ABSTRACT
Difficulties related to the obtainment of stem/progenitor cells from skeletal muscle tissue make the search for new sources of myogenic cells highly relevant. Alveolar mucosa might be considered as a perspective candidate due to availability and high proliferative capacity of its cells. Human alveolar mucosa cells (AMC) were obtained from gingival biopsy samples collected from 10 healthy donors and cultured up to 10 passages. AMC matched the generally accepted multipotent mesenchymal stromal cells criteria and possess population doubling time, caryotype and immunophenotype stability during long-term cultivation. The single myogenic induction of primary cell cultures resulted in differentiation of AMC into multinucleated myotubes. The myogenic differentiation was associated with expression of skeletal muscle markers: skeletal myosin, skeletal actin, myogenin and MyoD1. Efficiency of myogenic differentiation in AMC cultures was similar to that in skeletal muscle cells. Furthermore, some of differentiated myotubes exhibited contractions in vitro. Our data confirms the sufficiently high myogenic potential and proliferative capacity of AMC and their ability to maintain in vitro proliferation-competent myogenic precursor cells regardless of the passage number.
Introduction
Aging as well as some diseases might disrupt regenerative capacity of skeletal muscle tissueCitation1 with development of muscular dystrophies, neuromuscular diseases, sarcopenia, and other myopathies.Citation2 Some of these diseases are potentially lethal, standard methods of their treatment, nevertheless, are often ineffective.Citation3 The use of myogenic cells is considered to be one of the most promising approaches to treatment of muscular disorders.Citation4
Regeneration of skeletal muscle tissue strongly relies on satellite cells (SC), residing in the niche between sarcolemma and basal lamina of mature skeletal muscle fibers.Citation5 The best approach to the treatment of skeletal tissue diseases is considered to be the transplantation of SCs.Citation6,7 Their use, however, has several limitations: isolation of SCs is difficult, SCs are relatively rare population and account for only a small part of skeletal muscle mass, moreover, transplantation causes significant loss of their myogenic properties.Citation1,8,9,10 Thus, the search for new sources of cells with myogenic potential is a relevant problem.Citation11
Apart from SCs, multipotent mesenchymal stromal cells (MMSC) are considered as a tool for muscle tissue regeneration. MMSC can be differentiated into three orthodox directions: osteo- chondro- and adipogenic, and, under specific conditions, into endothelial and neuronal directions.Citation12,13,14,15,16,17 MMSC can be obtained from bone marrow, adipose and other types of connective tissues.Citation12,18 Also it was shown, that MMSC could be differentiated into myogenic direction716 and are able to restore damaged myofibers.Citation10 It was described earlier that MMSC derived from different tissues can possess various colony-forming unit and differentiation efficiency as well as different proliferative capacity.Citation19,20,21 Another important issue is availability of the source tissue, namely invasiveness of manipulation and risk of complications, related to sample procurement.Citation22 Gingival mucosa is one of promising sources of MMSC due to availability and minimally invasiveness of its procurement and ability of gingival mucosa wounds to heal without formation of scar.Citation23 Gingiva derived MMSC possess some specific properties, which make them very attractive candidate for regenerative medicine.Citation24,25 Namely, they have strong immunomodulatory properties,Citation25 ability to differentiate into neuronal direction due to its development from neural crest.Citation26,27 Moreover, the amount of MMSC able to multipotent differentiation is higher in gingival mucosa compared to other tissues.Citation28,29,30 In primary cultures of gingival fibroblasts up to 90% of cells possess “stemness” markers and 40–70% of cells express pluripotency genes such as Oct4, Sox2, NanogCitation28 and active telomerase.Citation29
It is well known that gingiva can be visually divided into several zones: free gingiva, attached gingiva, alveolar mucosa etc. ().Citation31 It is not specified in most research articles which anatomical region was used for the obtainment of gingival MMSC. Our work is focused on attached gingiva – the source of attached gingiva derived MMSC (AGC), and alveolar mucosa – the source of alveolar mucosa derived MMSC (AMC). In this study we have shown in the first time that AMC, but not AGC, can differentiate into myogenic direction.
Figure 1. Characterization of mesenchymal cells derived from human Alveolar Mucosa. Typical data of one AMC culture is presented. Analysis of all investigated AMC has shown no significant deviations for cultures obtained from ten different patients. (A) Anatomical regions of human gingiva. The sources of Alveolar Mucosa (AMC) Attached Gingiva (AGC) and free gingiva cells are indicated. (B) Protein profiling of AMC. FACScan analysis data of different protein markers expression in AMC at early passages (2–3p). (C) Expression of positive mesenchymal markers in AMC*. (D) Immunofluorescence analysis of mesenchymal markers confirming morphology of investigated AMC, cell nuclei stained with DAPI (Bars 25 μm)*. * - Data of negative (unexpressed in AMC) markers is available in Suppl.1.
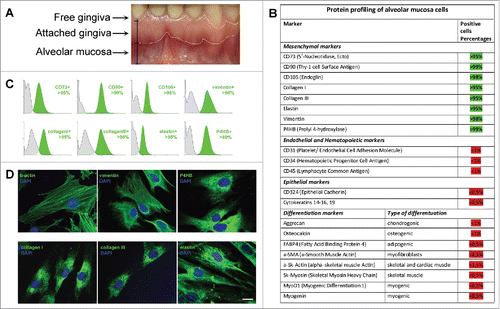
Results
AMC and AGC were obtained by enzymatic digestion of the biopsies of 10 donors. Main characteristics of AGC were described in details in our previous work.Citation21 First of all, to define characteristics of AMC and confirm their relationship to MMSC, we investigated their immunophenotype, morphology and differentiation potential. Cell adherence to plastic was confirmed on the first passage. All AMC cultures obtained from 10 donors showed similar protein profiles and cell surface antigens in sequence of 10 passages. According to flow cytometry data AMC expressed typical MMSC markers (CD73, CD90, CD105) and intracellular fibroblast proteins (collagen type I and III, elastin) (). Additionally, cells actively expressed b-actin, vimentin and prolyl 4-hydroxylase (P4HB) (). At the same time, no expression of epithelial (CD324, cytokeratins 14, 15, 16, and 19), endothelial and haematopoietic (CD31, CD34, CD45), skeletal and cardiac muscle (skeletal muscle actin (sk-actin), skeletal muscle myosin heavy chain (sk-myosin), MyoD1, myogenin), myofibroblasts (α-smooth muscle actin (αSMA)) markers and markers of osteogenic (ostecalcin), adipogenic (fatty acid binding protein 4 (FABP4)) and chondrogenic (aggrecan) differentiation was revealed (, Suppl.1A, B). There were no detectable differences when comparing different donors and/or passages. Mesenchymal morphology of all AMC cultures was confirmed by immunocytochemical analysis () with typical staining and localization of intracellular proteins. Further analysis demonstrated that AMC from 10 donors kept similar fibroblast-like morphology and pattern of f-actin fibers in cytoskeleton during cultivation up to passage 10 (). There were no statistically significant differences in population doubling time of all AMC cultures at different passages. In particular, population doubling time was 36 ± 1.2 h and 38 ± 1.2 h at passage 1 and 10, correspondingly. Cytogenetic analysis showed no chromosomal abnormalities both at passage 1 and 10 (). In addition, ten samples of AMC from different donors maintained the ability to differentiate in osteogenic, adipogenic, and chondrogenic lineages up to passage 8 when cultured in media supplemented with the corresponding induction factors. Immunofluorescence analysis showed expression of chondro- (aggrecan), osteo- (osteocalcin) and adipogenic (FABP4) markers after induction of differentiation (). Expression of specific markers of differentiation was not observed when culturing AMC under normal conditions.
Figure 2. Invariability of AMC morphology and karyotype stability at passages 1 and 10. Typical data of one of ten AMC cultures is presented; similar results have been obtained for other cultures. (A) Phase contrast microscopy of AMC culture at passages 1 and 10 (Bars 50 μm). (B) Immunofluorescence analysis of f-actin bundles (TRITC-phalloidin-red) of AMC at passages 1 and 10, cell nuclei stained with DAPI (Bars 50 μm). (C) Immunofluorescence analysis of morphology of interphase nuclei (stained with DAPI) of AMC at passages 1 and 10 (Bars 50 μm). (D) AMC's karyotyping at passages 1 and 10. G-band analysis of metaphase chromosomes.
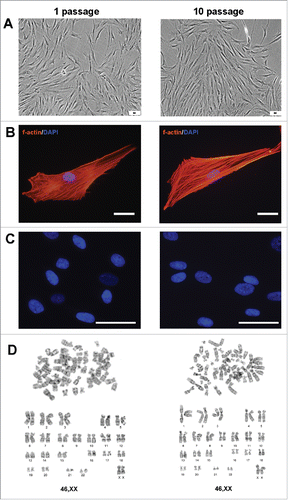
Figure 3. Abilities of AMC to differentiate into chondro-, osteo- and adipogenic directions. Immunofluorescence analysis of chondro- (aggrecan), osteo- (osteocalcin) and adipogenic (FABP4) markers under normal (upper panel) and differentiation inducting (lower panel) conditions, cell nuclei stained with DAPI (Bars 50 μm). Typical data of one of ten AMC cultures is presented; all ten tested AMC cultures were able to differentiate into three lineages.
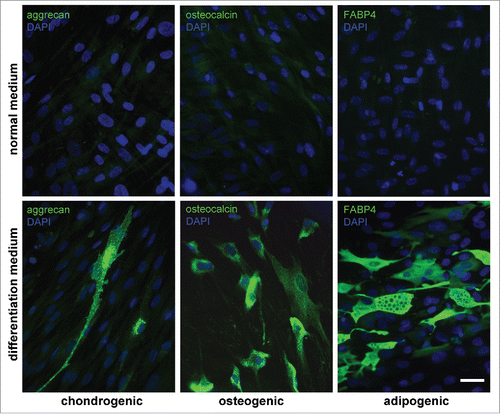
Furthermore, supplementation of culture medium with Transforming growth factor beta 1 (TGF-β1) promoted α-SMA expression in all primary AMC cultures followed by myofibroblasts formation (). After myofibroblastic conversion cells changed their morphology and increased in size. At the same time, induction of actin stress fibers formation was revealed (). The amount of myofibroblasts was dependent on the time of AMC culture exposition in TGF-β1 supplemented medium. AMC cultures treated with TGF-β1 for 5 consecutive passages showed significantly higher rate of α-SMA expression in comparison to cells treated for 72 h () and reached up to 90% percentages of all cells.
Figure 4. Ability of AMC to TGBβ1-induced myofibroblast differentiation. (A) Immunofluorescence analysis of α-smooth muscle actin (αSMA) (green) of AMC after 72 h of permanent TGFβ1 treatment and 5 passages of permanent TGFβ1 treatment, cell nuclei stained with DAPI (Bars 25 μm). Typical immunofluorescence staining of one of ten AMC cultures is presented. (B) FACScan analysis of α-smooth muscle actin (αSMA) (green) after 72 h of permanent TGFβ1 treatment and 5 passages of permanent TGFβ1 treatment of AMC culture presented in section 4A. (C) Percentage of αSMA-positive cells after 72 h of permanent TGFβ1 treatment and 5 passages of permanent during passaging TGFβ1 treatment. Average data for ten AMC cultures is presented (Mean ± SD).
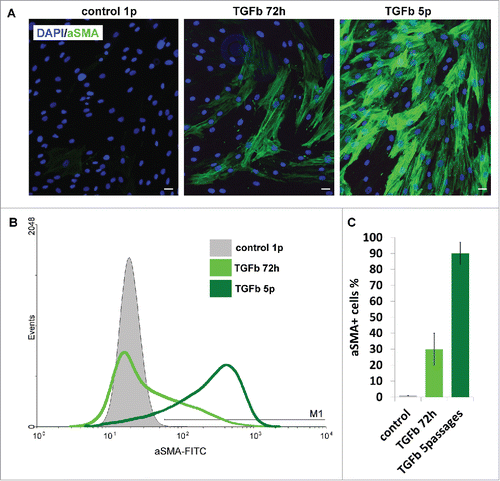
Possibility of induction of myogenic differentiation in vitro was demonstrated for undifferentiated AMC obtained from all donors both at early and late passages (from 1 to 10). For that purpose we supplied confluent cultures with low glucose DMEM and 2% horse serum without passaging which promote differentiation of myogenic cells to myotubes.Citation32,33,34,35 Cells alignment and formation of multinuclear myotubes were observed in AMC cultures 5–10 days after switching from standard cultivation medium to induction medium (). Moreover, some of differentiated myotubes exhibited the contraction in vitro. (). Myotubes formation was not detected in control AMC cultures without induction conditions. The expression of striated muscles' markers: sk-myosin, sk-actin, myogenin and MyoD1 was revealed using immunocytochemical staining in elongated multicellular myotube fibrils (). The specific striated pattern of sk-myosin and sk-actin fibers was detected at higher magnification ().
Figure 5. Microscopy analysis of myogenic proteins in AMC differentiated in myogenic direction. Typical data of one AMC culture is presented. All investigated AMC cultures obtained from ten different patients were able to differentiate in myogenic direction. (A) AMC derived myotubes phase contrast microscopy (Bars 25 μm). (B) AMC derived myotubes DIC microscopy. Suppl.2 myotube contraction video. (C) Immunofluorescence analysis of skeletal myosinandactin (red), myogenic transcriptional factors myogenin and MyoD1 (green), cell nuclei stained with DAPI (Bars 25 μm).
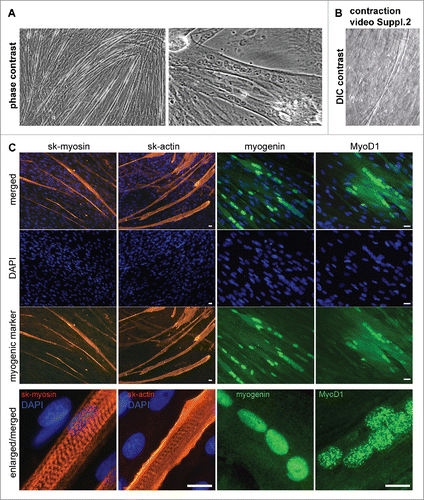
Additional experiments showed that anatomical localization of gingival mucosa for cells obtainment plays an important role. The ability to differentiate in myogenic direction was possessed only by AMC but not AGC ( and ). The results were reproducible in several experiments when using AMC and AGC from 10 donors at different passages. Percentage of myotubes covered area in differentiated AMC cultures was similar to that in cells derived from skeletal muscle tissue (SMC), whereas induction of myogenic differentiation in AGC did not result in myotubes production (). To confirm myogenic differentiation of AMC, we investigated the expression of genes related to myogenesis in these cells by reverse transcription–polymerase chain reaction (RT-PCR) in addition to skeletal actin and myosin staining of all donors. In differentiated AMC and SMC, but not AGC, expression of myogenin and MyoD1 were significantly higher than in undifferentiated primary cultures (). It is worth noting that for myogenic differentiation of SMC we used similar conditions as were described for AMC and AGC.
Figure 6. Comparison of AGC, AMC and SMC primary myogenic potential. (A) Phase contrast microcopy and simultaneous skeletal actin and myosin fluorescent staining of primary and induced in myogenic direction AGC, AMC and SMC cultures. One from each typical AGC, AMC and SMC cultures immunofluorescence staining are presented (Bars 100 μm). (B) Analysis of myotube/sk-actinandmyosin-positive areas of primary and induced in myogenic direction AGC, AMC and SMC cultures. Average data for ten AGC and AMC cultures obtained from the same donors and six SMC cultures obtained from other donors are presented (Mean ± SD). (C) The result of one of the representative End-point PCR experiments of MyoD1 and myogenin myogenic factors of one from each AGC, AMC and SMC cultures. α-tubulin mRNA was analyzed as loading control. (D) Relative quantities of MyoD1 and myogenin mRNAs estimated by Real-time qPCR. α-tubulin gene was used for data normalization. Average data for ten AGC and AMC cultures obtained from the same donors and six SMC cultures obtained from other donors are presented (Mean ± SD).
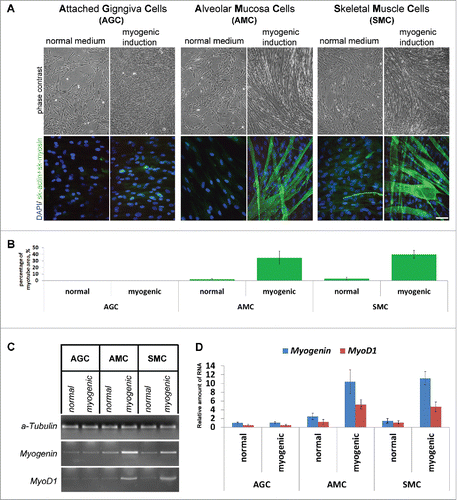
The single induction of myogenic differentiation in AMC cultures at different passages led to myotubes formation confirmed by detection of remarkable increase of myogenin RNA amount in cells () as well as percentage of myotubes covered area (). The efficiency of myogenic differentiation remained constant at different passages in all AMC cultures and was same to that in SMC cultures in regard to myogenin expression and percentage of myotubes covered area ().
Figure 7. Comparison of myogenic differentiation potential of AMC and SMC at different passages, failure of repeated myogenic differentiation of both cell types. (A) The result of one of the representative End-point PCR experiments of myogenin expression, α-tubulin mRNA was analyzed as loading control. Typical PCR results for one from each AMC and SMC cultures are presented. (B) Relative quantities of myogenin mRNAs estimated by Real-time qPCR. α-tubulin gene was used for data normalization. Average data for ten AMC cultures and six SMC cultures are presented (Mean ± SD). (C) Analysis of myotube/sk-actinandmyosin-positive areas of primary and induced in myogenic direction AMC and SMC at different passages. Average data for ten AMC cultures and six SMC cultures are presented (Mean ± SD).
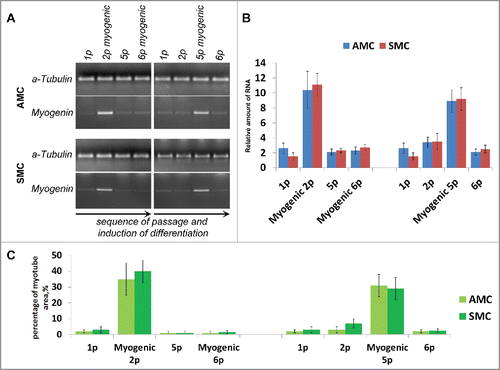
To further investigate the ability of AMC to differentiate in myogenic direction we performed experiment with serial inductions of myogenic differentiation. For that purpose confluent AMC cultures at passage 2 were supplied with low glucose DMEM and 2% horse serum and incubated for 10 days. After myotubes formation culture medium was switched back to DMEM/F12 with 20% FBS, cells were passed at density of 3×104 cells/cm2 and cultivated for two more passages. Myotubes showed no adherence to the plastic and were removed from culture during medium changing and passaging. Second induction of myogenic differentiation in the same cells culture at passage 6 did not led to production of new myotubes ().
Discussion
Search for cell populations with the ability of active proliferation and differentiation into certain direction is an important task of regenerative medicine.Citation36,37 In present study a new source of MMSC able to differentiate into myogenic direction is described.
The investigation of cell cultures isolated from alveolar mucosa (AMC) demonstrated that cells match the generally accepted MMSC criteria.Citation38 First of all, immunophenotypical analysis of AMC revealed expression of cell surface antigens and intracellular proteins which are relevant to MMSC. Expression of specific markers of differentiated cells was not detected in all primary cell cultures. Secondly, the ability of AMC to differentiate into canonical directions (osteogenic, adipogenic, and chondrogenic) was confirmed both at early and late passages. Apart from three orthodox directions of cell differentiation, the ability of AMC cultures to differentiate into myofibroblasts was demonstrated. It has been found, that the extent of myofibroblast activation depended on TGF-β1 stimulation time and almost all cells AMC culture can be conversed to myofibroblasts. Furthermore, in terms of safety, сells morphology and karyotype were stable during cultivation up to passage 10 that is very important for possibility of their use in cell therapy. These results agree with other studies devoted to gingival MMSC that reported similar phenotype characteristics, differentiation potential and stable genomic behavior.Citation19,39,40
Also, we determined high proliferative capacity of AMC which remained constant from primary to long-term cultures. It should be noted that the population doubling time for bone marrow-derived MMSC reaches only 55 ± 3 hours under similar cultivation conditions.Citation41 Therefore AMC possess higher proliferative potential in comparison with bone marrow-derived MMSC. These results are in agreement with the study of Tomar et al. that reported resemblant population doubling time ranges for bone marrow and gingival MMSC.Citation39 Taking into account advantages of the gingiva as a cell source (a very accessible donor tissue) and peculiar properties of AMC (higher proliferative potential, caryotype and immunophenotype stability during long-term cultivation), this type of cells could be considered as an attractive candidate for application in regenerative medicine.
To determine whether AMC and AGC have an ability to differentiate in myogenic direction, confluent cultures were placed under the induction conditions. Myogenic induction of all primary AMC cultures resulted in differentiation of cells into multinucleated myotubes. Myotubes formation was not registered in all AGC and control (not differentiated) cultures. The efficiency of myogenic differentiation was high regardless of the donor or passage number, i.e., even at passage 10 the myotubes covered area was approximately 30–40%. Interestingly, Dezawa et al.Citation42 revealed the same fusion index in myogenic subpopulation of bone marrow-derived MMSC (MyoD+, myogenin+) cultured under special conditions which led to high efficiency of myogenic differentiation. Similar efficiency of myogenic differentiation of all AMC cultures at all passages suggests that AMC contain subpopulation of undifferentiated proliferation-competent cells with myogenic potential that could be easily obtained and expanded in vitro on a therapeutic scale in a short time period. To investigate whether AMC culture contains limited amount of progenitors or all cells possess myogenic potential we performed serial inductions of differentiation in same AMC culture. Repeated attempt of myogenic induction did not caused formation of new myotubes. Probably, after differentiation this subpopulation of cells loose ability to proliferate what is similar to SMC myogenic differentiation pattern. Despite this fact myogenic progenitor cells can be easily expanded under undifferentiated growth conditions.
Hereby, our data confirms the sufficiently high myogenic potential and proliferative capacity of AMC and their ability to maintain in vitro proliferation-competent myogenic precursor cells regardless of the passage number. Described features of AMC along with characteristics of cells source allows to obtain in vitro large amounts of cells with required characteristics in a short time period. This type of cells could be considered as an attractive candidate for application in regenerative medicine including treatment of muscular dystrophic diseases.
Materials and methods
Biopsy of gingiva
After the healthy donors (n = 10, 5 women and 5 men, mean age 36 ± 5 years) signed the informed consent, biopsies of the alveolar mucosa from the retromolar area and attached gingiva from the distal mandibular molar level were performed under local anesthesia (Sol. Articaini 40 mg/ml, 1.7 ml) (). Biopsy samples (2–3 mm3) were placed in sterile medium containing α-MEM (Sigma), 2% FBS (HyClone), penicillin (200 U/mL), streptomycin (200 mg/mL), and amphotericin (200 U/mL) (Stem Cell Technologies), labeled and delivered at +4°С to laboratory within 2 hours.
Biopsy of skeletal muscle
After the donors (n = 6, 3 women and 3 men, mean age 63 ± 3 years) signed the voluntary informed consent, the biopsy specimens of the quadriceps femoris muscle were taken during standard surgical treatment of the atherosclerotic vascular lesions of lower limbs. Biopsy samples (1–1.5 cm3) were placed in sterile medium containing α-MEM (Sigma), 2% FBS (HyClone), penicillin (200 U/mL), streptomycin (200 mg/mL), and amphotericin (200 U/mL) (Stem Cell Technologies), labeled and delivered at +4°С to laboratory within 2 hours.
Isolation and cultivation of cells
All biopsy samples were processed using similar protocol as follows. AMC were isolated from alveolar mucosa (N = 10), AGC – from attached gingiva (N = 10) and SMC – from skeletal muscle tissue (N = 6). Biopsy sample was washed with α-MEM (Sigma) supplemented with gentamicin (50 µg/mL) (Sigma). A sample was transferred into 15 mL tube in α-MEM supplemented with 10% FBS (HyClone), gentamicin (20 µg/mL), 0.05% solution of collagenase type II (Sigma) and incubated for 12 hours at 37°С. Before enzymatic digestion skeletal muscle samples were minced. After that, cell suspension was centrifuged at 200 g for 10 min. The cell pellet was resuspended in culture medium DMEM/F12 (HyClone) supplemented with 20% FBS (HyClone), gentamicin (20 µg/mL) and plated in culture flasks (NUNC) at density of 3–5×104 cells/cm2. Cells were cultured at 37°С, humidity 95% and 5% СО2 with medium change every 3 days. Cell cultures were passaged at 50% confluence. Visual observation of culture growth and cell morphology was conducted by phase contrast microscopy. Cell cultures at passages 1 to 10 were used for research experiments.
FACScan analysis
Cell suspension was fixed with 4% solution of paraformaldehyde (PFA) in phosphate–buffered saline (pH = 7.4). Fluorescently labeled antibodies against CD31FITC (Cat#560984 BDPharmingen), CD34PE (Cat#550761 BDPharmingen), CD45FITC (Cat#555482 BDPharmingen), CD73PE (Cat#550257 BDPharmingen), CD90APC (Cat#559869 BDPharmingen), CD324FITC (Cat# 612130 BDPharmingen), pan-cytokeratinsPE (14, 15, 16, and 19) (Cat# 550953 BDPharmingen), CD105Alexa488 (Cat#MHCD10520 LifeTechnologies) were used according to manufacturer's recommendations. For FACS analysis of other proteins cell suspensions were fixed with 2% PFA for 10 min and then 10 min with ice-cold methanol. After that cells were incubated with primary antibodies against human a-smooth muscle actin (α-SMA) (Cat#M0851 DAKO), collagen-I (Cat#2150–0001 Bio-Rad), collagen-III (Cat#2150–0100 Bio-Rad), elastin (Cat#4060–1060 Bio-Rad), vimentin (Cat#M0725 DAKO), prolyl 4-hydroxylase (P4HB) (Cat#NB300–517 Novus), aggrecan (Cat#AF1220 R&D Systems), osteocalcin (Cat#MAB1419 R&D Systems), fatty acid binding protein 4 (FABP4) (Cat#AF1443 R&D Systems), skeletal muscle actin (sk-actin) (Cat#M0851 DAKO), skeletal muscle myosin (sk-myosin) (Cat#18–0105 LifeTechnologies), MyoD1 (Cat#M3512 DAKO), myogenin (Cat#M3559 DAKO) followed by FITC-conjugated animal-corresponding antibodies (LifeTechnologies). Data was acquired and analyzed on FACS Cantoтм II with FACSDivaтм software (BD, USA).
Cytogenetic analysis
Karyotyping was performed by standard techniques. Cells were fixed and treated with Colcemid (0,05 mkg/ml) for 1 hour followed by treatment with 0,56 KCL (37°C) for 1 hour. Air-dried chromosome slides were prepared by routine methods. G-banding was performed using trypsin-Giemsa technique. Karyotypes were described according to the ISCN.Citation43
Osteogenic, adipogenic and chondrogenic differentiation
Human Mesenchymal Stem Cell Functional Identification kit (R&D Systems) was used for osteo-, adipo- and chondrogenic differentiation of cells. All manipulations were performed according to the manufacturer's protocol. Expression of osteocalcin, FABP4 and aggrecan was detected by immunocytochemical analysis using primary monoclonal antibodies against human osteocalcin, FABP4 and aggrecan provided in kit (R&D Systems) and secondary fluorescein-labeled (FITC) antibodies (LifeTechnologies).
Myofibroblast differentiation
To activate conversion to myofibroblasts cells were plated at density of 5×104 cells/cm2 in DMEM (HyClone) supplemented with 10% FBS (HyClone), 10 ng/mL transforming growth factor β1 (TGF-β1) (PeproTech). Analysis of TGB-β1-induced myofibroblasts was conducted after 3 days of cell incubation and after 5 passages in the TGB-β1 presence.
Myogenic differentiation
To induce myogenic differentiation, cells were seeded at density of 5×105 cells/cm2 and cultured in DMEM/F12 supplemented with 20% FBS (HyClone) until 90–100% confluent monolayer was formed. Then culture medium was replaced with low glucose DMEM (HyClone) supplemented with 2% horse serum (BioInd) and cells were incubated for 5–10 days at 37°С, humidity 95% and 5% СО2 until myotube-like structures formed.
Immunofluorescent microscopy
For fluorescent analysis of actin filaments, detection osteo-, chondroblasts and adipocytes, cells were fixed with 4% PFA. f-Actin was stained with fluorescent TRITC-labeled phalloidin (Cat#P1951 Sigma). Osteo-, chondro- and adipo- differentiated cells were stained as described above. Detection of b-actin (Cat#MCA5775GA Bio-Rad), vimentin (Cat#M0725 DAKO), collagen I (Cat#2150–0001 Bio-Rad), collagen III (Cat#2150–0100 Bio-Rad), elastin (Cat#4060–1060 Bio-Rad), P4HB (Cat#NB300–517 Novus), α-SMA (Cat#M0851 DAKO) and myogenic markers MyoD1 (Cat#M3512 DAKO), myogenin (Cat#M3559 DAKO), sk-actin (Cat#M0874 DAKO) and sk-myosin (Cat#18–0105, LifeTechnologies) were performed using primary untagged and secondary Alexa488,546-labeled (LifeTechnologies) antibodies. Cells were fixed with ice-cold methanol. Nuclei were stained with DAPI (Cat#32670 Sigma). Images were acquired using fluorescent Axioplan 200 microscope with Axiocam HRc camera and AxioVision software (Carl Zeiss, Germany).
Estimation of the efficiency of myogenic differentiation
To estimate the efficiency of myogenic differentiation, cells seeded on slide glasses were fixed with ice-cold methanol. The cells were simultaneously incubated with the primary murine antibodies specific to human sk-myosin (Cat#18–010, LifeTechnologies) and sk-actin (Cat#M0874 DAKO). Then the cells were incubated with the secondary FITC-labeled rabbit antibodies against murine antibodies (LifeTechnologies, USA). Images were visualized and photographed by means of Axioplan 2 microscope with Axiocam HRc camera, and AxioVision software (Carl Zeiss, Germany). FITC-stained areas were separated, green filled, converted to black and white BMP format and a ratio between the specifically stained area and the total field area was estimated on photographs using ImageJ software (National Institutes of Health, USA) (Suppl. 3). 25 independent fields were analyzed individually for each case and the data was expressed as mean values for all patients.
Reverse transcription-PCR assay
Total mRNA was isolated with SV Total RNA Isolation System (Promega) according to the manufacturer's protocols. To detect the corresponding gene expression using endpoint PCR, the following primers and conditions were used:
MyoD1: F 5′-AGCACTACAGCGGCGACT-3′,
R 5′-GCGACTCAGAAGGCACGTC-3′, 35 amplification cycles;
myogenin: F 5′-GGGGAAAACTACCTGCCTGTC-3′,
R 5′-AGGCGCTCGATGTACTGGAT-3′, 35 amplification cycles;
α-tubulin: F 5′-GTTGGTCTGGAATTCTGTCAG-3′,
R 5′-AAGAAGTCCAAGCTGGAGTTC-3′, 30 amplification cycles;
initial denaturation 95°C 60 s; amplification: denaturation 95°C 30 s, annealing 60°C 30 s (for all primers), extension 72°C 30 s; final extension 5 min at 72°C.
The detection of PCR bands was performed using Chemi-Smart 3000 Imaging System (Vilber Lourmat). For Real-time qPCR analysis IQ5 Biorad PCR Detection System (Biorad) was used. Real-time PCR was performed using 2.5x SYBR Green real-time PCR mix (Syntol, Russia) according to manufacturer's protocol with addition of 0.4 µM of primers (forward, reverse) and 50 ng of cDNA and DEPC-treated water to the final volume of 25 µL. PCR conditions were as follows: 94°C for 5 min, followed by 40 cycles of amplification: 94°C for 10 s, 60°C for 10 s, and 72°C for 20s. For all genes the same primers in qPCR were used. The PCR products were checked for specificity with agarose gel electrophoresis and melt-curve analysis. Data was analyzed with CFX Manager Software (BioRad). Alpha-tubulin gene was used for data normalization. Samples were collected from 3 independent cultures. Data was analyzed based on 2−ΔΔCt method.
Statistical analysis
All experiments were repeated 3 times for each of 10 cultures. Mean values and standard deviations were calculated, the assumption of normality was evaluated using the Shapiro-Wilk test, and Mann–Whitney U test was used to compare two independent groups, differences were considered to be statistically significant at p<0.05. Statistical analyses were performed using GraphPad Prism 5.0 software (GraphPad Software Inc., USA).
Disclosure of potential conflicts of interest
No potential conflicts of interest were disclosed.
1284714_Supplemental_Material.zip
Download Zip (19.6 MB)Funding
The study was financially supported by Russian Science Foundation (grant # 14-25-00166).
References
- Meregalli M, Farini A, Sitzia C, Torrente Y. Advancements in stem cells treatment of skeletal muscle wasting. Front Physiol 2014; 5(48):1-12; PMID:24478714
- McCullagh К, Perlingeiro R. Coaxing stem cells for skeletal muscle repair. Adv Drug Deliv Rev 2015; 84:198-207; PMID:25049085; http://dx.doi.org/10.1016/j.addr.2014.07.007
- Shi Х, Garry DJ. Muscle stem cells in development, regeneration, and disease. Genes Dev 2006; 20(13):1692-708; PMID:16818602; http://dx.doi.org/10.1101/gad.1419406
- Cerletti M, Jurga S, Witczak CA, Hirshman MF, Shadrach JL, Goodyear LJ, Wagers AJ. Highly efficient, functional engraftment of skeletal muscle stem cells in dystrophic muscles. Cell 2008; 134(1):37-47; PMID:18614009; http://dx.doi.org/10.1016/j.cell.2008.05.049
- Carlson ME, O'Connor MS, Hsu M, Conboy IM. Notch signaling pathway and tissue engineering. Front Biosci 2007; 12:5143-56; PMID:17569636; http://dx.doi.org/10.2741/2554
- Price F, Kuroda D, Rudnicki MA. Stem cell based therapies to treat muscular dystrophy. Biochim Biophys Acta 2007; 1772(2):272-83; PMID:17034994; http://dx.doi.org/10.1016/j.bbadis.2006.08.011
- Gussoni E, Blau HM, Kunkel LM. The fate of individual myoblasts after transplantation into muscles of DMD patients. Nat Med 1997; 3(9):970-7; PMID:9288722; http://dx.doi.org/10.1038/nm0997-970
- Farini A, Razini P, Erratico S, Torrente Y, Meregalli M. Cell based therapy for Duchenne muscular dystrophy. J Cell Physiol 2009; 221(3):526-34; PMID:19688776; http://dx.doi.org/10.1002/jcp.21895
- Meligy FY, Shigemura K, Behnsawy HM, Fujisawa M, Kawabata M, Shirakawa T. The efficiency of in vitro isolation and myogenic differentiation of MSCs derived from adipose connective tissue, bone marrow, and skeletal muscle tissue. In Vitro Cell Dev Biol Anim 2012; 48(4):203-15; PMID:22396125; http://dx.doi.org/10.1007/s11626-012-9488-x
- Ferrari G, Cusella-De Angelis G, Coletta M, Paolucci E, Stornaiuolo A, Cossu G, Mavilio F. Muscle regeneration by bone marrow-derived myogenic progenitors. Science 1998; 279(5356):1528-30; PMID:9488650; http://dx.doi.org/10.1126/science.279.5356.1528
- Dezawa M, Ishikawa H, Hoshino M, Itokazu Y, Nabeshima Y. Potential of bone marrow stromal cells in applications for neuro-degenerative, neuro-traumatic and muscle degenerative diseases. Curr Neuropharmacol 2005; 3(4):257-66; PMID:18369401; http://dx.doi.org/10.2174/157015905774322507
- Fridenshtein AIa, Chailakhin RK, Gerasimov IuV. Proliferative and differentiation potentials of skeletogenic bone marrow colony-forming cells. Tsitologiia 1986; 28(3):341-9; PMID:3521008
- Caplan AI. Mesenchymal stem cells. J Orthop Res 1991; 9:641-50; PMID:1870029; http://dx.doi.org/10.1002/jor.1100090504
- Bianco P, Robey PG, Simmons PJ. Mesenchymal stem cells: revisiting history, concepts, and assays. Cell Stem Cell 2008; 2(4):313-9; PMID:18397751; http://dx.doi.org/10.1016/j.stem.2008.03.002
- Hematti P. Mesenchymal stromal cells and fibroblasts: a case of mistaken identity? Cytotherapy 2012; 14(5):516-21; PMID:22458957; http://dx.doi.org/10.3109/14653249.2012.677822
- Zuk PA, Zhu M, Mizuno H, Huang J, Futrell JW, Katz AJ, Benhaim P, Lorenz HP, Hedrick MH. Multilineage cells from human adipose tissue: implications for cell-based therapies. Tissue Eng 2001; 7(2):211-28; PMID:11304456; http://dx.doi.org/10.1089/107632701300062859
- Oswald J, Boxberger S, Jørgensen B, Feldmann S, Ehninger G, Bornhäuser M, Werner C. Mesenchymal stem cells can be differentiated into endothelial cells in vitro. Stem Cells 2004; 22(3):377-84; PMID:15153614; http://dx.doi.org/10.1634/stemcells.22-3-377
- Robey PG. Cell Sources for Bone Regeneration: The Good, the Bad, and the Ugly (But Promising). Tissue Engineering 2011; 17(6):423-30; PMID:21797663; http://dx.doi.org/10.1089/ten.teb.2011.0199
- Santamaria S, Sanchez N, Sanz M, Garcia-Sanz JA. Comparison of periodontal ligament and gingiva-derived mesenchymal stem cells for regenerative therapies. Clin Oral Investig 2016; [ Epub ahead of print]; PMID:27270903; http://dx.doi.org/10.1007/s00784-016-1867-3
- Sekiya I, Larson BL, Smith JR, Pochampally R, Cui JG, Prockop DJ. Expansion of human adult stem cells from bone marrow stroma: conditions that maximize the yields of early progenitors and evaluate their quality. Stem Cells 2002; 20(6):530-41; PMID:12456961; http://dx.doi.org/10.1634/stemcells.20-6-530
- Zorin VL, Komlev VS, Zorina AI, Khromova NV, Solovieva EV, Fedotov AY, Eremin II, Kopnin PB. Octacalcium phosphate ceramics combined with gingiva-derived stromal cells for engineered functional bone grafts. Biomed Mater 2014; 9(5):055005; PMID:25167539; http://dx.doi.org/10.1088/1748-6041/9/5/055005
- Sakaguchi Y, Sekiya I, Yagishita K, Muneta T. Comparison of human stem cells derived from various mesenchymal tissues: superiority of synovium as a cell source. Arthritis Rheum 2005; 52(8):2521-29; PMID:16052568; http://dx.doi.org/10.1002/art.21212
- Mitrano TI, Grob MS, Carrión F, Nova-Lamperti E, Luz PA, Fierro FS, Quintero A, Chaparro A, Sanz A. Culture and characterization of mesenchymal stem cells from human gingival tissue. J Periodontol 2010; 81(6):917-25; PMID:20450355; http://dx.doi.org/10.1902/jop.2010.090566
- Zhang QZ, Nguyen AL, Yu WH, Le AD. Human oral mucosa and gingiva: a unique reservoir for mesenchymal stem cells. J Dent Res 2012; 91(11):1011-8; PMID:22988012; http://dx.doi.org/10.1177/0022034512461016
- Fournier BP, Larjava H, Häkkinen L. Gingiva as a source of stem cells with therapeutic potential. Stem Cells Dev 2013; 22(24):3157-77; http://dx.doi.org/10.1089/scd.2013.0015
- Xu X, Chen C, Akiyama K, Chai Y, Le AD, Wang Z, Shi S. Gingivae contain neural-crest- and mesoderm-derived mesenchymal stem cells. J Dent Res 2013; 92(9):825-32; PMID:23867762; http://dx.doi.org/10.1177/0022034513497961
- Marynka-Kalmani K, Treves S, Yafee M, Rachima H, Gafni Y, Cohen MA, Pitaru S. The lamina propria of adult human oral mucosa harbors a novel stem cell population. Stem Cells 2010; 28(5):984-95; PMID:20474080
- Treves-Manusevitz S, Hoz L, Rachima H, Montoya G, Tzur E, Vardimon A, Narayanan AS, Amar S, Arzate H, Pitaru S. Stem cells of the lamina propria of human oral mucosa and gingiva develop into mineralized tissues in vivo. J Clin Periodontol 2013; 40(1):73-81; PMID:23137193; http://dx.doi.org/10.1111/jcpe.12016
- Tomar GB, Srivastava RK, Gupta N, Barhanpurkar AP, Pote ST, Jhaveri HM, Mishra GC, Wani MR. Human gingiva-derived mesenchymal stem cells are superior to bone marrow-derived mesenchymal stem cells for cell therapy in regenerative medicine. Biochem Biophys Res Commun 2010; 393(3):377-83; PMID:20138833; http://dx.doi.org/10.1016/j.bbrc.2010.01.126
- Huang GT, Gronthos S, Shi S. Mesenchymal stem cells derived from dental tissues vs. those from other sources: their biology and role in regenerative medicine. J Dent Res 2009; 88(9):792-06; PMID:19767575; http://dx.doi.org/10.1177/0022034509340867
- Scheid RC, Woelfel JB. Woelfel's dental anatomy: Its relevance to dentistry. Philadelphia (PA): Lippincott Williams Wilkins; 2007.
- Andrés V, Walsh K. Myogenin expression, cell cycle withdrawal, and phenotypic differentiation are temporally separable events that precede cell fusion upon myogenesis. J Cell Biol 1996; 132(4):657-66; PMID:8647896; http://dx.doi.org/10.1083/jcb.132.4.657
- Lipton BH, Konigsberg IR. A fine-structural analysis of the fusion of myogenic cells. J Cell Biol 1972; 53(2):348-64; PMID:4554365; http://dx.doi.org/10.1083/jcb.53.2.348
- Konigsberg IR. Diffusion-mediated control of myoblast fusion. Dev Biol 1971; 26(1):133-52; PMID:4329509; http://dx.doi.org/10.1016/0012-1606(71)90113-8
- Buckley PA, Konigsberg IR. Myogenic fusion and the duration of the post-mitotic gap (G1). Dev Biol 1974; 37(1):193-212; PMID:4823501; http://dx.doi.org/10.1016/0012-1606(74)90179-1
- Zorin V, Zorina A, Cherkasov V, Deev R, Kopnin P, Isaev A. Clinical-instrumental and morphological evaluation of the effect of autologous dermal fibroblasts administration. J Tissue Eng Regen Med 2014; [ Epub ahead of print]; PMID:25524377; http://dx.doi.org/10.1002/term.1976
- Petrof G, Martinez-Queipo M, Mellerio JE, Kemp P, McGrath JA. Fibroblast cell therapy enhances initial healing in recessive dystrophic epidermolysis bullosa wounds: results of a randomized, vehicle-controlled trial. Br J Dermatol 2013; 169(5):1025-33; PMID:24032424; http://dx.doi.org/10.1111/bjd.12599
- Dominici M, Le Blanc K, Mueller I, Slaper-Cortenbach I, Marini F, Krause D, Deans R, Keating A, Prockop Dj, Horwitz E. Minimal criteria for defining multipotent mesenchymal stromal cells. The International Society for Cellular Therapy position statement. Cytotherapy 2006; 8(4):315-31.
- Tomar GB, Srivastava RK, Gupta N, Barhanpurkar AP, Pote ST, Jhaveri HM, Mishra GC, Wani MR. Human gingiva-derived mesenchymal stem cells are superior to bone marrow-derived mesenchymal stem cells for cell therapy in regenerative medicine. Biochem Biophys Res Commun 2010; 393(3):377-83; PMID:20138833; http://dx.doi.org/10.1016/j.bbrc.2010.01.126
- Yang H, Gao LN, An Y, Hu CH, Jin F, Zhou J, Jin Y, Chen FM. Comparison of mesenchymal stem cells derived from gingival tissue and periodontal ligament in different incubation conditions. Biomaterials 2013; 34(29):7033-47; PMID:23768902; http://dx.doi.org/10.1016/j.biomaterials.2013.05.025
- Zorin VL, Zorina AI, Eremin II, Bozo IY, Solovieva EV, Hromova NV, Kopnin PB. Comparative analysis of osteogenic potentials of multipotent mesenchymal stromal cells isolated from oral mucosa and bone marrow. Gens Cells 2014; 9(1):50-7. Russian
- Dezawa M, Ishikawa H, Itokazu Y, Yoshihara T, Hoshino M, Takeda S, Ide C, Nabeshima Y. Bone marrow stromal cells generate muscle cells and repair muscle degeneration. Science 2005; 309(5732):314-7; PMID:16002622; http://dx.doi.org/10.1126/science.1110364
- Shaffer LG, McGowan-Jordan J, Schmid M. An International System for Human Cytogenetic Nomenclature (2013). Recommendations of the International Standing Committee on Human Cytogenetic Nomenclature. Basel (Switzerland): S. Karger; 2013.