Abstract
c-Src can disrupt the connexin43 (Cx43) and zonula occludens-1 (ZO-1) interaction, leading to down-regulation of gap junction intercellular communication. Previously, the authors characterized the interaction of domains from these proteins with the carboxyl terminus of Cx43 (Cx43CT) and found that binding of the c-Src SH3 domain to Cx43CT disrupted the Cx43CT/ZO-1 PDZ-2 domain complex. Because Cx43 and Cx40 form heteromeric connexons and display similar mechanisms of pH regulation, the authors addressed whether Cx40CT interacts with these domains in a similar manner as Cx43CT. Nuclear magnetic resonance (NMR) data indicate that Cx40CT is an intrinsically disordered protein. NMR titrations determined that PDZ-2 affected the last 28 Cx40CT residues and SH3 shifted numerous amino-terminal Cx40CT residues. Finally, the Cx40CT/PDZ-2 complex was unaffected by SH3 and both domains interacted simultaneously with Cx40CT. This result differs from when the same experiment was performed with Cx43CT, suggesting different mechanisms of regulation exist between connexin isoforms, even when involving the same molecular partners.
Gap junctions are integral membrane proteins that enable the direct cytoplasmic exchange of ions and low-molecular-weight metabolites between adjacent cells. They provide a pathway for the propagation and/or amplification of signal transduction cascades triggered by cytokines, growth factors, and other cell signaling molecules involved in growth regulation and development. Gap junctions are formed by the apposition of connexons from adjacent cells, where each connexon is formed by six connexin proteins. Connexins are tetraspan transmembrane domain proteins with intracellular amino and carboxyl termini. There are 21 different connexin genes in the human genome. The major divergence in primary structures, which allow specific regulatory properties for each isoform, occurs in the carboxyl terminal (CT) domain. Each of the connexins can form gap junctions by themselves; however, studies have demonstrated that many cells coexpress more than one connexin isoform, giving rise to heteromeric connexons (i.e., more than one connexin isotype in the same single connexon; for review see Koval Citation2006).
Connexin43 (Cx43) and connexin40 (Cx40) coexpress in several tissues, including cardiac atrial and ventricular myocytes, and vascular smooth muscle (He et al. Citation1999). The intercellular communication provided by Cx43 and Cx40 gap junctions (GJIC) is a principal determinant of myocardial conduction, and altered expression has been implicated in arrhythmogenesis (van Veen et al. Citation2006). Cx43 and Cx40 can hetero-oligomerize, leading to the formation of heterologous gap junctions. The physiological consequences of hetero-oligomerization on GJIC remain unclear, though functional studies demonstrate that these structures display biophysical properties that do not correspond to their homomeric forms (Burt et al. Citation2001; Cottrell and Burt Citation2001; Cottrell et al. Citation2002; Gu et al. Citation2000b; Valiunas et al. Citation2000, Citation2001). Because the sensitivity of different homomeric gap junctions to biochemical modulators varies widely, the question arises as to what are the types of interactions that occur between connexins to integrate regulatory signals in heteromeric channels. Evidence supports involvement of the CT domains in regulation of these heteromeric channels.
Previous studies have shown that pH regulation, as well as the existence of the lower-conductance state of Cx43 and of Cx40, depend on the presence of the CT domain (Gu et al. Citation2000a, Citation2000b; Stergiopoulos et al. Citation1999). Moreover, replacing the Cx40CT with the CT domain of Cx43 rescues the behavior of the homologous channel, both when the fragments (i.e., the Cx40 truncated channel and the Cx43CT domain) are separately coexpressed and when both domains are covalently attached (Anumonwo et al. Citation2001; Stergiopoulos et al. Citation1999). Additionally, coexpression of Cx43 and Cx40 in the same cell enhances the susceptibility of the gap junction channel to acidification-induced uncoupling (Gu et al. Citation2000a, Citation2000b). These data support the notion that both Cx43 and Cx40 (1) interact within a heteromeric connexon in a synergistic manner; (2) have regulatory domains (e.g., the CT domain) that can specifically interact with a channel formed by another connexin; and (3) follow the “particle-receptor” hypothesis for pH gating. In this regard, studies from our laboratory have characterized the pH-dependent structural changes of the Cx43CT domain (Hirst-Jensen et al. Citation2007; Sorgen et al. Citation2004b), as well as the structural changes that occur upon binding known molecular partners involved in pH regulation (Sorgen et al. Citation2004a). However, from the standpoint of the Cx40CT, little information is available regarding molecular partner interactions and currently no structure is available.
Here, we provide evidence that the Cx40CT is intrinsically disordered in structure and report the Cx40CT structural changes that occur upon binding to the SH3 domain of c-Src and the second PDZ domain (PDZ-2) of zonula occludens (ZO)-1. Our experiments identify selected groups of Cx40CT residues whose position in space is altered by the presence of the ligate. The regions of structural reorganization include but span beyond those expected from consensus SH3 and PDZ binding domains. Although the overall Cx40CT structural changes mediated by the SH3 and PDZ-2 domains resemble that of the Cx43CT domain, potentially significant differences in the mechanism of ZO-1 dissociation from these CT domains are discussed.
METHODS
Expression and Purification of Recombinant Proteins
Cx40CT (residues 251 to 355 of Cx40 and four additional amino acids appended to the amino terminus; see Duffy et al. 2002), 15N-Cx40CT, the SH3 domain of c-Src, and the PDZ-2 domain of ZO-1 were expressed and purified as previously described (Sorgen et al. Citation2004a). All proteins were confirmed for purity and analyzed for degradation by sodium dodecyl sulfate–polyacrylamide gel electrophoresis (SDS-PAGE) and mass spectroscopy.
Nuclear Magnetic Resonance
Nuclear magnetic resonance (NMR) data were acquired at 7°C using a 600-MHz Varian INOVA NMR spectrometer outfitted with either a cryoprobe or pentaprobe. Experimental data to determine backbone sequential assignments have been described (Bouvier Citation2007). Connectivities were derived from nuclear Overhauser effects (NOEs) observed in 15N-NOESY-HSQC and 13C-NOESY-HSQC spectra, with mixing times of 125 and 150 ms, respectively. NMR spectra were processed using NMRPipe (Delaglio et al. Citation1995) and analyzed using NMRView (Johnson Citation1994).
Gradient-enhanced two-dimensional 1H15N-HSQC experiments (Kay et al. Citation1992) were used to observe all backbone amide resonances in 15N-labeled Cx40CT. Data were acquired with 1024 complex points in the direct dimension and 256 complex points in the indirect dimension. Sweep widths were 10,000 Hz in the proton dimension and 2500 Hz in the nitrogen dimension. The buffer conditions for each protein (i.e., Cx40CT, c-Src SH3 domain, and ZO-1 PDZ-2 domain) were equilibrated in 1× phosphate-buffered saline (PBS) at pH 6.0 and 1 mM dithiothreitol (DTT), using NAP-5 columns (GE Healthcare). Binding isotherms from 1H15N-HSQC titration experiments were calculated with ORIGIN 7.0 software (Microcal Software, Northampton, MA).
RESULTS
Structure Prediction and Description of the Cx40CT
Our laboratory recently published the resonance assignments for the Cx40CT (Bouvier Citation2007). We have now combined the information provided from the α-and β-carbon chemical shift values and NOE spectroscopy to predict the residues that may be present in stable secondary structures (). A major factor affecting α-and β-carbon chemical shift values is the backbone geometry, which can cause differences of greater than ± 2 ppm from random coiled values for residues in α-helical and β-sheet conformations (Iwadate et al. Citation1999). Analysis of the Cx40CT found little to no chemical shift deviations from random coil for the α-and β-carbons (). Additionally, the 15N-NOESY-HSQC and 13C-NOESY-HSQC spectra contained little to no medium-or long-range NOE connectivities (i.e., i,i+2, i,i+3, or greater), which are commonly found in well-folded structures (). These observations, combined with the low chemical shift dispersion found in the 1H15N-HSQC (Bouvier Citation2007), are consistent with the prediction of the Cx40CT as an intrinsically disordered protein.
Figure 1 Secondary structural analysis of the Cx40CT domain. (A) Analysis of the chemical shift deviations from random coil for α-and β-carbon chemical shift. All residues located immediately N-terminal to a proline residue, except Q320, had α-carbon chemical shift values shifted approximately −2 ppm. (B) Backbone NOEs used in the structural analysis.
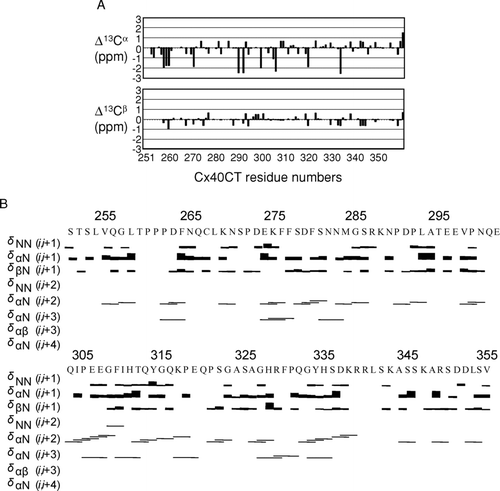
Structural Changes in the Cx40CT Resulting from Binding of Cellular Partners
We have previously characterized the structural modifications brought about by the interaction of Cx43CT with two well-known molecular partners, c-Src and ZO-1 (Sorgen et al. Citation2004a). Because Cx43 and Cx40 are coexpressed throughout the cardiovascular system, form heteromeric connexons, and display similar mechanisms of pH regulation, we addressed whether Cx40 has the ability to interact with domains from these proteins in a similar manner as Cx43. The identification of the Cx40CT assignments allowed for an assessment of the modifications that result from its interaction with the SH3 domain of c-Src and the PDZ-2 domain of ZO-1 (Bouvier Citation2007). Unlabeled SH3 or PDZ-2 fragments were diluted in a 1× PBS buffer solution (pH 6.0) containing 15N-Cx40CT, and 1H15N-HSQC spectra were acquired. The 1H15N-HSQC is a two-dimensional (2D) NMR experiment where each amino acid (except proline) gives one signal (or chemical shift) that corresponds to its N-H amide group. These chemical shifts are sensitive to the chemical environment and even small changes in structure and/or dynamics can change the chemical shift of an amino acid.
The addition of the PDZ-2 domain of ZO-1 affected the resonance peaks of the last 28 amino acids of the Cx40CT, a region larger than what was expected from a putative “PDZ binding domain” (Songyang et al. Citation1997). Indeed, as seen in , the signal from residues G333 to V355 completely disappeared (i.e., broadened beyond detection), whereas the amplitude of the peaks corresponding to residues H328 to Q332 decreased in intensity. These changes can be explained by decreased tumbling rates caused by an increase in molecular weight and/or intermediate chemical exchange rates, both indicative of a structural modification caused by the association of the two molecules.
Figure 2 1H15N-HSQCs of the Cx40 carboxyl terminus (Cx40CT) in the presence of the PDZ-2 domain of ZO-1. This experiment allowed for detection of the regions of Cx40CT that were structurally modified when in the presence of a binding domain from the molecular partner ZO-1. (A) Cx40CT was titrated with the PDZ-2 domain of ZO-1 to a 1:2 molar ratio. The control spectra, Cx40CT only (black), have been overlapped with spectra obtained when both molecules were present at a 1:2 molar ratio (red). The amino acid peaks that were affected have been labeled. (B) Close-up view from A (dotted box) to illustrate a sample of the affected amino acids. (C) Summary of the Cx40CT residues affected by the presence of the ZO-1 PDZ-2 domain. Residues that disappeared (green) and decreased in intensity (grey) have been labeled.
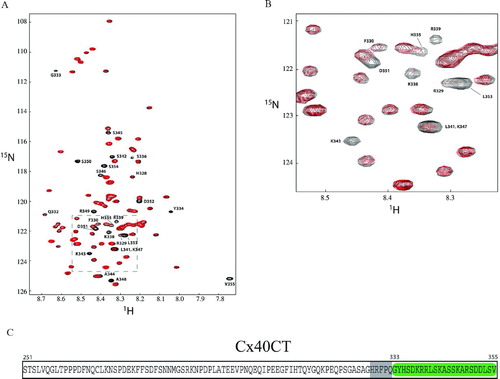
To calculate the dissociation constant (KD) for the Cx40CT/ZO-1 PDZ-2 domain interaction, 1H15N-HSQC data were obtained upon titration of Cx40CT with different concentrations of PDZ-2 (). The decreasing signal intensity for a subset of the Cx40CT residues affected as a result of the increasing PDZ-2 concentrations (; residues R349, S350, S354, and V355) were fit according to the nonlinear least squares method and the titration data afforded a KD of 27.6 ± 2.2 μ M (mean ± SD; ).
Figure 3 Change in chemical shift intensities on Cx40CT binding to the ZO-1 PDZ-2 domain. (A) 1H15N-HSQC titration of 15N-Cx40CT with unlabeled PDZ-2 in which the Cx40CT:PDZ-2 concentration ratios ranged from 1:0 to 1:5. Each titration contained the same concentration of 15N-Cx40CT (20 μ M) with different concentrations of the PDZ-2 domain. The cross-peak color changes according to the concentration ratio (Cx40CT:PDZ-2 1:0 [black], 1:0.5 [red], 1:1 [green], 1:2 [blue], 1:2.5 [magenta], 1:3.5 [black], 1:5 [cyan]). The arrow points in the direction of the increasing PDZ-2 concentrations. The overlapped spectra have been offset for easier visualization of the decrease in signal intensity. A294 is the control demonstrating a Cx40CT residue that had no change in signal intensity. (B) The dissociation constant (KD) for the Cx40CT/PDZ-2 interaction was estimated by fitting the observed decreases in signal intensity for Cx40CT residues R349, S350, S354, and V355 as a function of PDZ-2 concentration.
![Figure 3 Change in chemical shift intensities on Cx40CT binding to the ZO-1 PDZ-2 domain. (A) 1H15N-HSQC titration of 15N-Cx40CT with unlabeled PDZ-2 in which the Cx40CT:PDZ-2 concentration ratios ranged from 1:0 to 1:5. Each titration contained the same concentration of 15N-Cx40CT (20 μ M) with different concentrations of the PDZ-2 domain. The cross-peak color changes according to the concentration ratio (Cx40CT:PDZ-2 1:0 [black], 1:0.5 [red], 1:1 [green], 1:2 [blue], 1:2.5 [magenta], 1:3.5 [black], 1:5 [cyan]). The arrow points in the direction of the increasing PDZ-2 concentrations. The overlapped spectra have been offset for easier visualization of the decrease in signal intensity. A294 is the control demonstrating a Cx40CT residue that had no change in signal intensity. (B) The dissociation constant (KD) for the Cx40CT/PDZ-2 interaction was estimated by fitting the observed decreases in signal intensity for Cx40CT residues R349, S350, S354, and V355 as a function of PDZ-2 concentration.](/cms/asset/87ad9fc3-486c-493c-b498-98f0c08cdcb0/icac_a_301600_uf0003_b.jpg)
Addition of the SH3 domain strongly shifted (≥ 0.035 ppm) the resonance peaks of amino acids V255 to N265, F276 to S278, S281, G285 to A294, and I310 to H311 and weakly shifted (< 0.035 ppm) the resonance peaks of amino acids L254, Q266-L268, N270, D279 to F280, G308, G315, K317, E319, S325, G327 to H328, F330, Q332 to H335, and R339 (). Interestingly, amino acids H328, F330, Q332 to H335, and R339 overlap with the residues involved in binding the ZO-1 PDZ-2 domain to Cx40CT. The KD for the Cx40CT/c-Src SH3 domain interaction was also calculated by 1H15N-HSQC data obtained upon titration of Cx40CT with different concentrations of SH3 (). For the SH3 domain, the change in chemical shifts for a subset of the Cx40CT residues affected as a result of the increasing SH3 concentrations (; residues Q256, G257, R287, and N289) were fit according to the nonlinear least squares method and the titration data afforded a KD of 1.1 ± 0.2 mM (mean ± SD; ).
Figure 4 1H15N-HSQCs of the Cx40 carboxyl terminus (Cx40CT) in the presence of the SH3 domain of c-Src. This experiment allowed for detection of the regions of Cx40CT that were structurally modified when in the presence of a binding domain from the molecular partner c-Src. (A) Cx40CT was titrated with the SH3 domain of c-Src to a 1:4 molar ratio. The control spectra, Cx40CT only (black), have been overlapped with spectra obtained when both molecules were present at a 1:4 molar ratio (red). The amino acid peaks that were affected have been labeled. (B) Close-up view from panel A (dotted box) to illustrate a sample of the affected amino acids. (C) Summary of the Cx40CT residues affected by the presence of the c-Src SH3 domain. Residues that shifted ≥ 0.035 ppm (red) and < 0.035 ppm (grey) have been labeled.
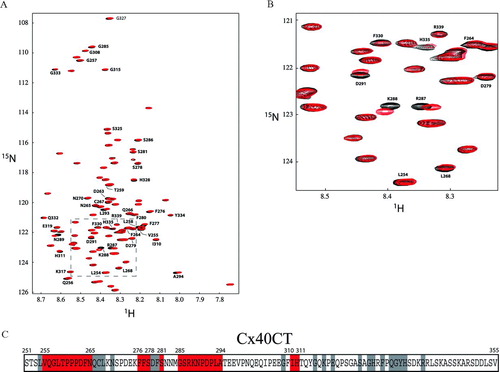
Figure 5 Change in chemical shift position on Cx40CT binding to the c-Src SH3 domain. (A) 1H15N-HSQC titration of 15N-Cx40CT with unlabeled SH3 in which Cx40CT:SH3 concentration ratios ranged from 1:0 to 1:32. Each titration contained the same concentration of 15N-Cx40CT (100 μ M) with different concentrations of the c-Src SH3 domain. The cross-peak color changes according to the concentration ratio (Cx40CT:SH3 1:0 [black], 1:1 [green], 1:7 [blue], 1:13 [red], 1:32 [black]). The arrow points in the direction of the increasing SH3 concentrations. Titrations of 1:0.6, 1:4, 1:10, 1:16, and 1:24 were also collected and used for calculating the dissociation constant (B), but were not included for easier visualization of the chemical shift changes. N300 is the control demonstrating a Cx40CT residue that did not shift. (B) The dissociation constant (KD) for the Cx40CT/SH3 interaction was estimated by fitting the change in chemical shift (Δ δ) for Cx40CT residues G257, Q256, R287, and N289 as a function of SH3 concentration. The Δ δ was calculated according to the formula Δ δav = √((Δ δHN)2+ (Δ δ N/5)2).
![Figure 5 Change in chemical shift position on Cx40CT binding to the c-Src SH3 domain. (A) 1H15N-HSQC titration of 15N-Cx40CT with unlabeled SH3 in which Cx40CT:SH3 concentration ratios ranged from 1:0 to 1:32. Each titration contained the same concentration of 15N-Cx40CT (100 μ M) with different concentrations of the c-Src SH3 domain. The cross-peak color changes according to the concentration ratio (Cx40CT:SH3 1:0 [black], 1:1 [green], 1:7 [blue], 1:13 [red], 1:32 [black]). The arrow points in the direction of the increasing SH3 concentrations. Titrations of 1:0.6, 1:4, 1:10, 1:16, and 1:24 were also collected and used for calculating the dissociation constant (B), but were not included for easier visualization of the chemical shift changes. N300 is the control demonstrating a Cx40CT residue that did not shift. (B) The dissociation constant (KD) for the Cx40CT/SH3 interaction was estimated by fitting the change in chemical shift (Δ δ) for Cx40CT residues G257, Q256, R287, and N289 as a function of SH3 concentration. The Δ δ was calculated according to the formula Δ δav = √((Δ δHN)2+ (Δ δ N/5)2).](/cms/asset/02678936-97d5-416d-911f-cc3fde8b04b3/icac_a_301600_uf0005_b.jpg)
Cx40CT Simultaneously Associates with Multiple Cellular Partners
c-Src can disrupt the Cx43/ZO-1 interaction, leading to down-regulation of gap junction intercellular communication (Duffy et al. Citation2004; Toyofuku et al. Citation2001). Our previous NMR studies demonstrated that binding of the c-Src SH3 domain to the Cx43CT disrupted the association of the Cx43CT/ZO-1 PDZ-2 domain complex (Sorgen et al. Citation2004a). Here, we determined if a similar mechanism exists in which SH3-induced structural modifications in Cx40CT affected the conformation of the region ascribed as the PDZ-2 binding domain (residues H328 to V355; see ). 15N-Cx40CT (, black) was combined with unlabeled PDZ-2 in PBS buffer (pH 6.0) to a final 1:2 molar concentration (, green); unlabeled SH3 was titrated into the complex (, blue: 1:2:2 molar concentration; red: 1:2:4 molar concentration) and 1H15N-HSQC spectra were acquired. Clearly, addition of SH3 had no effect on the Cx40CT residues, which were affected as a result of the Cx40CT/PDZ-2 interaction. It is worth noting that the presence of the PDZ-2 domain did not affect the shifts in the Cx40CT resonance peaks caused by the presence of the SH3 domain. Finally, a decrease in the intensity of the peaks was observed for Cx40CT residues R287 to A294, which only shifted in the presence of SH3 alone. This observation is consistent with either an increase of the molecular weight of Cx40CT due to the binding of both the c-Src SH3 and ZO-1 PDZ-2 domains or the exchange rate of this Cx40CT region bound to the SH3 domain changed from fast to intermediate on the NMR time scale (i.e., higher binding affinity). Altogether, these data suggest that both c-Src and ZO-1 interact simultaneously with the Cx40CT and that c-Src alone may not be responsible for displacement of the Cx40CT/ZO-1 interaction, a departure from the observations made with the Cx43CT.
Figure 6 1H15N-HSQCs demonstrating the Cx40CT interacts simultaneously with the ZO-1 PDZ-2 and c-Src SH3 domains. The Cx40CT residues that disappeared due to the Cx43CT/PDZ-2 interaction (G333-V355; green) and shifted ≥ 0.035 ppm due to the addition of the SH3 domain (V255-N265, F276-S278, S281, G285-A294, and I310-H311; black) have been labeled. Colors correspond to 1H15N-HSQC spectra of Cx40CT alone (black), Cx40CT combined with unlabeled PDZ-2 (green), 15N-Cx40CT/ZO-1 PDZ-2/c-Src SH3 (1:2:2 molar ratio; blue), and 15N-Cx40CT/ZO-1 PDZ-2/c-Src-SH3 (1:2:4 molar ratio; red). Each sample contained the same concentration of 15N-Cx40CT (100 μ M).
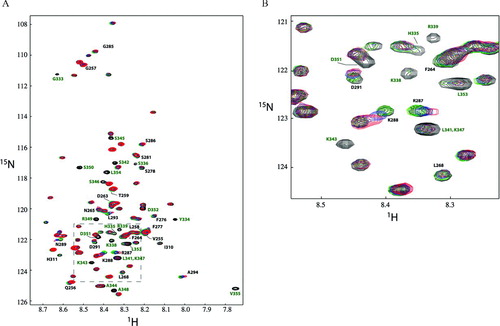
DISCUSSION
We have characterized the structure of the carboxyl terminal domain of Cx40 by the use of NMR. Our data identified the structural modifications brought about by the interaction of Cx40CT with two well-known molecular partners and determined that those modifications are rigid, so that binding to one partner does not affect the interaction with the other. These studies further expand our structural knowledge of how connexin domains integrate in multimolecular complexes and provide insight into the molecular mechanisms involved in the regulation of Cx40.
Comparison of the Cx40CT and Cx43CT Structures
The Cx43CT structure was determined to be predominately random coiled, but shows two short helical domains, which may be involved in protein interactions (Sorgen et al. Citation2002, Citation2004b). In this article, we show that the Cx40CT, on the other hand, is completely unstructured according to standard NMR predictors (i.e., α-and β-carbon chemical shift values and NOEs connectivities). Our previous work showed that the Cx43CT domain undergoes pH-dependent homo-oligomerization; we also demonstrated that residues within the two helical domains are involved in the dimerization interface (Hirst-Jensen et al. Citation2007; Sorgen et al. Citation2004b). In contrast, sedimentation equilibrium and chemical cross-linking studies of the Cx40CT indicated only a small increase in dimerization upon acidification of the solvent (unpublished data). Together, these data support the notion that helical domains play an important role in the process of pH-dependent homo-oligomerization, which may occur in some, but not all connexin CT domains.
An interesting question that arises is, what is the physiological importance of an intrinsically disordered Cx40CT or Cx43CT domain? In general, intrinsically disordered proteins have been identified as playing important roles in molecular recognition, molecular assembly, protein modifications, and entropic chains (Dunker et al. Citation2002). In the case of membrane proteins, like Cx40 and Cx43, intrinsically disordered domains seem to play an important role in cell signaling events, acting as components of macromolecular processes and substrates for regulatory events such as phosphorylation (Dunker and Obradovic Citation2001). We speculate that a disordered CT allows the connexin molecule to be a point of convergence for alternative regulatory pathways. Multiple alternative interactions of low affinity (even if of high specificity) allows a single molecule to rapidly transition from one molecular partner to another, thus acting as a “switchboard” for adapting the properties of cell communication to the environment prevalent in the intracellular space. Interestingly, the CT domains of Cx40 and Cx43 are not alone in their structural characteristics. Analysis of genomic sequence data strongly suggested that there is a high prevalence of intrinsically disordered domains. Indeed, an estimated 41% of human membrane proteins, including connexins, glutamate [NMDA] receptor subunit ε 4, and voltage-dependent T-type calcium channel α-1G subunit (Minezaki et al. Citation2007), have intrinsically disordered regions with more than 30 consecutive residues, and these residues are preferentially localized at the cytoplasmic side.
Comparison of the Cx40CT and Cx43CT Interaction with the ZO-1 PDZ-2 Domain
Many studies have focused on the relationship between Cx43 and ZO-1 (for review see Herve Citation2007). ZO-1 is important for maintaining cell polarity as well as for coupling the extracellular environment to intracellular signaling pathways and the cytoskeleton (Itoh et al. Citation1993). A Cx43/ZO-1 interaction has been identified in many cells (e.g., myocytes [Giepmans and Moolenaar 1998]); however, ZO-1 binding is not essential for the formation of functional Cx43 channels (Dunham et al. Citation1992; Fishman et al. Citation1991). Data suggest that this interaction may regulate the formation (Toyofuku et al. Citation1998) and size of the Cx43 gap junction plaque (Hunter et al. Citation2005), as well as the internalization and remodeling of Cx43 upon changes in the intracellular environment (Barker et al. Citation2002; Duffy et al. Citation2004).
We demonstrated that the PDZ-2 domain of ZO-1 affected residues H328-V355 of Cx40, a region larger than what was expected from a consensus “PDZ binding domain” (Doyle et al. Citation1996; Morais Cabral et al. Citation1996). The different dynamics between residues H328 to Q332 and G333 to V355 indicate a possible tighter association between residues G333 to V355, which contains the consensus-binding motif for ZO-1. Although this result is similar to what was observed when the PDZ-2 domain associated with the Cx43CT (Sorgen et al. Citation2004a), the Cx43CT and Cx40CT domains differed in the number of residues affected and in the dynamics of the interaction. Overall, the PDZ-2 domain affected the last 28 residues of the Cx40CT and 19 residues of the Cx43CT (S364 to I382). If a comparison is made between the more “tightly” associating residues, only the last 11 Cx43CT residues were affected, whereas the last 23 Cx40CT were affected. This suggests an overall tighter association of the PDZ-2 domain with Cx40CT than Cx43CT.
Comparison of the Cx40CT and Cx43CT Interaction with the c-Src SH3 Domain
Many studies have demonstrated an association of Cx43 with c-Src, a plasma membrane-associated tyrosine kinase (Solan and Lampe Citation2005). c-Src– (and v-Src)–induced phosphorylation of Cx43 has been correlated with an inhibition of gap junction intercellular communication (GJIC). Src activation may lead to tyrosine phosphorylation (Lin et al. Citation2001) as well as indirectly serine phosphorylation (perhaps MAPK-mediated; Zhou et al. 1999). The mechanism of channel closure has been proposed to occur through a “particle-receptor” type of interaction, similar to that proposed for pH gating of Cx43 channels (Cottrell et al. Citation2003; Homma et al. Citation1998; Morley et al. Citation1996; Zhou et al. Citation1999), which results in a decrease in electrical coupling caused by a reduced channel open probability and possibly altered selectivity (Cottrell et al. Citation2003).
Although many studies have determined that the SH3 domain of activated Src binds the CT domain of Cx43 near P280 (Calero et al. Citation1998; Kanemitsu et al. Citation1997; Sorgen et al. Citation2004a), to the best of our knowledge, no studies have demonstrated that c-Src can associate with Cx40. Our data indicate that SH3 binding induces major structural changes throughout the Cx40CT (V255 to N265, F276 to S278, S281, G285 to A294, and I310 to H311), unlike the Cx43CT, which was isolated to one specific region (K264 to K287). The Cx40CT sequence does not contain a consensus PXXP SH3 binding motif; however, a number of peptides lacking a PXXP motif, but rich in prolines and/or positively charged residues, have been identified (Jia et al. Citation2005). Two of the Cx40CT domains affected by SH3 correspond to this description: V255 to N265 contains three prolines and R287 to L293 contains one arginine, one lysine, and two prolines. Another difference between these CT domains upon binding the SH3 domain was that the Cx40CT residues shifted (exchange between the free and fully bound states of Cx40CT is fast on the chemical shift timescale), whereas Cx43CT residues K264 to 287 disappeared (exchange is intermediate on the chemical shift timescale), suggesting the SH3 domain has a tighter binding affinity for the Cx43CT. This observation is supported by the NMR data where the binding affinity for the SH3 interaction with Cx40CT (KD = 1.1 mM) was significantly lower than those values calculated by SPR for the Cx43CT (KD = 4.5 μ M; Duffy et al. Citation2004).
Comparison of c-Src–Mediated Signaling via Cx40CT and Cx43CT
The binding of Cx43 to c-Src and to ZO-1 are interdependent. c-Src can disrupt the Cx43/ZO-1 interaction, leading to down-regulation of GJIC (Duffy et al. Citation2004; Toyofuku et al. Citation2001). Interestingly, the primary binding sites for ZO-1 (PDZ-2 domain) and c-Src (SH3 domain) correspond to Cx43 regions that are distant in primary sequence (∼ 100 residues apart) (Sorgen et al. Citation2004a). Our NMR studies demonstrated that binding of the c-Src SH3 domain has global effects on the Cx43CT structure, extending upstream to the c-Src SH2 binding domain and downstream to the PDZ-2 binding domain of ZO-1; moreover, SH3 binding to the Cx43CT disrupted the association of the Cx43CT/ZO-1 PDZ-2 domain complex (Sorgen et al. Citation2004a). Surprisingly, in spite of the sequence differences between the Cx40CT and Cx43CT domains, the residues affected upon binding of the SH3 and PDZ-2 domains to Cx40CT are very similar to those observed for Cx43CT, suggesting a comparable signalling event mediated by c-Src (). However, the Cx40CT/ZO-1 PDZ-2 domain complex is not affected by the addition of the c-Src SH3 domain. We have observed that an interaction between the ZO-1 PDZ-2 and c-Src SH3 domains exists and that this interaction may be involved in displacement of the Cx43CT/PDZ-2 binding (unpublished data). Therefore, we speculate that, if compared to the Cx43CT/PDZ-2 association, a combination of an increased affinity of Cx40CT for PDZ-2, and the increased number of Cx40CT residues affected by the PDZ-2 interaction (competitive inhibition), hinders the ability of SH3 to displace PDZ-2 from its Cx40CT binding site. Future studies will address the physiological role of c-Src in the context of binding and regulating homomeric and heteromeric Cx40 gap junctions, as well as determining whether Cx40CT phosphorylation or other molecular partner interactions are necessary to dissociate the Cx40CT/ZO-1 interaction.
Figure 7 Comparison of the Cx40CT and Cx43CT residues affected by the interaction with the ZO-1 PDZ-2 and c-Src SH3 domains. Data for the Cx43CT interaction with the ZO-1 PDZ-2 and c-Src SH3 domains was obtained from (Sorgen et al. Citation2004a).
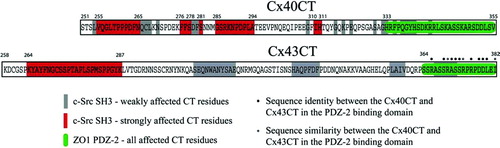
This work was supported by United States Public Health Service grant GM072631 and the Nebraska Research Initiative for the Nebraska Center for Structural Biology. The authors would like to thank Dr. Mario Delmar for his critical reading of the manuscript.
REFERENCES
- Anumonwo J M, Taffet S M, Gu H, Chanson M, Moreno A P, Delmar M. The carboxyl terminal domain regulates the unitary conductance and voltage dependence of connexin40 gap junction channels. Circ Res 2001; 88: 666–673
- Barker R J, Price R L, Gourdie R G. Increased association of ZO-1 with connexin43 during remodeling of cardiac gap junctions. Circ Res 2002; 90: 317–324
- Bouvier D, Kieken F, Sorgen P L. 1H, 13C, and 15N backbone resonance assignments of the carboxyl terminal domain of Connexin40. Biomol NMR Assign 2007; 1: 1–3
- Burt J M, Fletcher A M, Steele T D, Wu Y, Cottrell G T, Kurjiaka D T. Alteration of Cx43:Cx40 expression ratio in A7r5 cells. Am J Physiol Cell Physiol 2001; 280: C500–C508
- Calero G, Kanemitsu M, Taffet S M, Lau A F, Delmar M. A 17mer peptide interferes with acidification-induced uncoupling of connexin43. Circ Res 1998; 82: 929–935
- Cottrell G T, Burt J M. Heterotypic gap junction channel formation between heteromeric and homomeric Cx40 and Cx43 connexons. Am J Physiol Cell Physiol 2001; 281: C1559–C1567
- Cottrell G T, Lin R, Warn-Cramer B J, Lau A F, Burt J M. Mechanism of v-Src-and mitogen-activated protein kinase-induced reduction of gap junction communication. Am J Physiol Cell Physiol 2003; 284: C511–C520
- Cottrell G T, Wu Y, Burt J M. Cx40 and Cx43 expression ratio influences heteromeric/heterotypic gap junction channel properties. Am J Physiol Cell Physiol 2002; 282: C1469–C1482
- Delaglio F, Grzesiek S, Vuister G W, Zhu G, Pfeifer J, Bax A. NMRPipe: A multidimensional spectral processing system based on UNIX pipes. J Biomol NMR 1995; 6: 277–293
- Doyle D A, Lee A, Lewis J, Kim E, Sheng M, MacKinnon R. Crystal structures of a complexed and peptide-free membrane protein-binding domain: molecular basis of peptide recognition by PDZ. Cell 1996; 85: 1067–1076
- Duffy H S, Ashton A W, O'Donnell P, Coombs W, Taffet S M, Delmar M, Spray D C. Regulation of connexin43 protein complexes by intracellular acidification. Circ Res 2004; 94: 215–222
- Duffy H S, Sorgen P L, Girvin M E, O'Donnell P, Coombs W, Taffet S M, Delmar M, Spray D C. pH-dependent intramolecular binding and structure involving Cx43 cytoplasmic domains. J Biol Chem 2002; 277: 36706–36714
- Dunham B, Liu S, Taffet S, Trabka-Janik E, Delmar M, Petryshyn R, Zheng S, Perzova R, Vallano M L. Immunolocalization and expression of functional and nonfunctional cell-to-cell channels from wild-type and mutant rat heart connexin43 cDNA. Circ Res 1992; 70: 1233–1243
- Dunker A K, Brown C J, Lawson J D, Iakoucheva L M, Obradovic Z. Intrinsic disorder and protein function. Biochemistry 2002; 41: 6573–6582
- Dunker A K, Obradovic Z. The protein trinity—linking function and disorder. Nat Biotechnol 2001; 19: 805–806
- Fishman G I, Moreno A P, Spray D C, Leinwand L A. Functional analysis of human cardiac gap junction channel mutants. Proc Natl Acad Sci U S A 1991; 88: 3525–3529
- Giepmans B N, Moolenaar W H. The gap junction protein connexin43 interacts with the second PDZ domain of the zona occludens-1 protein. Curr Biol 1998; 8: 931–934
- Gu H, Ek-Vitorin J F, Taffet S M, Delmar M. Coexpression of connexins 40 and 43 enhances the pH sensitivity of gap junctions: A model for synergistic interactions among connexins. Circ Res 2000a; 86: E98–E103
- Gu H, Ek-Vitorin J F, Taffet S M, Delmar M. UltraRapid communication: Coexpression of connexins 40 and 43 enhances the pH sensitivityof gap junctions: A model for synergistic interactions among connexins. Circ Res 2000b; 86: 1100
- He D S, Jiang J X, Taffet S M, Burt J M. Formation of heteromeric gap junction channels by connexins 40 and 43 in vascular smooth muscle cells. Proc Natl Acad Sci U S A 1999; 96: 6495–6500
- Herve J, Bourmeyster N, Sarrouilhe D, Duffy H. Gap junctional complexes: From partners to functions. Prog Biophys Mol Biol 2007; 94: 29–65
- Hirst-Jensen B J, Sahoo P, Kieken F, Delmar M, Sorgen P L. Characterization of the pH-dependent interaction between the gap junction protein connexin43 carboxyl terminus and cytoplasmic loop domains. J Biol Chem 2007; 282: 5801–5813
- Homma N, Alvarado J L, Coombs W, Stergiopoulos K, Taffet S M, Lau A F, Delmar M. A particle-receptor model for the insulin-induced closure of connexin43 channels. Circ Res 1998; 83: 27–32
- Hunter A W, Barker R J, Zhu C, Gourdie R G. Zonula occludens-1 alters connexin43 gap junction size and organization by influencing channel accretion. Mol Biol Cell 2005; 16: 5686–5698
- Itoh M, Nagafuchi A, Yonemura S, Kitani-Yasuda T, Tsukita S. The 220-kD protein colocalizing with cadherins in non-epithelial cells is identical to ZO-1, a tight junction-associated protein in epithelial cells: cDNA cloning and immunoelectron microscopy. J Cell Biol 1993; 121: 491–502
- Iwadate M, Asakura T, Williamson M P. C alpha and C beta carbon-13 chemical shifts in proteins from an empirical database. J Biomol NMR 1999; 13: 199–211
- Jia C Y, Nie J, Wu C, Li C, Li S S. Novel Src homology 3 domain-binding motifs identified from proteomic screen of a Pro-rich region. Mol Cell Proteomics 2005; 4: 1155–1166
- Johnson BAa.B. R. A. NMRView: A computer program for the visualization and analysis of NMR data. J Biomol NMR 1994; 4: 603–614
- Kanemitsu M Y, Loo L W, Simon S, Lau A F, Eckhart W. Tyrosine phosphorylation of connexin 43 by v-Src is mediated by SH2 and SH3 domain interactions. J Biol Chem 1997; 272: 22824–22831
- Kay L E, Keifer P, Saarinen T. J Am Chem Soc 1992; 114: 10663
- Koval M. Pathways and control of connexin oligomerization. Trends Cell Biol 2006; 16: 159–166
- Lin R, Warn-Cramer B J, Kurata W E, Lau A F. v-Src-mediated phosphorylation of connexin43 on tyrosine disrupts gap junctional communication in mammalian cells. Cell Commun Adhes 2001; 8: 265–269
- Minezaki Y, Homma K, Nishikawa K. Intrinsically disordered regions of human plasma membrane proteins preferentially occur in the cytoplasmic segment. J Mol Biol 2007; 368: 902–913
- Morais Cabral J H, Petosa C, Sutcliffe M J, Raza S, Byron O, Poy F, Marfatia S M, Chishti A H, Liddington R C. Crystal structure of a PDZ domain. Nature 1996; 382: 649–652
- Morley G E, Taffet S M, Delmar M. Intramolecular interactions mediate pH regulation of connexin43 channels. Biophys J 1996; 70: 1294–1302
- Solan J L, Lampe P D. Connexin phosphorylation as a regulatory event linked to gap junction channel assembly. Biochim Biophys Acta 2005; 1711: 154–163
- Songyang Z, Fanning A S, Fu C, Xu J, Marfatia S M, Chishti A H, Crompton A, Chan A C, Anderson J M, Cantley L C. Recognition of unique carboxyl-terminal motifs by distinct PDZ domains. Science 1997; 275: 73–77
- Sorgen P L, Duffy H S, Cahill S M, Coombs W, Spray D C, Delmar M, Girvin M E. Sequence-specific resonance assignment of the carboxyl terminal domain of Connexin43. J Biomol NMR 2002; 23: 245–246
- Sorgen P L, Duffy H S, Sahoo P, Coombs W, Delmar M, Spray D C. Structural changes in the carboxyl terminus of the gap junction protein connexin43 indicates signaling between binding domains for c-Src and zonula occludens-1. J Biol Chem 2004a; 279: 54695–54701
- Sorgen P L, Duffy H S, Spray D C, Delmar M. pH-Dependent Dimerization of the Carboxyl Terminal Domain of Cx43. Biophys J 2004b; 87: 574–581
- Stergiopoulos K, Alvarado J L, Mastroianni M, Ek-Vitorin J F, Taffet S M, Delmar M. Hetero-domain interactions as a mechanism for the regulation of connexin channels. Circ Res 1999; 84: 1144–1155
- Toyofuku T, Akamatsu Y, Zhang H, Kuzuya T, Tada M, Hori M. c-Src regulates the interaction between connexin-43 and ZO-1 in cardiac myocytes. J Biol Chem 2001; 276: 1780–1788
- Toyofuku T, Yabuki M, Otsu K, Kuzuya T, Hori M, Tada M. Direct association of the gap junction protein connexin-43 with ZO-1 in cardiac myocytes. J Biol Chem 1998; 273: 12725–12731
- Valiunas V, Gemel J, Brink P R, Beyer E C. Gap junction channels formed by coexpressed connexin40 and connexin43. Am J Physiol Heart Circ Physiol 2001; 281: H1675–1689
- Valiunas V, Weingart R, Brink P R. Formation of heterotypic gap junction channels by connexins 40 and 43. Circ Res 2000; 86: E42–E49
- van Veen T A, van Rijen H V, Jongsma H J. Physiology of cardiovascular gap junctions. Adv Cardiol 2006; 42: 18–40
- Zhou L, Kasperek E M, Nicholson B J. Dissection of the molecular basis of pp60(v-src) induced gating of connexin 43 gap junction channels. J Cell Biol 1999; 144: 1033–1045