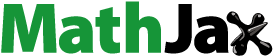
Abstract
Tiotropium and olodaterol are mainstay treatments for chronic obstructive pulmonary disease (COPD) and yield important clinical improvements, especially when used in fixed-dose combination. Whilst previous studies have shown consistent delivery of tiotropium to the lungs with the Respimat® inhaler, no such study has been carried out for olodaterol or the components of their fixed-dose combination (TIO/OLO). Combining in vitro and in silico models, we measured the amount of drug retained in the mouth–throat area, entering the trachea and reaching the lung periphery. We applied a hybrid deposition model that considered the experimentally determined output of an Alberta throat model (in vitro – dose to lung) combined with a computational fluid dynamic model of the lungs (in silico). Regardless of the COPD breathing pattern, ≥50% of the nominal dose of either tiotropium, olodaterol, or TIO and OLO in the fixed-dose combination reached the lung. Of the dose reaching the lungs, greater than 50% is deposited in the lung periphery (from generation 8 onwards). Our study demonstrated that aerosol delivery via the Respimat inhaler achieved high deposition deep into the lung periphery with all formulations evaluated.
Introduction
Chronic obstructive pulmonary disease (COPD) affects more than 250 million people globally and is expected to be the third-leading cause of death worldwide by 2030 [Citation1,Citation2]. The disease is characterized by chronic and often progressive respiratory symptoms, such as breathlessness, cough, increased sputum production and reduced exercise tolerance. Whilst preventable and treatable, COPD cannot be cured.
Small airways disease (SAD) is a key feature of COPD, in which the site of airflow obstruction resides in the peripheral airways (those with a diameter of less than 2 mm) that make up the bronchioles, terminal bronchioles, respiratory bronchioles, alveolar ducts and alveolar sacs [Citation3,Citation4]. In the respiratory tree, small airways typically reside from generation (G) 8 onwards [Citation3]. Patients with SAD and COPD typically have more severe airflow obstruction and poorer health status compared with those without small airway dysfunction [Citation5]. SAD can cause gas trapping on expiration and is the main cause of hyperinflation [Citation4]. The prevalence of SAD increases with COPD progression, making this area of the lung an important target for therapy [Citation6]. Since most inhaled therapies do not sufficiently reach the small airways [Citation7], there is a need for delivery devices designed to provide aerosol formulations that can do so.
Inhaled therapies such as the long-acting muscarinic antagonist (LAMA) tiotropium and the long-acting β2-agonist (LABA) olodaterol are a cornerstone of treatment for COPD, and yield improvements in lung function, dyspnea, exercise tolerance and quality of life, in particular when used in a fixed-dose combination [Citation8–11].
Marketed formulations of tiotropium, olodaterol and tiotropium/olodaterol fixed-dose combination (TIO/OLO) are available through delivery by the Respimat® Soft Mist™ inhaler, which uses spring power to produce a slow-moving liquid aerosol that produces a high fraction of fine particles [Citation12]. Fine particle fraction (FPF [<5 µm]) is important, as regional lung deposition varies according to the aerosol particle size and flow rate; there is debate around the cutoff value but the landmark of 5 µm is also adopted by pharmacopeial texts. Particles that are smaller than 5 µm have the greatest potential for deposition in the lungs [Citation13].
Aerosol velocity and spray duration also influence lung deposition, and the lower velocity and longer duration of the aerosol cloud generated by the Respimat device have been shown to result in higher lung deposition and lower oropharyngeal deposition compared with other inhalers [Citation12].
Mouth–throat models, such as the idealized ‘Alberta throat’ model mimicking the aerodynamic behavior of the mouth–throat region of adult subjects [Citation14], have been used for many years for lung deposition studies. The geometry of the model represents that of an average adult and is based on data from the literature and measurements using computed tomography scans and direct observation. The model possesses all the basic anatomical features of the real extrathoracic airway such as the oral cavity, oropharynx, larynx and trachea [Citation14]. In order to predict the fate of aerosol particles within lungs, single-path models or even whole-lung simulations have been carried out [Citation15–17]. Due to limited computer power and the complexity of the human lung, some approximations and simplifications are generally considered. Simulation models can be further refined by using functional respiratory imaging technology that uses computed tomography scans during full inhalation and full exhalation to show how different regions of the lung are functioning [Citation18]. Recently, in the framework of the European COST initiative, a multicenter project named SIMINHALE has investigated the fundamentals of these simulation models, underlining the need for, and the relevance of, predictions of drug delivery to the lungs [Citation19].
A number of in vivo and in vitro studies have investigated lung deposition by Respimat for various drugs, including tiotropium, and demonstrated deep lung deposition for drug aerosols with very similar characteristics to those produced by the device [Citation20–22]. However, the deposition of olodaterol in the lungs, either as monotherapy or in combination with tiotropium, has not yet been investigated by a dedicated study, but is implied from successful pharmacodynamic outcomes in clinical studies [Citation8,Citation23]. In this study, we aimed to evaluate deposition of olodaterol (monotherapy or as part of the fixed-dose combination of TIO/OLO) in the lungs with Respimat. As in vivo radiolabeled deposition studies using Respimat have already demonstrated deep lung deposition for drug aerosols [Citation20,Citation21,Citation24], there are ethical implications in repeating them, especially when no benefits are expected for the recipients of the radiolabeled products. Therefore, in this study, we repeated the in vitro/in silico work in which we first employed an Alberta throat model to measure the amount of drug retained in the oropharyngeal area and the amount entering the trachea (ultimately reaching the lungs), which is affected by the particle size distribution and the inhalation flow simulated. We then used an idealized single-path computational fluid dynamics (CFD) adult lung model to simulate the particle deposition by impaction and gravitational particle settling in the different regions of the lung from the trachea to G23.
Methods
This study consisted of two steps (): in the first step, an in vitro model was used to mimic aerosol flow in the mouth–throat region, with data obtained at the exit of the modeled trachea, simulating the amount of dose reaching the lung. In the second step, the dose-to-lung data were used in an in silico model, representing idealized lung geometry, to simulate particle deposition deep into the lungs.
Figure 1. Schematic of two-step study design. The idealized single-path model is shown in an extended view without considering the gravity angle.

In vitro study
A lung simulator (ASL 5000 Active Servo Lung, IngMar Medical, Pittsburgh, PA, USA) was programmed with breathing patterns of patients with moderate (50% ≤ forced expiratory volume in 1 s [FEV1] < 80% of predicted) or very severe (FEV1 < 30% of predicted) COPD (according to Global Initiative for Chronic Obstructive Lung Disease FEV1 cutoffs for disease classification) that had been generated in a previous study [Citation25]. For each formulation (tiotropium, olodaterol and the fixed-dose combination of TIO/OLO), three Respimat devices (Spiriva®, Striverdi® and Spiolto®) were tested to determine the amount of dose deposited in the lung, expressed as percent value of the nominal dose (ND). The average ND of tiotropium was 2.5 µg/actuation (Spiriva), of olodaterol was 2.5 µg/actuation (Striverdi), and of TIO/OLO combination was 2.5 µg + 2.5 µg/actuation (Spiolto), equaling the average delivered dose per actuation according to recent pharmacopeial definitions [Citation26]. Two actuations, one immediately after the other, form the equivalent of one ‘therapeutic dose’, which patients with COPD can take once daily. In order to obtain a good signal-to-noise ratio, 10 doses were accumulated during analytical work. The measurements were carried out in triplicate.
For the breathing pattern of very severe COPD [Citation25], the experimental set-up included an Alberta throat model, which is an aluminum cast mimicking the internal anatomy of an adult throat [Citation14]. This was attached to a mixing inlet connected to the lung simulator and placed on top of a Next Generation Impactor (NGI; Copley Scientific Ltd, Nottingham, UK) (). At the exit of the NGI, two pumps were connected that generated a constant airflow of 70 L/min. Details of the set-up have been described previously [Citation22]. The mass median aerodynamic diameter (MMAD) and the FPF (<5 μm) of the ND were measured using the NGI.
Figure 2. Experimental set-up for measuring deposition in the mouth–throat model (Alberta throat). NGI: Next Generation Impactor; RH: relative humidity.

To mimic the wet mucosal surface of the throat, a thin layer of Brij-glycerol emulsion (Brij 35®, polyoxyethylenmonolaurylester, Serva, Electrophoresis GmbH, Heidelberg, Germany) was used to coat the inside of the throat model and the NGI plates (with aerosols of aqueous solutions, such a coating is not essential in the same manner as for testing dry powder aerosols, where it is required to eliminate particle bounce-back). For the breathing pattern of moderate COPD [Citation25], a simplified experimental set-up was used, in which a filter was connected to the throat model instead of the NGI (the NGI was not used, as the moderate COPD inspiratory profile peak flow would exceed the flow calibration of the NGI). This set-up did not require a mixing inlet.
Outputs from the NGI cups and filter, the Respimat mouthpiece and the throat model were quantitatively determined by validated high-performance liquid chromatography [Citation22].
In silico studies
For the CFD assessments, an individual path model with realistic lung geometry (up to G23) was constructed based on lung pathology, as described by Finlay and employed in the work of Ciciliani et al. [Citation22,Citation27]. The individual path model was generated by a CAD program (SolidWorks, Dassault Systèmes, Vélizy-Villacoublay Cedex, France) and the geometry was then transferred to the computational fluid dynamic program (Fluent 15, back-option 14.5, ANSYS Inc., Canonsburg, PA, USA).
In our model, the airway of G8 has a diameter of 2.86 mm and, therefore, is above the defined limit of 2.0 mm that discriminates small airways [Citation7]; however, for consistency with published work that also used G8/B8 as the cutoff [Citation15], the two regions from trachea to G7 and G8 to alveoli were modeled. Boundary conditions (fictive air outflow starting at G15 and increasing to G23) were defined in order to account for the alveolar volume. The boundary condition at the trachea is velocity-in. At the first bifurcation, an asymmetric branching was enforced, considering the left–right bronchi asymmetry. The lower airway branchings were symmetrical. All open-ended branches have the condition velocity-out, and the outflow according to the pre-defined branching scheme was enforced. In addition, starting at G15, the flow into the alveoli was taken into account by setting the walls to velocity-out with small contributions to the outflow in order to reach a defined lung volume of 4 L. With respect to particles, all walls have the condition “trap”. Detailed lists of the fate of the particles were generated. Further improvements to the previous model were incorporated by tilting the individual segments between branching generations in order to obtain the average gravity angle calculated by Yeh and Schum [Citation28]. Apart from the first branching ratio (42% left and 58% right), the airflow was assumed to be equally distributed at the individual branches. The branching ratio in this model is higher compared with the model reported in the study by Longest et al. [Citation15]; however, this higher asymmetry results in good convergence of the simulation. The deposition mechanisms explicitly programmed were impaction and sedimentation. The mesh consisted of computational cells that were scaled from large size (at the trachea) to very small dimensions (at G23) in order to limit the computational effort. The total number of mesh cells was 8.4 million. The mesh size was smoothly adapted to the reduction of the airway caliber when traveling along the generations. Step effects were avoided by adding a short pre-run tube at the inlet and tubes added to the outlets after the branchings. The mesh was refined in order to provide sufficiently different pathways for particle tracks that are required during the subsequent Lagrangian particle simulation. The individual path was then extrapolated to the whole lung. An equal branching ratio was assumed (see above) and the statistical weight was obtained by the number of tube sections present in the specific generation.
Mean flow rates of 27.5, 55 and 82.5 L/min were used for CFD simulations. The flow rates were derived from a numerical fit of observed flow profiles of COPD patients [Citation25]. The value of 55 L/min was derived from averaging the first 1.5 s of the flow profile characterizing COPD patients inhaling in a way that was interpreted as a “slow and deep” breath. As the inhalation flow consists of a slow start before reaching a peak and falling off, an average of 55 L/min ± 50% (i.e. 22.5, 55 and 82.5 L/min) was selected to generate a range of air flows within the lung geometry. A turbulent k-omega-SST model was used for the simulation. The same geometry, mesh and flow rates were applied to the different drug products during the deposition calculation.
Particle size distributions mimicking the in vitro distribution patterns at the outlet of the Alberta throat model were applied to the in silico model. The particle range studied was simulated with test droplets having diameters from 0.2 to 9.5 μm, representing the experimentally determined size distributions (please see additional information in the Supplement for more information on size bins). The initial number of particles at the inlet of the trachea was 200,000 for individual size bins. Spherical droplets were generated in the program, mimicking the particle size distributions measured at the outlet of the Alberta throat. The standard Lagrangian particle tracking was applied. The droplets were modeled to flow out of the trachea of the Alberta throat into the upper part of the in silico CFD lung model. If a wall collision was detected in the model, then deposition in the upper airways was concluded. Any droplets passing through the model indicated deposition deeper in the lung. The percent lung deposition for eight particle size fractions was calculated using the formula:
This calculation of particle numbers of the same size and mass was then converted into a mass-weighted distribution. The weighing factor was determined from the experimental mass-weighted particle size distribution obtained by cascade impaction, allowing calculation of the overall deposition for each individual drug.
Results
In vitro study
shows the in vitro dose to the lung that was collected at the outlet of the Alberta throat model. For both moderate and very severe COPD inhalation patterns, ≥50% of the ND reached the lung. The highest percentage of ND reaching the lung was measured for the OLO component of the fixed-dose TIO/OLO combination under the very severe COPD breathing pattern (72% of ND), followed by the TIO component of the TIO/OLO fixed-dose combination (69% of ND), tiotropium (64% of ND) and olodaterol (58% of ND). For moderate COPD, the difference between the drug formulations was less pronounced, with results in the region of 50%. The differences in lung deposition were only significant (p < 0.05) when comparing moderate and very severe COPD inhalation patterns for the OLO component in the TIO/OLO combination (Supplementary Table 1).
Figure 3. Dose to lung collected at the outlet of the Alberta throat model. Error bars are standard deviation. COPD: chronic obstructive pulmonary disease; ND: nominal dose; OLO: olodaterol in the fixed-dose combination; TIO: tiotropium in the fixed-dose combination; TIO/OLO: tiotropium/olodaterol fixed-dose combination.

shows the cumulative particle size distributions of the lung fraction (the proportion of particles delivered to the NGI) for each of the formulations. The distribution indicates an FPF (<5 µm) at the outlet of the Alberta throat of 60–70% of the lung fraction and an MMAD in the range of 3–4 µm for all formulations (tiotropium: 3.30 µm; olodaterol: 3.72 µm; and 3.42 µm for the combination of TIO/OLO [based on analysis of TIO as only one droplet diameter in a homogenous solution]).
Figure 4. Cumulative particle size distribution, measured by NGI at the outlet of the Alberta throat model (very severe COPD). COPD: chronic obstructive pulmonary disease; FPF: fine particle fraction; MMAD: mass median aerodynamic diameter; NGI: Next Generation Impactor; OLO: olodaterol in the fixed-dose combination; TIO: tiotropium in the fixed-dose combination; TIO/OLO: tiotropium/olodaterol fixed-dose combination.

The FPF of the lung dose is shown in . Over 40% of the ND measured <5 µm for tiotropium and for the fixed-dose combination consisting of TIO/OLO; for olodaterol, this was 34%.
Figure 5. Fine particle fraction (<5 µm) in percent of the nominal dose measured at the outlet of the Alberta throat model. Error bars are standard deviations. COPD: chronic obstructive pulmonary disease; FPF: fine particle fraction; ND: nominal dose; OLO: olodaterol in the fixed-dose combination; TIO: tiotropium in the fixed-dose combination; TIO/OLO: tiotropium/olodaterol fixed-dose combination.

In silico studies
The deposition pattern in the 23 generations of the idealized three-dimensional single-path in silico lung model was investigated for all three formulations. Regional particle deposition was simulated using CFD () under three different flow rates (27.5, 55 and 82.5 L/min). At the flow rate of 27.5 L/min, between 10–13% and 87–90% of dose to lung was deposited in the trachea–G7 and G8–G23 regions for all formulations, respectively. Deposition for all formulations in the trachea–G7 region increased with increasing flow rates (25.0–30.3% for 55 L/min and 38.8–45.5% for 82.5 L/min); in the G8–G23 region, deposition decreased with increasing flow rates (69.7–75.0% for 55 L/min and 54.1–60.9% for 82.5 L/min). At each flow rate, percentage deposition was similar for all the formulations except olodaterol, which was slightly higher in the trachea–G7 region and slightly lower in the G8–G23 region compared with tiotropium and the fixed-dose combination components of TIO/OLO.
Figure 6. Regional deposition of particles in an adult lung model using a three-dimensional single-path simulation under flow rates of a) 27.5 L/min, b) 55 L/min and c) 82.5 L/min (reference: dose to lung, very severe COPD). G0–G23 represent generations of the lung branchings. With the exception of the first branching, the model assumes an equal flow separation into two daughter branches. The error bars have been calculated based on error propagation of experimental data (input variability from the particle size distributions of ). COPD: chronic obstructive pulmonary disease; DTL: dose to lung; OLO: olodaterol in the fixed-dose combination; TIO: tiotropium in the fixed-dose combination; TIO/OLO: tiotropium/olodaterol fixed-dose combination.

Discussion
This study has shown that both tiotropium and olodaterol, either alone or combined in a fixed-dose formulation, are delivered deep into the lungs via the Respimat device. Consistently high lung deposition of tiotropium and other drug formulations by Respimat has previously been shown [Citation20–22]. This study is the first to evaluate the deposition of olodaterol by Respimat either as monotherapy or as part of the fixed-dose combination of TIO/OLO using a combination of in vitro and in silico techniques.
Inhaled bronchodilator therapy is the cornerstone for the management of COPD, with dual bronchodilation recommended by the American Thoracic Society as the preferred treatment for patients experiencing dyspnea or exercise intolerance [Citation29]. Bronchodilators act by binding to the muscarinic acetylcholine receptors (mAChRs) (for LAMAs) and β2-adrenoceptors (for LABAs). It has been shown that the M3 type muscarinic receptors are found predominantly in the bronchus, with the density decreasing from the segmental to subsegmental bronchus, and is absent in lung parenchyma. The M1 subtype occurred in the lung only, and the M2 subtype was distributed ubiquitously in the bronchus and lungs. Adrenergic receptors are present along the airway, with increasing densities in the lung periphery. Muscarinic contractions and adrenoceptor relaxations in both bronchial regions are mediated through M3-mAChRs and β2-adrenoceptors, respectively, with effects on the periphery more driven by adrenergic agonism [Citation30]. The Respimat device produces a slow-moving aerosol with long spray duration and high FPF, which translates into high drug deposition into the lungs and periphery, as shown previously using scintigraphy () [Citation12,Citation20]. This uniformity can increase the precision of drug deposition near the receptors, and thus may increase the efficiency and effectiveness of inhaled drugs [Citation7].
Figure 7. Typical scintigraphic images for Respimat® SMI™, Turbohaler® DPI at slow inhaled flow rate, Turbohaler® DPI with fast inhaled flow rate, and Becloforte® pMDI. Reprinted with permission from Pitcairn et al. [Citation20]. Copyright © 2005. The publisher for this copyrighted material is MaryAnn Liebert, Inc. publishers. DPI: dry powder inhaler; pMDI: pressurized metered-dose inhaler; SMI: Soft MistTM Inhaler.
![Figure 7. Typical scintigraphic images for Respimat® SMI™, Turbohaler® DPI at slow inhaled flow rate, Turbohaler® DPI with fast inhaled flow rate, and Becloforte® pMDI. Reprinted with permission from Pitcairn et al. [Citation20]. Copyright © 2005. The publisher for this copyrighted material is MaryAnn Liebert, Inc. publishers. DPI: dry powder inhaler; pMDI: pressurized metered-dose inhaler; SMI: Soft MistTM Inhaler.](/cms/asset/d54ef4d6-3cf7-4cf0-9e87-07e1590fce8e/icop_a_1853091_f0007_c.jpg)
Accumulating evidence has indicated that some LAMAs may modulate airway contractility and airway hyper-responsiveness not only by blocking mAChRs expressed on the airway smooth muscle, but also via anti-inflammatory mechanisms such as blocking mAChRs expressed on inflammatory cells, submucosal glands and epithelial cells [Citation31], and reducing expression of inflammatory mediators [Citation32]. Moreover, non-neuronal acetylcholine released from airway epithelium modulates airway smooth muscle contractility in small airways and potentially also inflammation, making delivery to the small airways equally important.
Our finding that both tiotropium and olodaterol, either alone or combined in a fixed-dose formulation, are delivered deep into the lungs via Respimat is an important observation for the large proportion of COPD patients who also suffer from SAD. Small airways are structurally and physiologically very different from large airways [Citation3]. This portion of the airways, whilst therapeutically an important target, is difficult to reach sufficiently for most inhaled therapies. There is a significant correlation between SAD, dyspnea and quality of life [Citation33]. Effective targeting of the small airways in COPD may reduce the rate of emphysema progression [Citation34]. Our in vitro/in silico findings could therefore have direct relevance when translated into the clinical arena.
In this study, the Alberta throat model, which is a physiologically idealized model with demonstrated capability of mimicking aerosols and flow motion in the human mouth–throat, was used [Citation35–37]. The Alberta throat model was selected because of the documented development history of the throat and its geometric similarity to the dimensions of a human mouth [Citation14], making it suitable for analytical work in the laboratory. It replaces the United States Pharmacopeia sample inlet, which consists of a rectangular bend. The realistic Alberta throat model coupled with simulated inspiration profiles is considered superior to current compendial methods [Citation38]. Historically, the Andersen cascade impactor was used in environmental sciences; as the size range of measurable particles overlapped with the range of pharmaceutical aerosols, the pharmaceutical community used this instrument for the determination of aerodynamic particle size distributions. However, its stacked design requires strict leak checks, plus there is the risk of inadvertent exchange of stages resulting in invalid measurements. Therefore, based on experiences with this impactor and other impactor types, an industry consortium designed the Next Generation (Pharmaceutical) Impactor, NGI [Citation39]. The NGI mechanical design is robust: the stages cannot be mixed, and calibrations are available for 15 L/min and for the range from 30 L/min to 100 L/min.
Results from this mouth–throat model in vitro set-up indicate that the proportion of drug entering the simulated lung is similar for the fixed-dose combination of TIO/OLO and tiotropium monotherapy, with slightly lower values for olodaterol monotherapy. The difference found with olodaterol when comparing monotherapy with OLO in the fixed-dose combination is likely due to differences of the formulations: whilst hydrochloric acid is part of the aqueous solution in the fixed-dose combination and tiotropium monotherapy, the aqueous solution for olodaterol monotherapy contains citric acid; the different acids result in different electrical conductivities of the solutions for inhalation, which in turn give rise to different electrostatics, resulting in slightly different deposition characteristics.
As previously observed, the dose to the lung was lower using the moderate COPD breathing pattern, compared with a severe COPD breathing pattern [Citation22]. This is due to moderate COPD patients inhaling more strongly than patients who suffer from very severe disease, thereby generating increased airflow velocity. This results in particles not being able to follow the airflow through the throat and impacting in the mouth–throat region instead. However, for both very severe and moderate COPD breathing patterns, the modeled dose to the lung was ≥50% of the nominal dose for all active drugs investigated.
Particle size is a major determinant in how an inhaled drug is deposited and distributed within the lungs, and is thus related to local efficacy [Citation40]. An MMAD of less than 5 µm is required to allow the drug to reach the lower respiratory tract, past the carina, and into the small airways [Citation41]. However, the ideal particle size for inhaled pharmaceuticals is still subject to discussion, and deposition in the airways is also dependent on other variable factors, such as flow rate, co-ordination of the inhalation and airway geometry. The cumulative particle size distribution for the in vitro dose to the lung was very similar for all formulations with an MMAD of 3–4 µm and an FPF at the outlet of the Alberta throat of 60–70%. This high FPF is in line with, and builds on, what we have demonstrated previously in a comparison of four inhalers, which showed that Respimat (tiotropium) had the highest percentage of FPF [Citation22].
The FPFs assessed in this study are higher using the idealized Alberta throat model [Citation42] rather than the pharmacopeial FPF definitions, the latter based on the right-angle bend European Pharmacopeia/United States Pharmacopeia (Ph.EUR./USP) throat and constant flow rate breathing patterns [Citation26,Citation43]. Previous studies have shown that the Ph.EUR./USP throat removes fewer large particles from aerosols than is likely to occur in reality [Citation44,Citation45]. This implies that large particles are filtered out more efficiently by the Alberta throat model, resulting in a shift in the MMAD to smaller values and increased FPF (<5 µm) in the impactor when using the more realistic model.
A previous study (using the pharmacopeial set-up) investigating the aerodynamic particle size distribution of TIO/OLO using the Andersen cascade impactor found that there were a considerable number of fine particles present in the formulation [Citation46]. Generally, it is believed that small particles (0.1–1 µm) are mainly exhaled; however, it has been argued that their capability to be distributed throughout the whole lungs and to reach distal airways may lead to a higher pulmonary deposition [Citation41].
CFD deposition calculations were used to simulate regional deposition of particles in the adult lung. Our findings show that a large portion of the aqueous aerosols generated by Respimat penetrate deep into the lung and subsequently deposit in the periphery. The highest deposition of particles in the periphery using the single-path simulation was observed with the OLO and TIO components of the fixed-dose combination and with tiotropium monotherapy (as shown previously) [Citation22], with a slightly lower peripheral deposition with olodaterol monotherapy (consistent with the slightly lower proportion of dose delivered to the lungs for this formulation). In the periphery, particle deposition for all formulations was highest at lower flow rates and decreased consistently as the flow rate increased. This can be attributed to the fact that faster inspiratory flow rates can increase inertial impaction of aerosols in the oropharynx and at bifurcations in the large central airways, and this reduces the proportion of particles deposited in the peripheral airways [Citation47].
Good lung deposition with the Respimat device has been demonstrated with several formulations: an in vivo scintigraphic study found that the deposition of corticosteroids from the Respimat device was more efficient compared with a dry powder inhaler or a pressurized metered-dose inhaler (pMDI) [Citation20]. Similar findings were also reported by Brand et al., who found that in vivo delivery of radiolabeled fenoterol hydrobromide 50 μg/ipratropium bromide 20 μg to the lungs with Respimat was more efficient than with a pMDI, even among patients with poor inhaler technique [Citation24].
Although this work is a result of in vitro experimentation and CFD modeling, where possible the work was adjusted to be as realistic as possible by factoring in elements such as humidity, a fluid film applied to the throat model, accounting for gravity, and using a lung path model based on geometry gained from pathology. However, there are limitations. The Alberta throat model, although more realistic than a right-angle bend, is a simplification of reality. It has been characterized to capture the typical deposition properties of the human oropharynx [Citation48]. Regarding the CFD model, as with all mathematical models, the results will be dependent upon a number of assumptions. In this analysis, calculations were refined over those previously published [Citation22]; we assumed asymmetric airflow at the non-equal first bifurcation (reflecting lung physiology more closely) [Citation49]. As in previously studied aqueous models, we did not account for particle shrinking as the formulations are highly dilute and shrinkage would not be anticipated in vivo in lungs with relative humidity approaching 100%.
In addition, although this study was conducted with the disposable Respimat device, results are expected to be equivalent with the re-usable Respimat device, as the drug formulations as well as the metering and aerosolization systems are identical in the two devices [Citation46].
In conclusion, we have shown that aerosol delivery via the Respimat inhaler achieves high particle deposition deep into the lung periphery with all formulations evaluated. Targeting the small airways is an important treatment goal in COPD, and our results show that tiotropium and olodaterol as well as the fixed-dose combination TIO/OLO reach this area of the lung, which may help to alleviate progressive pathological abnormalities associated with the disease.
Supplemental Material
Download PDF (709.4 KB)Supplemental Material
Download PDF (92.4 KB)Acknowledgments
We thank Prof. Warren Finlay for a prototype of his idealized throat model (now available at Copley Scientific Limited, Nottingham, UK). Dr. Ralf Kröger (ANSYS Germany) was consulted regarding the CFD calculations.
Data availability statement
The data used and analyzed during the current study are available from the corresponding author on reasonable request.
Declaration of interest
AMC’s research for her PhD thesis was funded by Boehringer Ingelheim. HW is an employee of Boehringer Ingelheim and has patent WO2004024340A1-Blockiervorrichtung für ein Sperrspannwerk. PL has received consulting fees from Boehringer Ingelheim. MD completed an internship with Boehringer Ingelheim at the time of this work. TV has received consulting and presentation fees from Boehringer Ingelheim.
Additional information
Funding
References
- World Health Organization. Burden of COPD [Internet]. 2019. [cited 2019 Dec 12]. Available from: http://www.who.int/respiratory/copd/burden/en/.
- World Health Organization. Chronic obstructive pulmonary disease (COPD) [Internet]. 2017. [cited 2019 Dec 20]. Available from: http://www.who.int/mediacentre/factsheets/fs315/en/.
- McNulty W, Usmani OS. Techniques of assessing small airways dysfunction. Eur Clin Respir J. 2014;1:1. DOI:10.3402/ecrj.v1.25898
- Singh D. Small airway disease in patients with chronic obstructive pulmonary disease. Tuberc Respir Dis (Seoul). 2017;80(4):317–324. DOI:10.4046/trd.2017.0080
- Pisi R, Aiello M, Zanini A, et al. Small airway dysfunction and flow and volume bronchodilator responsiveness in patients with chronic obstructive pulmonary disease. Int J Chron Obstruct Pulmon Dis. 2015;10:1191–1197. DOI:10.2147/COPD.S82509
- Crisafulli E, Pisi R, Aiello M, et al. Prevalence of small-airway dysfunction among COPD patients with different GOLD stages and its role in the impact of disease. Respiration. 2017;93(1):32–41. DOI:10.1159/000452479
- Lavorini F, Pedersen S, Usmani OS, et al. Dilemmas, confusion, and misconceptions related to small airways directed therapy. Chest. 2017;151(6):1345–1355. DOI:10.1016/j.chest.2016.07.035
- Buhl R, Maltais F, Abrahams R, et al. Tiotropium and olodaterol fixed-dose combination versus mono-components in COPD (GOLD 2-4). Eur Respir J. 2015;45(4):969–979. DOI:10.1183/09031936.00136014
- Singh D, Ferguson GT, Bolitschek J, et al. Tiotropium + olodaterol shows clinically meaningful improvements in quality of life. Respir Med. 2015;109(10):1312–1319. DOI:10.1016/j.rmed.2015.08.002
- O'Donnell DE, Casaburi R, Frith P, et al. Effects of combined tiotropium/olodaterol on inspiratory capacity and exercise endurance in COPD. Eur Respir J. 2017;49(4):1601348. DOI:10.1183/13993003.01348-2016
- Troosters T, Bourbeau J, Maltais F, et al. Enhancing exercise tolerance and physical activity in COPD with combined pharmacological and non-pharmacological interventions: PHYSACTO randomised, placebo-controlled study design. BMJ Open. 2016;6(4):e010106 DOI:10.1136/bmjopen-2015-010106
- Anderson P. Use of Respimat Soft Mist inhaler in COPD patients. Int J Chron Obstruct Pulmon Dis. 2006;1(3):251–259. DOI:10.2147/copd.2006.1.3.251
- Laube BL, Janssens HM, de Jongh FH, International Society for Aerosols in Medicine, et al. What the pulmonary specialist should know about the new inhalation therapies. Eur Respir J. 2011;37(6):1308–1331. DOI:10.1183/09031936.00166410
- Johnstone A, Uddin M, Pollard A, et al. The flow inside an idealised form of the human extra-thoracic airway. Exp Fluids. 2004;37(5):673–689. DOI:10.1007/s00348-004-0857-4
- Longest PW, Tian G, Walenga RL, et al. Comparing MDI and DPI aerosol deposition using in vitro experiments and a new stochastic individual path (SIP) model of the conducting airways. Pharm Res. 2012;29(6):1670–1688. DOI:10.1007/s11095-012-0691-y
- Kolanjiyil AV, Kleinstreuer C. Computational analysis of aerosol-dynamics in a human whole-lung airway model. J Aerosol Sci. 2017;114:301–316. DOI:10.1016/j.jaerosci.2017.10.001
- Kolanjiyil AV, Kleinstreuer C, Sadikot RT. Computationally efficient analysis of particle transport and deposition in a human whole-lung-airway model. Part II: dry powder inhaler application. Comput Biol Med. 2017;84:247–253. DOI:10.1016/j.compbiomed.2016.10.025
- Hajian B, De Backer J, Vos W, et al. Functional respiratory imaging (FRI) for optimizing therapy development and patient care. Expert Rev Respir Med. 2016;10(2):193–206. DOI:10.1586/17476348.2016.1136216
- COST: European Cooperation in Science & Technology. MP1404 - Simulation and pharmaceutical technologies for advanced patient-tailored inhaled medicines (SimInhale): Final Assessment Review [Internet]. [cited 2020 Jun 4]. Available from: https://e-services.cost.eu/files/domain_files/MPNS/Action_MP1404/final_achievement_report/final_achievement_report-MP1404.pdf.
- Pitcairn G, Reader S, Pavia D, et al. Deposition of corticosteroid aerosol in the humanlung by Respimat® Soft Mist™ inhaler compared to deposition by metered dose inhaler or by Turbuhaler® dry powder inhaler. J Aerosol Med. 2005;18(3):264–272. DOI:10.1089/jam.2005.18.264
- Newman SP, Brown J, Steed KP, et al. Lung deposition of fenoterol and flunisolide delivered using a novel device for inhaled medicines: comparison of RESPIMAT with conventional metered-dose inhalers with and without spacer devices. Chest. 1998;113(4):957–963. DOI:10.1378/chest.113.4.957
- Ciciliani AM, Langguth P, Wachtel H. In vitro dose comparison of Respimat® inhaler with dry powder inhalers for COPD maintenance therapy. Int J Chron Obstruct Pulmon Dis. 2017;12:1565–1577. DOI:10.2147/COPD.S115886
- Koch A, Pizzichini E, Hamilton A, et al. Lung function efficacy and symptomatic benefit of olodaterol once daily delivered via Respimat® versus placebo and formoterol twice daily in patients with GOLD 2–4 COPD: results from two replicate 48-week studies. Int J Chron Obstruct Pulmon Dis. 2014;9:697–714. DOI:10.2147/COPD.S62502. eCollection 2014.
- Brand P, Hederer B, Austen G, et al. Higher lung deposition with Respimat Soft Mist inhaler than HFA-MDI in COPD patients with poor technique. Int J Chron Obstruct Pulmon Dis. 2008;3(4):763–770.
- Wachtel H, Flüge T, Gössl R. Flow-pressure-energy-power: which is the essential factor in breathing patterns of patients using inhalers? Respir Drug Deliv. 2006;2:511–514.
- European Directorate for the Quality of Medicines Healthcare. European Pharmacopoeia (Ph. Eur.). 10th ed. Strasbourg, France: EDQM Council of Europe; 2020. (Council of Europe, editor.).
- Finlay WH. The mechanics of inhaled pharmaceutical aerosols: an introduction. London: Academic Press; 2001.
- Yeh HC, Schum GM. Models of human lung airways and their application to inhaled particle deposition. Bull Math Biol. 1980;42(3):461–480. DOI:10.1007/BF02460796
- Nici L, Mammen MJ, Charbek E, et al. Pharmacologic management of COPD: an official American Thoracic Society clinical practice guideline. Am J Respir Crit Care Med. 2020;201(9):e56–e69. DOI:10.1164/rccm.202003-0625ST
- Ikeda T, Anisuzzaman A, Yoshiki H, et al. Regional quantification of muscarinic acetylcholine receptors and β-adrenoceptors in human airways. Br J Pharmacol. 2012;166(6):1804–1814. DOI:10.1111/j.1476-5381.2012.01881.x
- Alagha K, Palot A, Sofalvi T, et al. Long-acting muscarinic receptor antagonists for the treatment of chronic airway diseases. Ther Adv Chronic Dis. 2014;5(2):85–98. DOI:10.1177/2040622313518227
- Kang K, Kim HH, Choi Y. Tiotropium is predicted to be a promising drug for COVID-19 through transcriptome-based comprehensive molecular pathway analysis. Viruses. 2020;12(7):776. DOI:10.3390/v12070776
- Haruna A, Oga T, Muro S, et al. Relationship between peripheral airway function and patient-reported outcomes in COPD: a cross-sectional study. BMC Pulm Med. 2010;10:10 DOI:10.1186/1471-2466-10-10
- Higham A, Quinn AM, Cancado JED, et al. The pathology of small airways disease in COPD: historical aspects and future directions. Respir Res. 2019;20(1):49 DOI:10.1186/s12931-019-1017-y
- DeHaan WH, Finlay WH. In vitro monodisperse aerosol deposition in a mouth and throat with six different inhalation devices. J Aerosol Med. 2001;14(3):361–367. DOI:10.1089/089426801316970321
- Stapleton K-W, Guentsch E, Hoskinson M, et al. On the suitability of k–ε turbulence modeling for aerosol deposition in the mouth and throat: a comparison with experiment. J Aerosol Sci. 2000;31(6):739–749. DOI:10.1016/S0021-8502(99)00547-9
- Finlay WH, Martin AR. Recent advances in predictive understanding of respiratory tract deposition. J Aerosol Med Pulm Drug Deliv. 2008;21(2):189–206. DOI:10.1089/jamp.2007.0645
- Wei X, Huynh BK, Kaviratna A, et al. Comparing aerosol doses and aerodynamic particle size distributions from a dry powder inhaler, pressurized metered dose inhaler, and soft mist inhaler using realistic and compendial in vitro testing. Respir Drug Deliv. 2018;2:675–680.
- Marple VA, Olson BA, Santhanakrishnan K, et al. Next generation pharmaceutical impactor: a new impactor for pharmaceutical inhaler testing. Part III. Extension of archival calibration to 15 L/min. J Aerosol Med. 2004;17(4):335–343. DOI:10.1089/jam.2004.17.335
- Darquenne C. Aerosol deposition in health and disease. J Aerosol Med Pulm Drug Deliv. 2012;25(3):140–147. DOI:10.1089/jamp.2011.0916
- Jabbal S, Poli G, Lipworth B. Does size really matter?: Relationship of particle size to lung deposition and exhaled fraction. J Allergy Clin Immunol. 2017;139(6):2013–2014.e1. DOI:10.1016/j.jaci.2016.11.036
- Zhang Y, Gilbertson K, Finlay WH. In vivo-in vitro comparison of deposition in three mouth-throat models with Qvar® and Turbuhaler® inhalers. J Aerosol Med. 2007;20(3):227–235. DOI:10.1089/jam.2007.0584
- The United States Pharmacopeial Convention. <601> Inhalation and nasal drug products—aerosols, sprays, and powders—performance quality tests [Internet]. 2015. [cited 2019 Dec 31]. Available from: https://www.uspnf.com/print/pdf/node/62616.
- Cheng YS, Zhou Y, Su WC. Deposition of particles in human mouth–throat replicas and a USP induction port. J Aerosol Med Pulm Drug Deliv. 2015;28(3):147–155. DOI:10.1089/jamp.2013.1105
- Mitchell J, Copley M, Sizer Y, et al. Adapting the Abbreviated Impactor Measurement (AIM) concept to make appropriate inhaler aerosol measurements to compare with clinical data: a scoping study with the “Alberta” Idealized Throat (AIT) inlet. J Aerosol Med Pulm Drug Deliv. 2012;25(4):188–197. DOI:10.1089/jamp.2011.0925
- Dhand R, Eicher J, Hansel M, et al. Improving usability and maintaining performance: human-factor and aerosol-performance studies evaluating the new reusable Respimat inhaler. Int J Chron Obstruct Pulmon Dis. 2019;14:509–523. DOI:10.2147/COPD.S190639
- Capstick TG, Clifton IJ. Inhaler technique and training in people with chronic obstructive pulmonary disease and asthma. Expert Rev Respir Med. 2012;6(1):91–101. DOI:10.1586/ers.11.89
- Grgic B, Finlay WH, Heenan AF. Regional aerosol deposition and flow measurements in an idealized mouth and throat. J Aerosol Sci. 2004;35(1):21–32. DOI:10.1016/S0021-8502(03)00387-2
- Longest PW, Tian G, Khajeh-Hosseini-Dalasm N, et al. Validating whole-airway CFD predictions of DPI aerosol deposition at multiple flow rates. J Aerosol Med Pulm Drug Deliv. 2016;29(6):461–481. DOI:10.1089/jamp.2015.1281