.62–0.83 mg/L, Lake Mondsee water 0.51 mg/L, CI 0.48–0.54 mg/L; ; see Table A 6 for all LC50 and EC50 values). The highest mortality of fish embryos occurred during the first 2 weeks in all test media at AgNP concentrations ≥0.5 mg/L (). Thereafter, minor changes in mortality were observed in ISO, whereas in ASTM and Lake Mondsee water, mortality increased on a weekly basis at concentrations ≥0.5 mg/L. The toxicity of the stabilization agent NM-300 K DIS was negligible in all media for C. lavaretus.
Figure 2. Dose-response curves and data points of AgNP exposure to C. lavaretus in ASTM (blue), ISO (red), and Lake Mondsee water (green) and R. rutilus in Lake Mondsee water (black) for (a) lethal and (b) non-lethal adverse effects. Non-lethal adverse effects include adverse factors for the embryo such as edema or phenotypic defects of the head, tail, or yolk. Coloration around each line display the respective 95% CIs. Open symbols depict the observed effects per replicate (C. lavaretus: ASTM □, ISO ○, Lake Mondsee water Δ; R. rutilus: Lake Mondsee water ◊). Black horizontal dashed line displays the median lethal/non-lethal adverse effect line. n = 3, expect for ASTM 0.1 mg/L and 0.5 mg/L, ISO 2 mg/L, and Lake Mondsee water 0.5 mg/L with n = 2, because mortality in the internal plate control exceeded 25% in one replicate
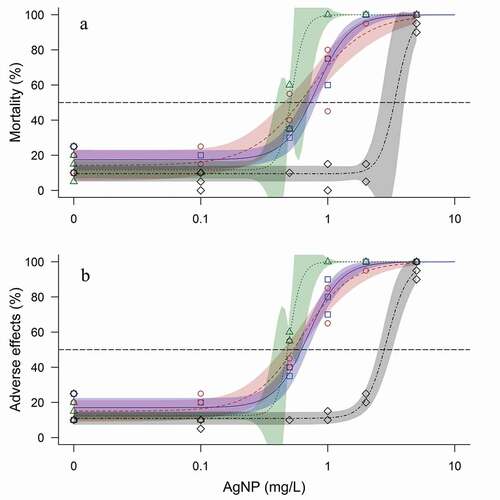
Figure 3. Average mortality over time for C. lavaretus and R. rutilus embryos after exposure (8 weeks and 9 days, respectively) to AgNPs ranging from Control (0) to 5 mg/L (nominal concentration) and stabilization agent NM-300 K DIS in ASTM, ISO, and Lake Mondsee water medium. n = 60 for each data point, except for ASTM 0.1 mg/L and 0.5 mg/L, ISO 2 mg/L, and Lake Mondsee water 0.5 mg/L with n = 40, because the mortality in the internal plate control exceeded 25% in one well plate
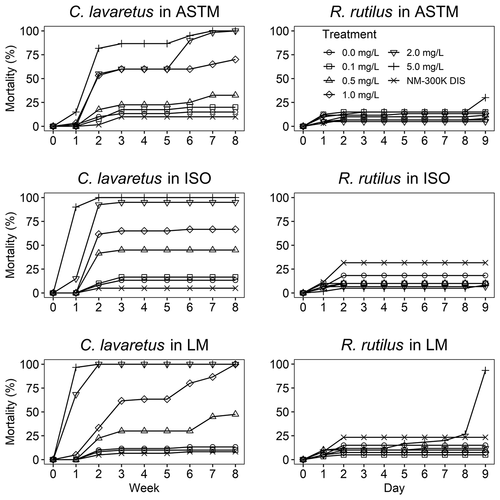
Other adverse effects such as edema, coagulation, or malformations of the head, tail, or yolk sac correlated with the mortality patterns described before and resulted in mortality in most cases within a few days () for dose–response curve of effects and Figure A 1 for time to adverse effects.
No marked effects were observed for R. rutilus when exposed to AgNPs in ASTM and ISO. However in Lake Mondsee water mortality slowly rose at 5 mg/L and changed from 26.7 ± 17.6% to 93.3 ± 2.9% during the last day of exposure (i.e. day 9, ). A numerical higher mortality was detected in the NM-300 K DIS control in ISO and Lake Mondsee water within the first two day of the experiment but did not change thereafter.
Eye-point stage
Eye pigmentation of C. lavaretus embryos was significantly delayed in ASTM spiked with 2 and 5 mg/L AgNPs (). For ISO and Lake Mondsee water, concentrations >2 mg/L and >1 mg/L respectively, resulted in 100% mortality before fish embryos reached the eye-point stage. Further, a significantly delayed eye pigmentation was noted in ISO at 2 mg/L, whereas AgNP exposure in Lake Mondsee water significantly delayed eye pigmentation at 0.5 and 1 mg/L. For R. rutilus embryos, no significant differences were observed for all treatments. Most fish embryos reached the eye-point stage on day 6 and only three embryos reached this stage on day 7 (i.e. control; 0.5 mg/L; 1 mg/L, one embryo each).
Figure 4. Developmental time (± SD) until reaching the eye-point stage for C. lavaretus embryos. Number of embryos surviving until this developmental stage in the respective AgNP exposure sample are displayed in each bar. Asterisks* indicate significant difference to the control at p < .05 Bonferroni adjusted Mann-Whitney U-test). Missing data points (bars) indicate that the mortality was at 100% before the eye-point stage was reached. n = 60 for each data point, except for ASTM 0.1 mg/L and 0.5 mg/L, ISO 2 mg/L, and Lake Mondsee water 0.5 mg/L with n = 40, because mortality in the internal plate control exceeded 25% in one well plate
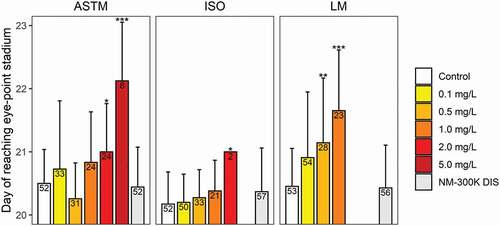
Histological analysis
The optical analysis of the fish embryos revealed that silver particles (i.e. AgNPs and large silver salt particles) could not be detected within the embryos using optical dark field microscopy. Based upon the comparison with positive control, no significant numbers of light-scattering particles were detected within the tissue, in the chorion space, and on the chorion (Figure A 2), indicating absence of AgNPs.
AgNP concentration and stability
TEM provided insight on primary particle sizes in each medium over time, irrespective of their agglomeration state. Only negligible changes in the size of primary AgNPs were found in ASTM and ISO after 168 hr, whereas primary particle size decreased in Lake Mondsee water from 14.3 ± 3.3 to 9.8 ± 3 nm () and particles were significantly smaller in Lake Mondsee water after 168 hr compared to ASTM and ISO (15.3 ± 2.1 and 13.7 ± 3.6 nm, respectively).
Table 1. Mode of the particle size distribution function in nm (± SD) of primary AgNPs (NM-300 K) in ASTM, ISO, Lake Mondsee water, and MilliQ after 0, 24, 96, and 168 hr determined by TEM. Different letters indicate significant difference between test media for each time point (Bonferroni adjusted Mann-Whitney U-test). n.a. = not analyzed
AgNPs as small as 10.5 nm (LOD) were detected with the spICP-MS using 5 µs time resolution (Figure A 3–6) after careful method optimization. This particle size LOD was only possible using a modified spICP-MS as described in section “spICP-MS measurements.” Because the obtained size distributions were better approximated by a Poisson distribution model, and not a Gaussian distribution model, average AgNP sizes could not be determined. However, spICP-MS measurements revealed a large size variation of AgNPs. Particle sizes up to 130 nm were found, while the majority of particles (95%) was found to be ≤50 nm throughout all test media and time points (see Figure A 3–6). The results further suggested that AgNPs in MilliQ, ASTM, and ISO tend to be larger (95% being ≤58 nm, ≤60 nm, and ≤51 nm respectively) compared to Lake Mondsee water (95% being ≤37 nm). In addition, less AgNP events were detected over time by spICP-MS measurements, which correlates with a decrease of total silver concentration in the test media over time, measured by ICP-MS.
Measured concentrations of total silver were considerably lower in comparison to the nominal concentration (): 68.1% of the initially spiked AgNPs (i.e. 100 µg/L) were measurable in MilliQ water after 0 hr and recovery in the three applied test media ASTM, ISO, and Lake Mondsee water were 43.4%, 18.5%, and 47.9%, respectively. Irrespective of the test medium, total silver concentrations constantly declined, getting as low as 4.4% of the originally applied AgNP concentration in ISO medium after 168 hr.
Figure 5. (a) Total silver as a measure for AgNP (n = 1 with 10 replicated measurements; nominal concentration: 100 µg/L) and (b) concentration of Ag+ dissolved from AgNPs assessed with filters of 50 kDa (n = 2 with 10 replicated measurements each; nominal concentration: 100 µg/L) in ASTM, ISO, and Lake Mondsee water after 0, 24, 96, and 168 hr. Dissolved silver from AgNP in MillQ water was only measured at 0 hr. Samples were acidified with HNO3 to pH 1–2, frozen and stored at −20°C until analysis with ICP-MS. Error bars represent standard deviation
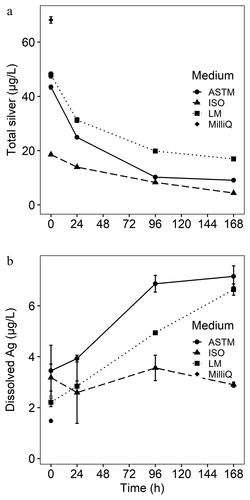
Concentrations of dissolved Ag increased from 3.5 ± 1 to 7.2 ± 0.4 µg/L in ASTM and from 2.2 ± 0.2 µg/L to 6.6 ± 0.2 µg/L in Lake Mondsee water within 168 hr, whereas differences in ISO medium were negligible (from 3.2 ± 0.5 to 2.9 ± 0.1 µg/L; B, n = 2). The initial concentration of dissolved Ag in MilliQ water was lowest at 1.5 ± 0.1 µg/L. Recovery rates for total silver of an unfiltered AgNO3 solution were 92.3% and 85.4% for nominal concentrations of 10 and 50 µg/L, respectively. Solely filtering Ag+ from this AgNO3 solution resulted in recovery rates of 102.7% and 100.2% at 10 and 50 µg/l Ag+, respectively, thus no reduction of Ag+ was expected when applying ultrafiltration.
The underlying assumption for Ag+ measurements was that the filtrate of a 50 kDa membrane does contain silver as dissolved species only and no AgNPs are present below the expected size separation limit of the filters (<5–6 nm). This assumption was verified by TEM for all media at any time point for which >99.9% of primary particles were >6 nm, with the exception of Lake Mondsee water after 168 hr. Here 9.6% of AgNPs were between 3.3 and <6 nm. These AgNPs potentially passed the 50 kDa membrane, resulting in an overestimation of released Ag+ by up to 10% for this particular sample.
According to thermodynamic calculations, Ag+ release from AgNP surfaces may result in a medium- and Ag-concentration-dependent species distribution (, Figure A 7). Assuming a presence of 5 µg Ag+/L in the test media, species distributions in ASTM and Lake Mondsee water would be comparable, with Ag+ (71.6% and 64.8%, respectively) and AgCl(aq) (25% and 34.3%, respectively) as the most common silver species. In ISO medium, Ag+ is assumed to mainly interact with Cl− by forming AgCl(aq) (66.7%) or AgCl2− (23.6%) with only a small % of Ag+ remaining in its initial form (9.5%). No precipitation of released Ag+ was predicted in all three test media at a concentration ≤150 µg/L, ≤50 µg/L, and ≤100 µg/L in ASTM, ISO, and Lake Mondsee water, respectively (Figure A 8).
Table 2. Silver species distribution (in %) according to thermodynamic calculations (based upon Gustafsson Citation2017) for low (0.5 µg/L) and high (5 µg/L) concentrations of Ag+ released from AgNPs in ASTM, ISO, and Lake Mondsee water
Discussion
Most AgNPs tend to agglomerate with increasing ionic strength (Bae et al. Citation2013; Badawy et al. Citation2010; Chambers et al. Citation2014; Metreveli et al. Citation2016; Römer et al. Citation2013) resulting in a decreased total surface area and consequently diminished dissolution of toxic Ag+ to the surrounding medium (Dobias and Bernier-Latmani Citation2013; Zook et al. Citation2012). In the present study, ionic strength was different between all three test media (ASTM: 16–18.8 mmol/L; ISO: 10.3–12.4 mmol/L; Lake Mondsee water: 5–6.1 mmol/L, Table A 1) and toxicity of AgNP seemed to directly correlate with this pattern: the toxicity of AgNP rose with decreasing ionic strength (LC50-8 week of C. lavaretus: ASTM 0.73 mg/L, CI 0.62–0.83 mg/L; ISO 0.06 mg/L, CI 0.42–0.64 mg/L; Lake Mondsee water 0.51 mg/L, CI 0.48–0.54 mg/L; ).
TEM analysis confirmed that AgNPs (i.e. NM-300 K) were significantly smaller in the medium with the lowest ionic strength (Lake Mondsee water; 9.8 ± 2.1 nm) after 168 hr than particles in all other tested media (from 13.7 ± 3.6 nm in ISO to 15.3 ± 2.1 nm in ASTM). However, no apparent correlation between ionic strength and Ag+ dissolution from AgNPs was observed within 168 hr: the concentration of dissolved Ag in the medium with the highest (ASTM) and lowest ionic strength (Lake Mondsee water) increased by 106% and 200% after 168 hr h, respectively. In contrast changes of dissolved Ag in the medium with intermediate ionic strength (ISO) were negligible, although initially being at a comparable level (2.2 ± 0.2 to 3.5 ± 1 µg/L ). The formation of solid phase precipitates (e.g. AgOH(s) and AgCl(s)), especially at high pH and high silver concentrations, may have introduced analytical biases, because solid phase precipitates might have been retained in the filters when measuring dissolved Ag. Thermodynamic calculations suggested no presence of AgOH(s) and appearance of AgCl(s) only at Ag+ concentrations above 150, 50, and 100 µg/L in ASTM, ISO, and Lake Mondsee water, respectively (Figure A 8). Thus, recovery rates for dissolved Ag released from AgNPs should not be influenced by solid phase precipitates in the present study. Kinetic processes which may also alter the formation of dissolved or solid species of Ag(I), might not be considered in the thermodynamic calculations. In addition, interactions of silver species with the filter membrane may differ between applied test media. Regardless of potential biases that might not be analyzed here, evidence indicates that the size of AgNPs in the different media was not the main reason for dissolution of the AgNPs (NM-300 K with NM-300 K DIS consisting of 4% polyoxyethylene glycerol trioleate and 4% polyoxyethylene (20) sorbitan mono-laurate) used in this study.
Chambers et al. (Citation2014) observed that higher ionic strength produced agglomeration for mercaptosuccinic-acid-capped AgNPs and chloride seemed to be a driving factor for their dissolution. In this case chloride concentrations were highest in ISO (144 mg/L), but comparably low in ASTM and Lake Mondsee water (8 and 10 mg/L, respectively), again not explaining the observed dissolution pattern. Therefore, AgNP dissolution patterns in the present study might be a result of other anions present in the three test media, but current research cannot fully explain the factors driving this process. Thus, further studies are needed to elucidate AgNP dissolution patterns in different test media, and impact of AgNP coatings and particle sizes.
Total silver concentrations as low as 18.5% of the initially spiked AgNP concentrations were measurable shortly after medium preparation (ISO, time point 0 hr; ). After 168 hr, recovery rates for ISO decreased to 4.4%. The same trend was also noted in the spICP-MS measurements, where the amount of AgNP particles was found to significantly fall over time (Figure A 3–6). Sample storage was essential in the present study, since sample shipping was required to perform ICP-MS analysis, but potentially introduced an error for the measurements. In addition, Ag+ and AgNPs are highly attracted by surface materials, such as glass, polystyrene, polycarbonate, and polypropylene, commonly used in ecotoxicological studies, resulting in recovery rates as low as 29.4% for spiked Ag+ from AgNO3 and even 0.8% for branched-polyethyleneimine coated AgNPs after 72 hr (Sekine et al. Citation2015). Thus, adsorption to the test vessels may explain the reduced initial AgNP concentration and constant AgNP decline in all three test media over time. Similarly, adsorption or uptake may have also occurred on fish embryos, however, this was not measurable with our applied techniques.
Chloride in the test medium was reported to decrease silver toxicity for Oncorhynchus mykiss by forming less toxic solid phase silver chloride (Brauner et al. Citation2003; Bury, Galvez, and Wood Citation1999; Morgan et al. Citation2005). Similar effects were found for D. rerio embryos exposed to AgNPs (Groh et al. Citation2015). Metreveli et al. (Citation2016) analyzed the species distribution of citrate-stabilized AgNPs in several ecotoxicological standard test media combining data obtained from dissolution experiments and species calculations. Metreveli et al. (Citation2016) noted that 5% of Ag+ are released from 30 nm citrate-stabilized AgNPs in ASTM hard water (Cl−: 3.8 mg/L) after 1 day, whereof 16% were transformed to dissolved AgCl(aq). By further addition of chloride (Cl−: 53.2 mg/L) the amount of transformation was elevated to 26% and additionally, 62% of released silver transformed to the solid phase precipitates of AgCl(s) (Metreveli et al. Citation2016) demonstrating the influence of the applied test medium on Ag+ speciation. Thermodynamic calculations of the silver species distribution in the present study revealed that the chloride concentration in the test medium markedly affected transformation of released Ag+ to AgCl(aq) (Figure A 7). In ISO medium, only 9.7% of initially released Ag+ was present as Ag+, whereas the remaining Ag+ was mainly transformed to AgCl(aq) (66.6%) or AgCl2− (23.6%). In comparison, 71.6% and 64.8% of initial Ag+ was still present as Ag+ in ASTM and Lake Mondsee water, respectively. At the same time, the high presence of sulfate in ASTM, which was reported to lower AgNP-induced toxicity for autotrophic nitrifying bacteria (Choi and Hu Citation2009), led to formation of AgSO4− in ASTM (2.9%).
Measuring dissolved Ag released from AgNPs (via filtration and ICP-MS) in combination with thermodynamic calculations for Ag+ speciations indicates that the presence of dissolved Ag in the test media cannot solely explain the observed adverse effects for C. lavaretus and R. rutilus embryos. However, the greater toxicity in the medium with the lowest ionic strength (Lake Mondsee water) might be related to significantly smaller size of AgNP in Lake Mondsee water compared to ASTM after 168 hr (9.8 ± 3 nm and 15.3 ± 2.1 nm, respectively). Therefore, identifying the link between test media, AgNP size, and ultimately AgNP toxicity needs to be a topic for further research.
The time-to-death analysis provided insight on fish embryo-specific responses to AgNP exposure (). For C. lavaretus mortality and adverse effects were most prominent during the first two weeks in a dose-dependent manner for all concentrations ≥0.5 mg/L. This time period included two critical developmental stages for C. lavaretus embryos (i.e. cleavage and early gastrulation; Kamler Citation1992a), potentially explaining the observed mortality pattern. For R. rutilus, a numerically higher mortality was found in the NM-300 K DIS control at the start of the experiment in ISO and Lake Mondsee water, but this might be a result of nonsuccessful fertilization, which might frequently occur within the first days. However, at the beginning of the organogenesis (day 4 to 5), mortality of R. rutilus embryos slowly increased in the highest AgNP concentration (5 mg/L) in one test medium (Lake Mondsee water) up to 93.3 ± 2.9% shortly before hatching (day 9).
For C. lavaretus embryos a LC50-8 week-range of 0.51 to 0.73 mg/L was obtained which is at the lower end compared to toxicity data reported by other investigators (Kashiwada et al. Citation2012; Laban et al. Citation2010; Muth-Köhne et al. Citation2013). Effects such as edema, coagulation, or malformations of the head, tail, or yolk sac, detected here, were also reported for other fish species (Asharani et al. Citation2011; Kashiwada et al. Citation2012; Muth-Köhne et al. Citation2013). These defects predominantly resulted in mortality within a few days, thus, embryos being adversely affected by AgNPs toward the end of the study may have likely died during or shortly after hatching.
The large variety in sizes and coatings of AgNPs entails difficulty of comparing observations between several studies, since both factors exert significant implications on the toxicity toward a test species (Cunningham et al. Citation2013; Kwok et al. Citation2012; Yang et al. Citation2012). Böhme et al. (Citation2015) reported a LC50 of 39.5 µg/L for 10–21 nm polyvinylpyrrolidone-coated AgNPs toward D. rerio after 48 hr, while Muth-Köhne et al. (Citation2013) noted a LC50 of 1.26 mg/L using the same species, test medium, and duration, but different AgNPs (15 nm, NM-300 K; see Klein et al. Citation2011). Griffitt et al. (Citation2008) detected an LC50 of 7.2 mg/L for D. rerio embryos (<24 hpf) using 20–30 nm metal oxide coated AgNPs after 48 hr, which is more than two orders of magnitude higher than the LC50 reported by Böhme et al. (Citation2015). For O. latipes embryos, LC50s were reported between 0.6 and 1.41 mg/L(Cho et al. Citation2013; Kashiwada et al. Citation2012; Wu et al. Citation2010), and P. promelas embryos are within a comparable range (1.25–1.36 mg/L; Laban et al. Citation2010).
Muth-Köhne et al. (Citation2013) indicated that AgNP-mediated toxicity toward D. rerio embryos may be compared with our results of C. lavaretus and R. rutilus, since the same test medium (ISO) and AgNPs (NM-300 K; Klein et al. Citation2011) was applied. Data indicated that embryos of C. lavaretus are at least 2-fold more sensitive than D. rerio (LC50: 0.6 and 1.26 mg/L, EC50: 0.53 and 1.09 mg/L, respectively). It is important to note that the exposure duration was only 48 hr for D. rerio compared to 8 weeks for C. lavaretus, however, the highest environmental exposure to AgNP and their transformation products might be expected in sediments (Gottschalk et al. Citation2009; Vogt et al. Citation2019). Therefore, considering the time spent for embryogenesis in potentially AgNP enriched sediments is of high ecological importance and needs to be considered when evaluating the risk of nanomaterials.
The surface properties of the chorion of C. lavaretus and R. rutilus embryos might also influenced AgNP-induced toxicity (Ali, Saber, and Taite Citation2017; Auffan et al. Citation2014). The chorion is a protective layer for fish embryos against several chemical stressors (Ali, Saber, and Taite Citation2017; Hagedorn et al. Citation1998; Villalobos et al. Citation2000), while enabling an interaction with the surrounding medium via chorion pores to ensure oxygen and water supply (Groot and Alderdice Citation1985; Hisaoka Citation1958). The applied optical dark field microscopy protocol could not confirm the presence of AgNP-aggregates on or inside the chorion, but AgNPs (14.4 ± 2.5 nm) used in the present study are smaller than chorion pores of most teleost species resent in alpine and subalpine regions by at least one order of magnitude (e.g. R. rutilus, 0.2 µm, Patzner, Riehl, and Glechner Citation1996; Rutilus frisii meidingeri, 0.5 µm, Citation1996; Chondrostoma nasus, 0.18 µm; Patzner, Weidinger, and Riehl Citation2006). Therefore, an uptake via chorion pores of both tested fish species is conceivable.
Accumulation of AgNPs inside the chorion was reported for several species including D. rerio (Asharani et al. Citation2008; Lee et al. Citation2012, Citation2007; Muth-Köhne et al. Citation2013), Pimephales promelas (Laban et al. Citation2010), Fundulus heteroclitus (Auffan et al. Citation2014), or O. latipes (Kashiwada et al. Citation2012). Once entered into the egg fluid, AgNPs might accumulate in the liver, the gills, and in several other organs (Jung et al. Citation2014) resulting in cellular injuries due to the highly reactive surface of AgNPs (George et al. Citation2012; Laban et al. Citation2010). In contrast, Osborne et al. (Citation2013) showed that AgNPs and titanium dioxide nanoparticles of 10 and 35 nm each, were not able to pass the chorion of D. rerio. Similar results were obtained by Fent et al. (Citation2010) using fluorescent silica nanoparticles at 60 and 200 nm. AgNPs were also observed to agglomerate inside the chorion pores, initiating indirect effects by hindering transportation processes via clogging of the chorion pores (Lee et al. Citation2012, Citation2007). Since blood circulation in fish embryos evolving inside the chorion is poorly developed, diffusion of oxygen via chorion pores is vital for the fish embryos (Kamler Citation1992b). Although the presence of AgNPs could not be confirmed on the surface of the chorion using optical dark field microscopy, clogging of chorion pores cannot be ruled out and further research on the protective function of the chorion toward AgNP exposure is needed.
To our knowledge, no toxicity test was performed to assess adverse effects of AgNPs using R. rutilus embryos and only one study was performed using a species of the same species complex as C. lavaretus embryos. Clark et al. (Citation2016) found early hatching of C. palaea embryos at 0.1 mg/LAgNPs, but mortality or other adverse effects did not occur (test medium: ISO), which is in agreement with the results obtained here. In contrast to the present study, AgNP exposure to C. lavaretus embryos started at 136.5 degree days (DD; DD = rearing temperature multiplied by days post fertilization), which corresponds to the observed results after 26–27 days (135.2–140.4 DD); a time-point, where mortality was already 32% higher for concentrations ≥0.5 mg/L when compared to control (). The tests in the present study were terminated shortly before hatching to determine the presence of AgNPs on the chorion, in the chorion space, and in the fish embryos via optical dark field microscopy. After hatching, such analyses are not possible due to the direct contact of the hatched larvae with the test medium, thus, the day of hatching was not assessed here. Clark et al. (Citation2016) suggested that an extended exposure duration throughout the whole embryogenesis might result in early hatching of embryos even at concentrations as low as 0.1 mg/L. As an alternative to the day of hatching of the fish larvae, the day of reaching the eye-point stage was assessed as a measure for their embryonic development. The embryonal development showed a clear dose-dependent manner for C. lavaretus embryos in all test media (), with significant effects at ≥0.5 mg/L in Lake Mondsee water. However, in ASTM and ISO development was significantly delayed at ≥2 mg/L, again showing a lower toxicity of AgNPs in the two standard test media compared to natural Lake Mondsee water.
Finally, concentrations that adversely affect fish species occurring in temperate aquatic habitats are currently orders of magnitude higher than the predicted environmental concentrations of AgNPs up to 0.08 µg/L in water (Mueller and Nowack Citation2008) and up to 16 µg/kg in sediments (Gottschalk, Sun, and Nowack Citation2013). However, a steady accumulation of AgNPs in lake sediments potentially becoming as high as 8.6 µg/kg per year (Gottschalk et al. Citation2009) may finally lead to a future risk for fish species spending their embryogenesis on or near sediments.
Conclusions
Data demonstrated the influence of the test medium on AgNP dissolution, Ag+ species distribution dissolved from AgNPs, and toxicity for fish species occurring in temperate aquatic habitats (i.e. C. lavaretus and R. rutilus). Ionic strength, which was reported to correlate with agglomeration as well as dissolution for several types of AgNPs, showed no clear pattern for AgNPs used in this study (i.e. NM-300 K) for both cases. For the first time, AgNP-induced toxicity was assessed over the whole embryogenesis of C. lavaretus and R. rutilus, two highly relevant species for temperate aquatic environments. Data showed that C. lavaretus is markedly more sensitive to AgNP exposure compared to R. rutilus. Further, C. lavaretus embryos were more sensitive compared to the standard test organism D. rerio exposed to the same AgNP species and same test medium (ISO; Muth-Köhne et al. Citation2013). The AgNP dissolution, although initially expected to exert significant implications on AgNP-induced toxicity, was not the driving factor for the observed mortality, as the highest dissolution was measured in the test medium with the highest LC50s (i.e. ASTM). In addition, standard test media provided by the ASTM or ISO guideline overestimated the LC50s compared to natural lake water by 30% and 15%, respectively, but physicochemical parameters influencing the toxicity were not clearly identified. Therefore, the present study indicates the urgent need for further research on physicochemical parameters driving AgNP-mediated toxicity. Consideration needs to be given to nonstandard test organisms and incubation media in ecotoxicological studies in order enable a comprehensive risk assessment of AgNPs in aquatic environments.
Supplemental Material
Download MS Word (3.8 MB)Acknowledgments
This study was supported by the FP7 ERA-NET on Nanosafety: Safe Implementation of Innovative Nanoscience and Nanotechnology (SIINN) within the project FENOMENO (Grant No.: 03XP0005), the German Federal Ministry of Education and Research (BMBF; 03XP0005A), the University of Siegen, and from the Austrian Research Promotion Agency (FFG; 844421). This research was also partly funded by the German Research Foundation, research unit INTERNANO (FOR 1536 “Mobility, aging and functioning of engineered inorganic nanoparticles at the aquatic–terrestrial interface,” subproject SCHA849/16). The authors thank A. Wiedelroither and B. Teufl for their support in the laboratory, J. Schmidt (Research Department for Limnology, Mondsee, University of Innsbruck) for performing the IC-measurements, N. Heupel (University of Siegen) for her help in the sample preparation for ICP-MS, as well as U. Jonas (University of Siegen) for access to the optical dark field microscope. Part of this work was performed at the Micro- and Nanoanalytics Facility (MNaF) of the University of Siegen. Finally, we want to thank four anonymous reviewers for their helpful comments in the draft of this manuscript.
Disclosure statement
All authors and co-authors declare that no conflict of interest exists in any way.
Data Availability Statement
All supportive data and associated metadata are available in the supplementary material.
Supplementary material
Supplemental data for this article can be accessed on the publisher’s website
Additional information
Funding
References
- Ali, M., S. Saber, and S. Taite. 2017. The protective layer of zebrafish embryo changes continuously with advancing age of embryo development (AGED). J. Toxicol. Pharmacol 1:009.
- Asharani, P. V., Y. L. Wu, Z. Gong, and S. Valiyaveettil. 2008. Toxicity of silver nanoparticles in zebrafish models. Nanotechnol 19 (25):255102. doi:https://doi.org/10.1088/0957-4484/19/25/255102.
- Asharani, P. V., Y. L. Wu, Z. Gong, and S. Valiyaveettil. 2011. Comparison of the toxicity of silver, gold and platinum nanoparticles in developing zebrafish embryos. Nanotoxicol 5 (1):43–54. doi:https://doi.org/10.3109/17435390.2010.489207.
- ASTM (ASTM International). 2007. Standard guide for conducting acute toxicity tests on test materials with fishes, macroinvertebrates, and amphibians (E729-96, Reapproved 2007). West Conshohocken, PA, United States.
- Auffan, M., C. W. Matson, J. Rose, M. Arnold, O. Proux, B. Fayard, W. Liu, P. Chaurand, M. R. Wiesner, J. Y. Bottero, et al. 2014. Salinity-dependent silver nanoparticle uptake and transformation by Atlantic killifish (Fundulus heteroclitus) embryos. Nanotoxicol 8 (Suppl 1):167–76. doi:https://doi.org/10.3109/17435390.2013.869627.
- Badawy, A. M. E., T. P. Luxton, R. G. Silva, K. G. Scheckel, M. T. Suidan, and T. M. Tolaymat. 2010. Impact of environmental conditions (pH, ionic strength, and electrolyte type) on the surface charge and aggregation of silver nanoparticles suspensions. Environ. Sci. Technol 44 (4):1260–66. doi:https://doi.org/10.1021/es902240k.
- Bae, S., Y. S. Hwang, Y. J. Lee, and S. K. Lee. 2013. Effects of water chemistry on aggregation and soil adsorption of silver nanoparticles. Environ. Health Toxicol 28:e2013006. doi:https://doi.org/10.5620/eht.2013.28.e2013006.
- Blaser, S. A., M. Scheringer, M. Macleod, and K. Hungerbuhler. 2008. Estimation of cumulative aquatic exposure and risk due to silver: Contribution of nano-functionalized plastics and textiles. Sci. Total Environ 390 (2–3):396–409. doi:https://doi.org/10.1016/j.scitotenv.2007.10.010.
- Böhme, S., H.-J. Stärk, T. Reemtsma, and D. Kühnel. 2015. Effect propagation after silver nanoparticle exposure in zebrafish (Danio rerio) embryos: A correlation to internal concentration and distribution patterns. Environ. Sci. Nano 2 (6):603–14. doi:https://doi.org/10.1039/C5EN00118H.
- Boyle, D., and G. G. Goss. 2018. Effects of silver nanoparticles in early life-stage zebrafish are associated with particle dissolution and the toxicity of soluble silver. NanoImpact 12:1–8. doi:https://doi.org/10.1016/j.impact.2018.08.006.
- Brauner, C. J., J. Wilson, C. Kamunde, and C. M. Wood. 2003. Water chloride provides partial protection during chronic exposure to waterborne silver in rainbow trout (Oncorhynchus mykiss) embryos and larvae. Physiol. Biochem. Zool 76 (6):803–15. doi:https://doi.org/10.1086/378136.
- Bury, N. R., F. Galvez, and C. M. Wood. 1999. Effects of chloride, calcium, and dissolved organic carbon on silver toxicity: Comparison between rainbow trout and fathead minnows. Environ. Toxicol. Chem 18 (1):56–62. doi:https://doi.org/10.1002/etc.5620180108.
- Chambers, B. A., A. R. Afrooz, S. Bae, N. Aich, L. Katz, N. B. Saleh, and M. J. Kirisits. 2014. Effects of chloride and ionic strength on physical morphology, dissolution, and bacterial toxicity of silver nanoparticles. Environ. Sci. Technol 48 (1):761–69. doi:https://doi.org/10.1021/es403969x.
- Chappell, M. A., L. F. Miller, A. J. George, B. A. Pettway, C. L. Price, B. E. Porter, A. J. Bednar, J. M. Seiter, A. J. Kennedy, and J. A. Steevens. 2011. Simultaneous dispersion-dissolution behavior of concentrated silver nanoparticle suspensions in the presence of model organic solutes. Chemosphere 84 (8):1108–16. doi:https://doi.org/10.1021/es403969x.
- Cho, J. G., K. T. Kim, T. K. Ryu, J. W. Lee, J. E. Kim, J. Kim, B. C. Lee, E. H. Jo, J. Yoon, I. C. Eom, et al. 2013. Stepwise embryonic toxicity of silver nanoparticles on Oryzias latipes. Biomed. Res. Int 2013:494671. doi:https://doi.org/10.1155/2013/494671.
- Choi, O., and Z. Q. Hu. 2009. Nitrification inhibition by silver nanoparticles. Water Sci. Technol 59 (9):1699–702. doi:https://doi.org/10.2166/wst.2009.205.
- Cingi, S., M. Keinänen, and P. J. Vuorinen. 2010. Elevated water temperature impairs fertilization and embryonic development of whitefish Coregonus lavaretus. J. Fish Biol 76 (3):502–21. doi:https://doi.org/10.1111/j.1095-8649.2009.02502.x.
- Clark, E. S., M. Pompini, A. Uppal, and C. Wedekind. 2016. Genetic correlations and little genetic variance for reaction norms may limit potential for adaptation to pollution by ionic and nanoparticulate silver in a whitefish (Salmonidae). Ecol. Evolut 6 (9):2751–62. doi:https://doi.org/10.1002/ece3.2088.
- Core Team, R. 2016. R: A language and environment for statistical computing (version 3.6.2). Vienna, Austria: R Foundation for Statistical Computing.
- Cunningham, S., M. E. Brennan-Fournet, D. Ledwith, L. Byrnes, and L. Joshi. 2013. Effect of nanoparticle stabilization and physicochemical properties on exposure outcome: acute toxicity of silver nanoparticle preparations in zebrafish (Danio rerio). Environ. Sci. Technol 47 (8):3883–92. doi:https://doi.org/10.1021/es303695f.
- Currie, L. A. 1972. The measurement of environmental levels of rare gas nuclides and the treatment of very low-level counting data. IEEE Trans. Nucl. Sci 19 (1):119–26. doi:https://doi.org/10.1109/TNS.1972.4326496.
- Dobias, J., and R. Bernier-Latmani. 2013. Silver release from silver nanoparticles in natural waters. Environ. Sci. Technol 47 (9):4140–46. doi:https://doi.org/10.1021/es304023p.
- Fent, K., C. J. Weisbrod, A. Wirth-Heller, and U. Pieles. 2010. Assessment of uptake and toxicity of fluorescent silica nanoparticles in zebrafish (Danio rerio) early life stages. Aquat. Toxicol 100 (2):218–28. doi:https://doi.org/10.1016/j.aquatox.2010.02.019.
- George, S., S. Lin, Z. Ji, C. R. Thomas, L. Li, M. Mecklenburg, H. Meng, X. Wang, H. Zhang, and T. Xia. 2012. Surface defects on plate-shaped silver nanoparticles contribute to its hazard potential in a fish gill cell line and zebrafish embryos. ACS Nano 6 (5):3745–59. doi:https://doi.org/10.1021/nn204671v.
- Gottschalk, F., T. Sonderer, R. W. Scholz, and B. Nowack. 2009. Modeled environmental concentrations of engineered nanomaterials (TiO2, ZnO, Ag, CNT, Fullerenes) for different regions. Environ. Sci. Technol 43 (24):9216–22. doi:https://doi.org/10.1021/es9015553.
- Gottschalk, F., T. Sun, and B. Nowack. 2013. Environmental concentrations of engineered nanomaterials: Review of modeling and analytical studies. Environ. Pollut 181:287–300. doi:https://doi.org/10.1016/j.envpol.2013.06.003.
- Griffitt, R. J., J. Luo, J. Gao, J. C. Bonzongo, and D. S. Barber. 2008. Effects of particle composition and species on toxicity of metallic nanomaterials in aquatic organisms. Environ. Toxicol. Chem 27 (9):1972–78. doi:https://doi.org/10.1897/08-002.1.
- Groh, K. J., T. Dalkvist, F. Piccapietra, R. Behra, M. J.-F. Suter, and K. Schirmer. 2015. Critical influence of chloride ions on silver ion-mediated acute toxicity of silver nanoparticles to zebrafish embryos. Nanotoxicol 9 (1):81–91. doi:https://doi.org/10.3109/17435390.2014.893379.
- Groot, E., and D. Alderdice. 1985. Fine structure of the external egg membrane of five species of Pacific salmon and steelhead trout. Can. J. Zool 63 (3):552–66. doi:https://doi.org/10.1139/z85-082.
- Gustafsson, J. P. 2017. Visual MINTEQ. Page https://vminteq.lwr.kth.se/, last visited: November 2017.
- Hagedorn, M., F. Kleinhans, D. Artemov, and U. Pilatus. 1998. Characterization of a major permeability barrier in the zebrafish embryo. Biol. Reprod 59 (5):1240–50. doi:https://doi.org/10.1095/biolreprod59.5.1240.
- Hartmann, G., C. Hutterer, and M. Schuster. 2013. Ultra-trace determination of silver nanoparticles in water samples using cloud point extraction and ETAAS. J.Anal. Atom Spectrom 28 (4):567–72. doi:https://doi.org/10.1039/C3JA30365A.
- Hawkins, A. D., C. Thornton, A. J. Kennedy, K. Bu, J. Cizdziel, W. Bradley, J. A. Steevens, and K. L. Willett. 2015. Gill histopathologies following exposure to nanosilver or silver nitrate. J. Toxicol. Environ. Health Part A 78 (5):301–15. doi:https://doi.org/10.1080/15287394.2014.971386.
- Hisaoka, K. K. 1958. Microscopic studies of the teleost chorion. Trans. Am. Microsc. Soc 77 (3):240–43. doi:https://doi.org/10.2307/3223685.
- ISO (International Organization for Standardization). 1992. Water quality - Determination of the acute lethal toxicity of substances to a freshwater fish [Brachydanio rerio Hamilton-Buchanan (Teleostei, Cyprinidae)]. Semi-static method, Geneva, Switzerland.
- Jung, Y., G. Metreveli, C. B. Park, S. Baik, and G. E. Schaumann. 2018. Implications of pony lake fulvic acid for the aggregation and dissolution of oppositely charged surface-coated silver nanoparticles and their ecotoxicological effects on Daphnia magna. Environ. Sci. Technol 52 (2):436–45. doi:https://doi.org/10.1021/acs.est.7b04635.
- Jung, Y.-J., K.-T. Kim, J. Y. Kim, S.-Y. Yang, B.-G. Lee, and S. D. Kim. 2014. Bioconcentration and distribution of silver nanoparticles in Japanese medaka (Oryzias latipes). J. Hazard. Mater 267:206–13. doi:https://doi.org/10.1016/j.jhazmat.2013.12.061.
- Kamler, E. 1992a. Critical periods in fish early life. In Early life history of fish: An energetics approach, edited by E. Kamler, 172. 1st. Springer Science & Business Media, Dordrecht, Netherlands. doi: https://doi.org/10.1007/978-94-011-2324-2.
- Kamler, E. 1992b. Metabolism. In Early life history of fish: An energetics approach, edited by E. Kamler, 144–61. 1st. Springer Science & Business Media, Dordrecht, Netherlands. doi: https://doi.org/10.1007/978-94-011-2324-2.
- Kashiwada, S., M. E. Ariza, T. Kawaguchi, Y. Nakagame, B. S. Jayasinghe, K. Gartner, H. Nakamura, Y. Kagami, T. Sabo-Attwood, P. L. Ferguson, et al. 2012. Silver nanocolloids disrupt medaka embryogenesis through vital gene expressions. Environ. Sci. Technol 46 (11):6278–87. doi:https://doi.org/10.1021/es2045647.
- Kaweeteerawat, C., P. N. Ubol, S. Sangmuang, S. Aueviriyavit, and R. Maniratanachote. 2017. Mechanisms of antibiotic resistance in bacteria mediated by silver nanoparticles. J. Toxicol. Environ. Health Part A 80 (23–24):1276–89. doi:https://doi.org/10.1080/15287394.2017.1376727.
- Kim, J., S. Kim, and S. Lee. 2011. Differentiation of the toxicities of silver nanoparticles and silver ions to the Japanese medaka (Oryzias latipes) and the cladoceran Daphnia magna. Nanotoxicol 5 (2):208–14. doi:https://doi.org/10.3109/17435390.2010.508137.
- Kittler, S., C. Greulich, J. Diendorf, M. Köller, and M. Epple. 2010. Toxicity of silver nanoparticles increases during storage because of slow dissolution under release of silver ions. Chem. Mat 22 (16):4548–54. doi:https://doi.org/10.1021/cm100023p.
- Klein, C., S. Comero, B. Stahlmecke, J. Romazanov, T. Kuhlbusch, E. Van Doren, P. De Temmerman, J. Mast, P. Wick, and H. Krug. 2011. NM-Series of representative manufactured nanomaterials: NM-300 Silver characterisation, stability, homogeneity. EUR 24693 EN-2011. doi:https://doi.org/10.2788/23079.
- Kortet, R., A. Vainikka, M. J. Rantala, J. Myntti, and J. Taskinen. 2004. In vitro embryo survival and early viability of larvae in relation to male sexual ornaments and parasite resistance in roach, Rutilus rutilus L. J. Evol. Biol 17 (6):1337–44. doi:https://doi.org/10.1111/j.1420-9101.2004.00760.x.
- Kottelat, M., and J. Freyhof. 2007. Handbook of European freshwater fishes. Cornol, Switzerland: Publications Kottelat.
- Kreyling, W. G., S. Fertsch-Gapp, M. Schäffler, B. D. Johnston, N. Haberl, C. Pfeiffer, J. Diendorf, C. Schleh, S. Hirn, M. Semmler-Behnke, et al. 2014. In vitro and in vivo interactions of selected nanoparticles with rodent serum proteins and their consequences in biokinetics. Beilstein J. Nanotechnol 5:1699–711. doi:https://doi.org/10.3762/bjnano.5.180.
- Kwok, K. W., M. Auffan, A. R. Badireddy, C. M. Nelson, M. R. Wiesner, A. Chilkoti, J. Liu, S. M. Marinakos, and D. E. Hinton. 2012. Uptake of silver nanoparticles and toxicity to early life stages of Japanese medaka (Oryzias latipes): Effect of coating materials. Aquat. Toxicol 120-121:59–66. doi:https://doi.org/10.1016/j.aquatox.2012.04.012.
- Laban, G., L. F. Nies, R. F. Turco, J. W. Bickham, and M. S. Sepulveda. 2010. The effects of silver nanoparticles on fathead minnow (Pimephales promelas) embryos. Ecotoxicol 19 (1):185–95. doi:https://doi.org/10.1007/s10646-009-0404-4.
- Lee, K. J., L. M. Browning, P. D. Nallathamby, T. Desai, P. K. Cherukuri, and X. H. Xu. 2012. In vivo quantitative study of sized-dependent transport and toxicity of single silver nanoparticles using zebrafish embryos. Chem. Res. Toxicol 25 (5):1029–46. doi:https://doi.org/10.1021/tx300021u.
- Lee, K. J., P. D. Nallathamby, L. M. Browning, C. J. Osgood, and X. H. Xu. 2007. In vivo imaging of transport and biocompatibility of single silver nanoparticles in early development of zebrafish embryos. ACS Nano 1 (2):133–43. doi:https://doi.org/10.1021/nn700048y.
- Lekamge, S., A. F. Miranda, B. Pham, A. S. Ball, R. Shukla, and D. Nugegoda. 2019. The toxicity of non-aged and aged coated silver nanoparticles to the freshwater shrimp Paratya australiensis. J. Toxicol. Environ. Health Part A 82 (23–24):1207–22. doi:https://doi.org/10.1080/15287394.2019.1710887.
- Lelek, A. 1987. Threatened fishes of Europe. Vol. 9 of The freshwater fishes of Europe. Wiesbaden, Germany: Aula-Verlag.
- Levard, C., E. M. Hotze, B. P. Colman, A. L. Dale, L. Truong, X. Y. Yang, A. J. Bone, G. E. Brown Jr, R. L. Tanguay, and R. T. Di Giulio. 2013. Sulfidation of silver nanoparticles: Natural antidote to their toxicity. Environ. Sci. Technol 47 (23):13440–48. doi:https://doi.org/10.1021/es403527n.
- Li, L., G. Hartmann, M. Döblinger, and M. Schuster. 2013. Quantification of nanoscale silver particles removal and release from municipal wastewater treatment plants in Germany. Environ. Sci. Technol 47 (13):7317–23. doi:https://doi.org/10.1021/es3041658.
- Li, L., M. Stoiber, A. Wimmer, Z. Xu, C. Lindenblatt, B. Helmreich, and M. Schuster. 2016. To what extent can full-scale wastewater treatment plant effluent influence the occurrence of silver-based nanoparticles in surface waters? Environ. Sci. Technol 50 (12):6327–33. doi:https://doi.org/10.1021/acs.est.6b00694.
- Liu, J., and R. H. Hurt. 2010. Ion release kinetics and particle persistence in aqueous nano-silver colloids. Environ. Sci. Technol 44 (6):2169–75. doi:https://doi.org/10.1021/es9035557.
- Ma, R., C. Levard, S. M. Marinakos, Y. Cheng, J. Liu, F. M. Michel, G. E. Brown, and G. V. Lowry. 2012. Size-controlled dissolution of organic-coated silver nanoparticles. Environ. Sci. Technol 46 (2):752–59. doi:https://doi.org/10.1021/es201686j.
- Maier, D., and A. Grohmann. 1977. Bestimmung der Ionenstärke natürlicher Wässer aus deren elektrischer Leitfähigkeit. Zeitschrift für Wasser- und Abwasserforschung 10:9–12.
- Metreveli, G., B. Frombold, F. Seitz, A. Grün, A. Philippe, R. R. Rosenfeldt, M. Bundschuh, R. Schulz, W. Manz, and G. E. Schaumann. 2016. Impact of chemical composition of ecotoxicological test media on the stability and aggregation status of silver nanoparticles. Environ. Sci. Nano 3 (2):418–33. doi:https://doi.org/10.1039/C5EN00152H.
- Monteiro, D. R., L. F. Gorup, A. S. Takamiya, A. C. Ruvollo-Filho, E. R. de Camargo, and D. B. Barbosa. 2009. The growing importance of materials that prevent microbial adhesion: Antimicrobial effect of medical devices containing silver. Int. J. Antimicrob. Agents 34 (2):103–10. doi:https://doi.org/10.1016/j.ijantimicag.2009.01.017.
- Morgan, T. P., C. M. Guadagnolo, M. Grosell, and C. M. Wood. 2005. Effects of water hardness on the physiological responses to chronic waterborne silver exposure in early life stages of rainbow trout (Oncorhynchus mykiss). Aquat. Toxicol 74 (4):333–50. doi:https://doi.org/10.1016/j.aquatox.2005.05.017.
- Mozhayeva, D., and C. Engelhard. 2017. Separation of silver nanoparticles with different coatings by capillary electrophoresis coupled to ICP-MS in single particle mode. Anal. Chem 89 (18):9767–74. doi:https://doi.org/10.1021/acs.analchem.7b01626.
- Mozhayeva, D., and C. Engelhard. 2019. A quantitative nanoparticle extraction method for microsecond time resolved single-particle ICP-MS data in the presence of a high background. J.Anal. Atom Spectrom 34 (8):1571–80. doi:https://doi.org/10.1039/c9ja00042a.
- Mozhayeva, D., and C. Engelhard. 2020. A critical review of single particle inductively coupled plasma mass spectrometry – A step towards the ideal method for nanomaterials characterization. J.Anal. Atom Spectrom 35 (9):1740–83. doi:https://doi.org/10.1039/C9JA00206E.
- Mozhayeva, D., I. Strenge, and C. Engelhard. 2017. Implementation of online preconcentration and microsecond time resolution to capillary electrophoresis single particle inductively coupled plasma mass spectrometry (CE-SP-ICP-MS) and its application in silver nanoparticle analysis. Anal. Chem 89 (13):7152–59. doi:https://doi.org/10.1021/acs.analchem.7b01185.
- Mueller, N. C., and B. Nowack. 2008. Exposure modeling of engineered nanoparticles in the environment. Environ. Sci. Technol 42 (12):4447–53. doi:https://doi.org/10.1021/es7029637.
- Muth-Köhne, E., L. Sonnack, K. Schlich, F. Hischen, W. Baumgartner, K. Hund-Rinke, C. Schafers, and M. Fenske. 2013. The toxicity of silver nanoparticles to zebrafish embryos increases through sewage treatment processes. Ecotoxicol 22 (8):1264–77. doi:https://doi.org/10.1007/s10646-013-1114-5.
- Nowack, B., and T. D. Bucheli. 2007. Occurrence, behavior and effects of nanoparticles in the environment. Environ. Pollut 150 (1):5–22. doi:https://doi.org/10.1016/j.envpol.2007.06.006.
- OECD (Organisation for Economic Co-operation and Development). 1992. Test No. 203: Fish, acute toxicity test. OECD Publishing.
- OECD (Organisation for Economic Co-operation and Development). 2004. Test no. 202: Daphnia sp. Acute immobilisation test. OECD Publishing.
- OECD (Organisation for Economic Co-operation and Development). 2012. Test no. 211: Daphnia magna reproduction test. OECD Publishing.
- OECD (Organisation for Economic Co-operation and Development). 2013. Test no. 236: Fish embryo acute toxicity (FET) test. OECD Publishing.
- OECD (Organisation for Economic Co-operation and Development). 2017. Test no. 318: Dispersion stability of nanomaterials in simulated environmental media. OECD Publishing.
- Osborne, O. J., B. D. Johnston, J. Moger, M. Balousha, J. R. Lead, T. Kudoh, and C. R. Tyler. 2013. Effects of particle size and coating on nanoscale Ag and TiO2 exposure in zebrafish (Danio rerio) embryos. Nanotoxicol 7 (8):1315–24. doi:https://doi.org/10.3109/17435390.2012.737484.
- Patzner, R. A., R. Riehl, and R. Glechner. 1996. Die Eier heimischer Fische. 11. Plötze–Rutilus rutilus (Linnaeus, 1758) und Perlfisch - Rutilus frisii meidingeri (Heckel, 1852) (Cyprinidae). Fischökologie 9:15–26.
- Patzner, R. A., C. Weidinger, and R. Riehl. 2006. Die Eier heimischer Fische: 18. Nase-Chondrostoma nasus (Linnaeus, 1758) (Cyprinidae). Österreichs Fischerei 59:163.
- Ritz, C., F. Baty, J. C. Streibig, D. Gerhard, and Y. Xia. 2015. Dose-response analysis using R. PLOS ONE 10 (12):e0146021. doi:https://doi.org/10.1371/journal.pone.0146021.
- Römer, I., A. J. Gavin, T. A. White, R. C. Merrifield, J. K. Chipman, M. R. Viant, and J. R. Lead. 2013. The critical importance of defined media conditions in Daphnia magna nanotoxicity studies. Toxicol. Lett. 223 (1):103–08. doi:https://doi.org/10.1016/j.toxlet.2013.08.026.
- Scott, W. B., and E. J. Crossman. 1973. Freshwater fishes of Canada. In Bulletin, 184. Fisheries Research Board of Canada, Ottawa, Canada.
- Sekine, R., K. Khurana, K. Vasilev, E. Lombi, and E. Donner. 2015. Quantifying the adsorption of ionic silver and functionalized nanoparticles during ecotoxicity testing: Test container effects and recommendations. Nanotoxicol 9 (8):1005–12. doi:https://doi.org/10.3109/17435390.2014.994570.
- Stebounova, L. V., E. Guio, and V. H. Grassian. 2010. Silver nanoparticles in simulated biological media: A study of aggregation, sedimentation, and dissolution. J. Nanopart. Res 13 (1):233–44. doi:https://doi.org/10.1007/s11051-010-0022-3.
- Stefanic, P. P., M. Jarnevic, P. Cvjetko, R. Biba, S. Sikic, M. Tkalec, M. Cindric, I. Letofsky-Papst, and B. Balen. 2019. Comparative proteomic study of phytotoxic effects of silver nanoparticles and silver ions on tobacco plants. Environ. Sci. Pollut. R 26 (22):22529–50. doi:https://doi.org/10.1007/s11356-019-05552-w.
- Strenge, I., and C. Engelhard. 2016. Capabilities of fast data acquisition with microsecond time resolution in inductively coupled plasma mass spectrometry and identification of signal artifacts from millisecond dwell times during detection of single gold nanoparticles. J.Anal. Atom Spectrom 31 (1):135–44. doi:https://doi.org/10.1039/C5JA00177C.
- Villalobos, S. A., J. T. Hamm, S. J. The, and D. E. Hinton. 2000. Thiobencarb-induced embryotoxicity in medaka (Oryzias latipes): Stage-specific toxicity and the protective role of chorion. Aquat. Toxicol 48 (2–3):309–26. doi:https://doi.org/10.1016/s0166-445x(99)00032-6.
- Vogt, R., D. Mozhayeva, B. Steinhoff, A. Schardt, B. T. F. Spelz, A. Philippe, S. Kurtz, G. E. Schaumann, C. Engelhard, H. Schönherr, et al. 2019. Spatiotemporal distribution of silver and silver-containing nanoparticles in a prealpine lake in relation to the discharge from a wastewater treatment plant. Sci. Total Environ 696:134034. doi:https://doi.org/10.1016/j.scitotenv.2019.134034.
- Walters, C. R., P. Cheng, E. Pool, and V. Somerset. 2016. Effect of temperature on oxidative stress parameters and enzyme activity in tissues of Cape River crab (Potamanautes perlatus) following exposure to silver nanoparticles (AgNP). J. Toxicol. Environ. Health Part A 79 (2):61–70. doi:https://doi.org/10.1080/15287394.2015.1106357.
- Wanzenböck, J. In press. Rearing and stocking of Coregonids: A comparison of aquaculture practices in Eurasia and North America. Adv. Limnol.
- Wickham, H. 2009. ggplot2: Elegant graphics for data analysis. New York: Springer-Verlag.
- Wu, Y., Q. Zhou, H. Li, W. Liu, T. Wang, and G. Jiang. 2010. Effects of silver nanoparticles on the development and histopathology biomarkers of Japanese medaka (Oryzias latipes) using the partial-life test. Aquat. Toxicol 100 (2):160–67. doi:https://doi.org/10.1016/j.aquatox.2009.11.014.
- Yang, X., A. P. Gondikas, S. M. Marinakos, M. Auffan, J. Liu, H. Hsu-Kim, and J. N. Meyer. 2012. Mechanism of silver nanoparticle toxicity is dependent on dissolved silver and surface coating in Caenorhabditis elegans. Environ. Sci. Technol 46 (2):1119–27. doi:https://doi.org/10.1021/es202417t.
- Zhang, Y., Y. Chen, P. Westerhoff, and J. Crittenden. 2009. Impact of natural organic matter and divalent cations on the stability of aqueous nanoparticles. Water Res 43 (17):4249–57. doi:https://doi.org/10.1016/j.watres.2009.06.005.
- Zook, J. M., M. D. Halter, D. Cleveland, and S. E. Long. 2012. Disentangling the effects of polymer coatings on silver nanoparticle agglomeration, dissolution, and toxicity to determine mechanisms of nanotoxicity. J. Nanopart. Res 14 (10):1165. doi:https://doi.org/10.1007/s11051-012-1165-1.