ABSTRACT
Maintenance of telomeres is essential for genome integrity and replicative capacity in eukaryotic cells. Telomerase, the ribonucleoprotein complex that catalyses telomere synthesis is minimally composed of a reverse transcriptase and an RNA component. The sequence and structural domains of human telomerase RNA (hTR) have been extensively characterized, while the regulation of hTR transcription, maturation, and localization, is not fully understood. Here, we provide an up-to-date review of hTR, with an emphasis on current breakthroughs uncovering the mechanisms of hTR maturation and localization.
Introduction
Telomeres are nucleoprotein structures at the ends of eukaryotic chromosomes that ensure the maintenance of genomic stability. Telomeric DNA shortens after each round of DNA replication due to the end replication problem [Citation1]. As a result, critically short telomeres trigger a DNA damage response and cause the cells to enter replicative senescence, which is one of the hallmarks of ageing[Citation2]. To maintain telomere length and replicative capacities, most eukaryotic organisms utilize the telomerase ribonucleoprotein complex to elongate telomeres by reverse transcription. Catalytically active human telomerase in cells is composed of the reverse transcriptase hTERT, the telomerase RNA hTR, telomerase Cajal Body protein 1 (TCAB1), dyskerin, NOP10, NHP2, and GAR1 [Citation3], although in vitro telomerase activity only requires hTERT and hTR [Citation4]. In humans, telomerase is present in germ cells, stem cells, and about 85% of cancer cells, while hTERT expression is inactivated in somatic cells to protect against tumorigenesis [Citation5,Citation6]. In addition, mutations in telomerase components lead to rapid telomere shortening and premature ageing diseases.
A review of human telomerase biogenesis has been recently published [Citation7]. In this article, we provide up-to-date information and perspectives on hTR biogenesis and functions, with an emphasis on the regulation of its transcription, maturation, and subcellular localization.
Structural domains of hTR
Telomerase RNA is highly variable in sequence and structure among species. The structural domains of the 451-nt hTR have been well studied and reviewed previously [Citation8,Citation9], and are shown in . The 5ʹ region of hTR possesses the template region, a pseudoknot secondary structure, and a template boundary element, together known as the core domain [Citation8,Citation9]. A structural and functional counterpart of the core domain exists in the telomerase RNA of other species.
Figure 1. The schematic diagram of hTR. The 5ʹ guanosine track forms a G-quadruplex structure at the early stage of hTR biogenesis, which competes with correct hTR folding and template boundary element (TBE) formation if not resolved properly. The core domain of hTR includes the pseudoknot secondary structure that interacts with hTERT, the template region, and TBE. The H/ACA domain comprises two hairpins, each is bound by a set of the H/ACA complex during hTR biogenesis. The apical region of the 5ʹ hairpin, termed conserved region (CR) 4/5, also interacts with hTERT. The apical loop of the 3ʹ hairpin contains the CAB box that interacts with TCAB1 and regulates hTR localization, and the BIO box that facilitates recruitment of the H/ACA complex to hTR
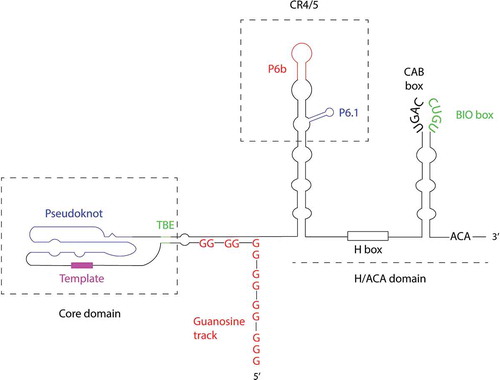
Unlike yeast telomerase RNA that resembles small nuclear RNA (snRNA) [Citation10–12], vertebrate telomerase RNA adopts the H/ACA domain at its 3ʹ end, which contains a 5ʹ hairpin followed by the H box comprising a consensus sequence (5ʹ-ANANNA-3ʹ where N is any nucleotide), a 3ʹ hairpin, and the ACA trinucleotide [Citation13]. The H/ACA box is also present in H/ACA box small nucleolar (sno) RNA and small Cajal Body (sca) RNA that guide the pseudouridylation of ribosomal RNA and snRNA, respectively [Citation14]. However, no pseudouridylation target of hTR has been reported. The P6b and P6.1 loops are positioned in the apical region of the 5ʹ hairpin known as the conserved region (CR) 4/5, which is absent in other H/ACA sno and scaRNAs [Citation15]. The apical loop of the 3ʹ hairpin contains the Cajal body box (CAB box, consensus UGAG) and biogenesis promoting box (BIO box, a unique feature in hTR) sequences, which are required for localization and accumulation of hTR, respectively [Citation16,Citation17].
hTR biogenesis and localization
Transcriptional regulation of hTR by promoter and downstream elements
While most of the human H/ACA box snoRNAs and scaRNAs are embedded in introns, hTR is transcribed individually under the control of its own promoter [Citation18]. Unlike ciliate telomerase RNAs which are transcribed by RNA Polymerase III (Pol III), yeast and vertebrate telomerase RNAs are transcribed by RNA Polymerase II (Pol II). Placing the hTR gene under the control of a Pol III promoter produces transcripts with accumulation defects [Citation19].
Pol II cooperates with the mediator or integrator complexes to effectuate transcription. Mediator is a multi-subunit scaffolding complex that connects transcription factors with Pol II and general transcription factors, and is required for the formation of the transcription pre-initiation complex [Citation20]. Unlike mediator that regulates transcription of most mRNAs, the integrator complex has been shown to terminate transcription of snRNA and replication-dependent histone mRNA [Citation21]. A recent study showed that the hTR promoter is involved in transcription termination by recruiting the integrator complex. When hTR is placed under the control of mediator-recruiting Pol II promoters, more abundant 3ʹ extended transcripts are produced [Citation22]. Longer transcripts are either degraded or processed into the mature form through a 3ʹ processing mechanism (discussed in greater detail throughout this review) distinct from those of snRNA and mRNA [Citation19]. Accordingly, transcription termination signals of snRNA or polyadenylation signals of mRNA placed downstream of the hTR gene, either immediately adjacent to position 451 or separated by 500 nt of hTR downstream genomic sequence, cause degradation of the hybrid hTR transcript [Citation19]. This suggests that 3ʹ processing machineries recruited by transcription termination signals of snRNA or polyadenylation signals of mRNA are not compatible with hTR processing.
The role of the 5ʹ guanosine track in hTR regulation
A track of guanosine nucleotides exists at the very 5ʹ end of hTR (), forming a G-quadruplex (G4) structure that protects hTR from degradation during the initial stage of transcription [Citation23]. The G4 structure is then resolved by the RNA helicase DHX36 to prevent the competition of the G4 structure with correct hTR folding and template boundary element formation [Citation23–25]. It was later shown that heterogeneous nuclear ribonucleoproteins F/H (hnRNP F/H), post-transcriptional regulators that bind to G-rich RNA sequences and mediate alternative splicing [Citation26,Citation27], also interact with the 5ʹ guanosine track of hTR [Citation28]. This preferentially occurs with tracks that are in a non-G4 state, which is proposed to inhibit refolding of the G4 structure and favour correct template boundary element formation.
Accumulation of hTR is ensured by the H/ACA complex
The pre-H/ACA complex containing NAF1, NOP10, NHP2, and the pseudouridine synthase dyskerin, is recruited to the H/ACA box of H/ACA RNA co-transcriptionally [Citation29]. Interaction between the pre-H/ACA complex and hTR is stimulated by the BIO box sequence (), which is a unique feature of hTR not present in other human H/ACA RNAs [Citation17]. In addition, a recent study showed that dyskerin binding to hTR is blocked by modified bases between CR4/5 and the H box, which can be resolved by the base excision repair enzyme SMUG1 [Citation30]. During the conversion from a pre-H/ACA to a mature H/ACA RNP, NAF1 is replaced by GAR1 [Citation31]. It has long been suggested that there are two sets of mature H/ACA complexes bound to hTR in the active telomerase [Citation17,Citation32], which is confirmed by cryo-EM structural analysis [Citation3]. Defects in NAF1 [Citation33], NHP2 [Citation34], NOP10 [Citation35], dyskerin [Citation36,Citation37], but not in GAR1, have been shown to reduce hTR levels, suggesting that the pre-H/ACA complex plays an important role in stabilizing hTR during the early stage of its biogenesis, at least upstream of NAF1 replacement by GAR1. It is proposed that the assembly of this complex is required for correct maturation and accumulation of hTR (discussed in the next section).
Mature H/ACA RNPs are guided to RNA substrates to catalyse pseudouridylation through base pairing between the sno/scaRNA components and target RNA substrates. In archaea, GAR1 has been shown to ensure accurate H/ACA RNP complex placement on target RNA and enhance pseudouridylation activity of dyskerin [Citation38]. However, its role in hTR biogenesis or function is still unclear. Before pre-H/ACA RNP assembly, the H/ACA RNP assembly factor, SHQ1, occupies the RNA-binding pocket of dyskerin in the cytoplasm, both to prevent non-specific binding of RNA and to stabilize dyskerin [Citation39]. Upon nuclear import, SHQ1 is dissociated from dyskerin by the R2TP complex [Citation40] containing PIH1D1, RPAP3, two proteins that are involved in target recognition; and two AAA+ ATPases pontin and reptin. The R2TP complex is previously known to cooperate with the chaperone Hsp90 to facilitate assembly of large protein complexes, such as RNA polymerase II and those containing PI-3 kinase-related protein kinases [Citation41]. In the case of hTR biogenesis, dissociation of SHQ1 from dyskerin by the R2TP complex allows pre-H/ACA complex assembly [Citation42].
Competing roles of degradation versus 3ʹ processing during hTR maturation
Mature hTR is 451 nucleotides in length. However, a subpopulation of the total hTR carrying 3ʹ extensions encoded by genomic sequences also exists, possibly due to read-through of the RNA polymerase. In steady state, about 70% to 80% of total hTR exists in the mature form [Citation43–46]. On the other hand, selective purification of nascent RNA transcripts showed that 72% to 75% of the nascent hTR produced within 4 h are extended at the 3ʹ end [Citation45]. These findings suggest that longer forms of hTR are the predominant transcriptional products, which are either processed into the mature form or degraded. This is a critical process, since the presence of even a single nucleotide downstream of position 451 of hTR reduces telomerase activity in vitro [Citation47].
The nuclear exosome complex is implicated in the degradation or processing of various RNA substrates. Its role in hTR biogenesis was first shown in early studies by Northern blot, in which the depletion of the exosome core component RRP40 or nuclease RRP6 leads to elevated intensity of a band representing mature hTR or short extended precursors [Citation44,Citation48,Citation49]. The exosome is recruited to different RNA substrates by at least three distinct adaptors: NEXT, TRAMP, and a recently identified PAXT connection [Citation50,Citation51]. Detailed reviews of the exosome and its adaptors have been recently published [Citation52–54]. In addition, the microRNA biogenesis factor DGCR8 facilitates exosome recruitment to hTR in the nucleolus [Citation55].
Substrate specificity of exosome adaptors is determined by their subcellular localization and the polyadenylation state of the target. NEXT is a nucleoplasmic exosome adaptor complex that preferentially recruits the exosome to degrade non-adenylated RNA [Citation56]. NEXT is comprised of the scaffolding protein ZCCHC8, the RNA helicase MTR4, and the RNA-binding protein RBM7 [Citation51]. It is implicated in the turnover of PROMoter uPstream Transcripts, enhancer RNA, and 3ʹ extended snRNA [Citation57–59]. Depletion of NEXT subunits ZCCHC8 or RBM7 increases the levels of hTR precursors carrying at least 50 nt of 3ʹ extension measured by qPCR, while the levels of mature or short extended products show no change on Northern blot [Citation44]. This suggests that NEXT may primarily lead to the degradation of longer hTR precursors that are not processed into the mature form (). Such long precursors are rare, since the vast majority of nascent hTR transcripts do not exceed 461 nt in length, which are likely to be processed into the mature form, given that the levels of nascent hTR only change mildly during the course of maturation [Citation45]. A more recent study reported that ZCCHC8 is implicated in the processing of hTR precursors, in which downregulation of ZCCHC8 leads to a decrease in total hTR levels and the percentage of mature hTR [Citation60]. We speculate that this is a NEXT-independent function, since ZCCHC8 depletion also increases the fraction of polyadenylated hTR, while NEXT targets are predominantly non-adenylated [Citation56]. In addition, unlike ZCCHC8, depletion of another NEXT component RBM7 increases total hTR levels measured by qPCR [Citation60]. Although earlier Northern blot analyses found that RBM7 depletion causes no change in the intensity of an RNA species representing mature hTR or short 3ʹ extended species, qPCR experiments using primers targeting a region 50 nt downstream of position 451 of hTR confirmed an increased amount of longer precursors that could account for the elevated levels of total hTR [Citation44].
Figure 2. A simplified model of hTR maturation. Dashed arrows indicate speculated pathways that will requires further investigation. Note that for simplicity, the triplex helix structure prior to binding of the H/ACA complex, the assembly of the H/ACA RNP with hTERT, and DGCR8 which facilitates the recruitment of the exosome to hTR in the nucleolus, are not included. Core exosome components other than RRP6, including RRP40, are together referred to as the core complex. (A) In the nucleoplasm, long hTR precursors are degraded through NEXT-dependent recruitment of the exosome. (B, C) In the nucleolus, polyadenylation of hTR precursors by the TRAMP complex leads to degradation by the exosome. (D) PARN mediates deadenylation of hTR precursors. (E) Deadenylated hTR precursors from 456 to 461 nt in length are either directly processed into the mature form by RRP6, or first processed to 455 nt by RRP6, followed by subsequent trimming by PARN. (F) Nucleoplasmic RRP6 might also directly process non-adenylated hTR precursors into the mature form. (G) Residual poly(A) tail and 3ʹ extension are removed by TOE1 and/or PARN in the Cajal body to complete hTR maturation. (H) ZCCHC8 might be involved in deadenylation and 3ʹ processing of short hTR precursors no longer than 461 nt in the nucleoplasm. (I) Polyadenyation of nucleoplasmic hTR precursors by PAPD5 or an unidentified poly(A) polymerase. Mature hTR either resides in the nucleoplasm or is translocated to the Cajal body by TCAB1
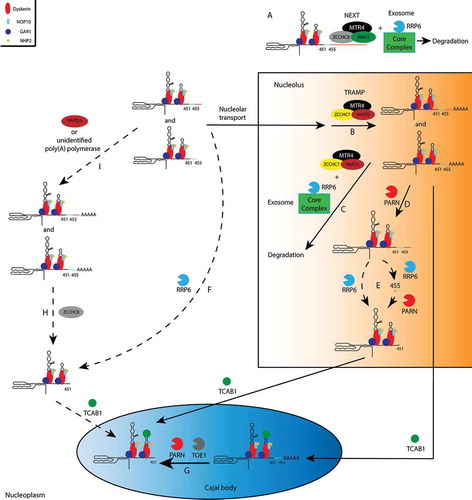
The current focus on hTR maturation has shifted to the antagonistic roles of the TRAMP complex and the poly(A)-specific ribonuclease PARN (), which are also implicated in the maturation pathway of H/ACA snoRNAs [Citation61]. The TRAMP complex is a nucleolar-specific exosome adaptor comprising the RNA helicase MTR4, the RNA-binding protein ZCCHC7, and the non-canonical poly(A) polymerase PAPD5 [Citation51]. Polyadenylation of hTR precursors by TRAMP promotes their degradation by the exosome [Citation44,Citation45,Citation48] (). On the other hand, PARN not only deadenylates hTR precursors to prevent their degradation [Citation44,Citation48,Citation49] but might also exhibit 3ʹ trimming activity [Citation43] (). Recombinant PARN has been shown to preferentially process hTR precursors no longer than 455 nt, with reduced activity towards precursors that are at least 460 nt in length, due to the attenuation of PARN activity by the CGC trinucleotide from position 456 to 458 [Citation43,Citation62–64]. Initial processing of longer precursors requires the exosome core nuclease RRP6, although an exosome-independent function of RRP6 is also speculated [Citation43]. Note that deadenylation of these long precursors might still require PARN or other deadenylases (). The activity of PARN towards hTR precursors no longer than 455 nt suggests that once longer precursors are trimmed to 455 nt, RRP6 might be replaced by PARN for further processing (). Given that PARN is localized in the nucleolus and membraneless nuclear compartments enriched for splicing factors known as Cajal Bodies (CBs) [Citation61], while RRP6 is found in both the nucleolus and nucleoplasm [Citation52–54], we speculate that if such replacement occurs, it likely takes place in the nucleolus. Another possibility is that RRP6 directly processes long precursors into mature hTR (). Interestingly, tertiary interactions between the 5ʹ hairpin, the H-box, and the 459-UUU-461 trinucleotide 3ʹ of the mature hTR end lead to the formation of a triple helix structure in vitro that inhibits RRP6 activity and leads to degradation of the hTR precursor. Binding of the H/ACA complex to the H/ACA box of hTR is proposed to disrupt the triple helix to first allow initial processing of hTR precursors longer than 455 nt by RRP6 instead of degradation [Citation43]. In support of this, an hTR mutation that is predicted to strengthen the tertiary interaction leads to processing defects in vitro and accumulation defects in cells, while nucleotide substitutions that favour disruption of the triple helix have opposite effects [Citation43]. Another proposed function of the H/ACA complex is the attenuation of excessive hTR processing and defining position 451 as the 3ʹ end of mature hTR [Citation43].
A parallel hTR maturation pathway independent of PAPD5 and PARN has recently been postulated based on maturation kinetic analysis of purified nascent transcripts subjected to 3ʹ Rapid amplification of cDNA end sequencing at different time points. The maturation kinetics of hTR in PAPD5/PARN double knockout cells is similar to that in wild type cells, while polyadenylation of nascent hTR is only mildly reduced [Citation45], implying that another ribonuclease plays a role in hTR precursor deadenylation and maturation. One candidate is TOE1 (), which has been shown to share overlapping deadenylation targets with PARN, including snoRNA, scaRNA, and hTR. On the other hand, deadenylation of 18S rRNA and snRNA is almost exclusively regulated by PARN and TOE1, respectively, due to the differences in their subcellular localization [Citation65]. TOE1 displays deadenylation and 3ʹ end processing activities towards hTR (predominantly short precursors no longer than 455 nt) in vitro and in cells [Citation47,Citation65]. However, unlike PARN deficiency, depletion of TOE1 does not significantly change total hTR levels. Additionally, a decrease of telomerase activity in TOE1-depleted cells even in the presence of PARN suggests that it has unique functions in telomerase regulation [Citation47]. Nonetheless, due to the enrichment of TOE1 in CBs [Citation47], other yet unidentified factors might be responsible for the maturation of hTR precursors in the nucleoplasm or the nucleolus in PAPD5/PARN double knockout cells.
Intriguingly, the length of the poly(A) tail of nascent hTR increases over time in PARN knockout cells [Citation45]. Concomitantly, the nuclear poly(A) binding protein PABPN1 binds hTR with longer poly(A) tails compared to those associated with hTERT or dyskerin [Citation48]. PABPN1 has previously been shown to promote degradation of polyadenylated RNA [Citation66–68]. Later studies confirm that it is a subunit of the PAXT connection, in which MTR4 stably interacts with the RNA-binding protein ZFC3H1, and PABPN1 transiently forms an interaction with MTR4-ZFC3H1, therefore leading to the term ‘connection’[Citation50]. PABPN1 recruits the exosome to target RNA by binding to the poly(A) tail. Surprisingly, PABPN1 depletion leads to decreased total hTR levels and an increased amount of 3ʹ extended precursors [Citation48]. Defects in hTR accumulation caused by PABPN1 knockdown can be rescued by co-depletion of exosome core components, suggesting that in the context of hTR maturation, PABPN1 functions in counteracting instead of facilitating exosome degradation of hTR precursors [Citation48]. In agreement with this, depletion of the PAXT-specific subunit ZFC3H1 does not change the levels of mature hTR or 3ʹ extended hTR [Citation50,Citation69]. Given that PABPN1 interacts with PARN in an RNA-independent manner [Citation48], it has been proposed that the protective function of PABPN1 in hTR maturation is achieved by recruitment of PARN to polyadenylated hTR precursor. We speculate that the gradual lengthening poly(A) tail acts as a sensor, and PABPN1 is preferentially recruited to hTR precursors with longer poly(A) tail to prioritize their maturation. However, given that PABPN1 is enriched in nuclear speckles [Citation70], while PARN is predominantly in the nucleolus and CBs [Citation61], it is still unclear in which compartment PABPN1-dependent recruitment of PARN to hTR precursors occurs.
Depletion of ZCCHC8, a nucleoplasmic component of the NEXT complex, increases the percentage of polyadenylated hTR [Citation60]. Although the TRAMP complex that polyadenylates hTR is a nucleolar-specific exosome cofactor, due to exclusive nucleolar localization of one of its components, ZCCHC7 [Citation51], another TRAMP component PAPD5 is found throughout the nucleus [Citation71]. Therefore, we speculate that nucleoplasmic PAPD5 also mediates hTR polyadenylation, which is then antagonized in a ZCCHC8-dependent manner (). However, given that hTR polyadenylation is only mildly affected in PARN/PAPD5 double knockout cells, it is also possible that a yet unidentified poly(A) polymerase (PAP) mediates hTR polyadenylation (). Canonical PAPs are unlikely to catalyse hTR polyadenylation, since polyadenylation signal is incompatible with hTR processing [Citation19]. In addition, depletion of canonical PAPs decreases hTR levels [Citation48], which is inconsistent with the role of polyadenylation in promoting hTR degradation. Given that PAPD5 is a homolog of yeast Trf4, we speculate that another Trf4 homolog in human, PAPD7 [Citation72], might also be involved in hTR polyadenylation.
The cap-binding complex (CBC) has previously been shown to recruit the exosome through NEXT and PAXT [Citation50,Citation59]. Depletion of CBC components increases the fraction of mature hTR while the percentage of polyadenylated hTR is decreased [Citation44]. The authors suggest that CBC might inhibit PARN activity towards polyadenylated hTR precursors, which has previously been shown for mRNA [Citation73].
Regulation of hTR localization
Results from conventional fluorescence in situ hybridization (FISH) show that throughout most of the cell cycle, hTR resides in CBs [Citation74,Citation75]. CBs also facilitate the recruitment of telomerase to telomeres [Citation76,Citation77], albeit they are not strictly necessary for this process, since telomere homeostasis can be maintained in human cells lacking the CB structural protein coilin [Citation78]. Localization of hTR to CBs requires binding of TCAB1 to the evolutionarily conserved CAB box sequence in the 3ʹ hairpin loop of hTR, which is also found in other H/ACA scaRNAs [Citation16,Citation75,Citation79]. Depletion of TCAB1 abolishes CB localization of hTR without affecting hTR levels or telomerase assembly [Citation79–81]. In addition, Nopp140, an intrinsically disordered protein found in both the nucleolus and CBs, is required for CB localization of H/ACA scaRNPs and TCAB1 [Citation82]. The idea that hTR is predominantly localized in CBs is challenged by a most recent study applying hTR live cell imaging, which demonstrates that only 5% to 10% of hTR is found in CBs in asynchronized cells [Citation83]. This is further confirmed by a highly sensitive FISH technique combined with super-resolution microscopy in the same study. hTR is transiently present in the nucleolus, which might represent precursor processing. The differences in live cell imaging/high-sensitivity FISH and conventional FISH could be caused by the extended residence time of hTR in CBs [Citation83] and detection limits of conventional FISH.
In mid-S-phase, the frequency of hTR and hTERT localization to telomeres peaks, indicative of telomerase recruitment to telomeres. In previous studies, CBs are found to be adjacent to hTR-containing telomere foci but not hTR-free telomeres, which would suggest that CBs may transport telomerase proximal to telomeres [Citation77,Citation84]. However, hTR live cell imaging shows that hTR recruitment to telomeres is not exclusively occurring in CBs, and overexpression of hTERT further reduces the fraction of hTR in CBs[Citation83]. In addition, depletion of coilin does not impair telomere localization of hTR [Citation78], in agreement with the non-essential role of CBs in transporting telomerase to telomeres.
Live cell imaging of fluorescently tagged hTERT and the telomeric DNA-binding protein TRF2 reveals that hTERT engages in distinct short and long interactions with telomeres, reflecting transient telomere probing and static elongation states. The short and long interaction requires hTERT binding to TPP1, a protein responsible for recruiting telomerase to telomeres [Citation85,Citation86]. An hTERT mutant defective in interacting with TPP1 shows impaired colocalization with TRF2 [Citation87] and inhibits hTR recruitment to telomeres [Citation83]. Once telomerase is localized at telomeres, hTR displays two patterns of movement with fast and slow diffusion rates, which represent substrate scanning and engaged base pairing with telomeric DNA, respectively [Citation83]. In the future, it will be interesting to apply live cell imaging in the analysis of hTR localization in different stages of the cell cycle.
Interestingly, aberrant localization of hTR in the cytoplasm has previously been observed when accumulation and/or processing defects are induced by depletion of dyskerin or PARN [Citation49]. This is unexpected, since previous results suggest that hTR biogenesis does not involve nuclear export [Citation88]. Nuclear localization of hTR in dyskerin-depleted and PARN-depleted cells can be restored by co-depletion of RRP6 and the cytoplasmic decapping factor DCP2, respectively [Citation49]. It is still unclear whether cytoplasmic hTR represents precursors or the mature form. The functional interaction between hTR processing, nuclear export factors, and cytoplasmic RNA turnover machineries requires further investigation.
The role of 5ʹ cap hypermethylation in regulating hTR localization and accumulation
Nuclear export of mRNA and snRNA requires interaction between the 5ʹ monomethylguanosine (MMG) cap and CBC, which then recruits export factors corresponding to the RNA type [Citation89,Citation90]. The MMG cap on precursor snRNAs recruits CBC, the adaptor protein PHAX, and the nuclear export factor CRM1 for translocation to the cytoplasm [Citation90–92], where the MMG cap of snRNA is hypermethylated to [Citation2,Citation7]-trimethylguanosine (TMG) by full-length trimethylguanosine synthase 1 TGS1 (TGS1-LF), which ensures reimport of the snRNP into the nucleus [Citation93]. The transport of snoRNA has been studied using C/D box snoRNA, a class of snoRNA that carries the C/D box secondary structure, as a model. Unlike snRNA, PHAX directs MMG-capped C/D box snoRNA precursors into CBs, where MMG is hypermethylated to TMG to mediate CBC-independent recruitment of CRM1 to C/D box snoRNA. C/D box snoRNPs are sequestered in CBs by TGS1-LF, while CRM1 dissociates TGS1-LF and allows translocation of the RNP into the nucleolus [Citation88,Citation94]. Note that TGS1-LF binds to both capped and uncapped C/D box snoRNAs, indicating a hypermethylation-independent function [Citation88]. In this case, cap hypermethylation of C/D box snoRNP in CBs is more likely to be catalysed by a shorter form of TGS1, which is the proteasomal processing product of TGS1-LF [Citation93].
Compared to C/D box snoRNA, the interplay between cap hypermethylation and nuclear transport/export of H/ACA snoRNAs and scaRNAs in human cells, including hTR, is less well understood. A recent study reported that TGS1 is involved in hypermethylating the cap of hTR [Citation95]. Depletion of TGS1 causes cytoplasmic accumulation of hTR. However, inhibition of CRM1 by leptomycin B (LMB) treatment does not alter the distribution of hTR between the cytoplasm and the nucleus in both control and TGS1-deficient cells [Citation95], thus the factors regulating hTR nuclear export upon TGS1 depletion remain unidentified. The nuclear fraction of hTR reveals a subcellular localization defect with nucleolar accumulation of hTR [Citation95]. This is either caused by disrupted CB formation or intrinsic defects in the CB translocation machineries in TGS1-deficient cells.
Despite the aberrant localization patterns, total hTR levels are increased by TGS1 depletion, without altering the percentage of 3ʹ extended and oligoadenylated hTR species, which is possibly due to the separation of cytoplasmic hTR from both nuclear RNA degradation and deadenylation/processing machineries. CRM1 inhibition increases total hTR levels in control cells but not in TGS1-null cells [Citation95]. Given that hTR distribution between the cytoplasm and the nucleus is not altered by CRM1 inhibition regardless of TGS1 expression state, the authors suggest that TGS1 and CRM1 function epistatically in regulating hTR levels through the intranuclear transport pathway. In TGS1-null cells, although cytoplasmic hTR is not associated with hTERT, nuclear hTR shows no defect in telomerase assembly. Telomerase activity and telomere length in TGS1-null cells increase compared to control cells, while most of the hTR molecules associated with hTERT do not contain the TMG cap, suggesting that hypermethylation of the hTR cap is dispensable for telomerase assembly and function [Citation95].
Interestingly, TGS1 depletion enhances the interaction between SmB protein and nuclear hTR, but not with cytoplasmic hTR [Citation95]. SmB is a component of the Sm protein complex that binds to cytoplasmic snRNAs and promotes their nuclear reimport [Citation96–98]. SmB has previously been found to be associated with hTR in a CAB box-dependent manner [Citation99], but the function of this interaction remains unclear. Finally, whether these effects are mediated by TGS1-LF or the short form requires further studies.
Assembly of hTR and hTERT
hTR and hTERT localization in the nucleolus peaks in early S phase, which was originally proposed to facilitate telomerase assembly [Citation100]. However, other studies reported that hTR and hTERT are localized in different compartment of the nucleolus [Citation84], or hTERT is found in the nucleolus in early S phase when expressed in primary cells but excluded from the nucleolus throughout the cell cycle in transformed cell lines [Citation101]. Another model suggests that hTR is assembled with hTERT in CBs [Citation83,Citation84]. However, observations that TCAB1 depletion does not affect telomerase assembly [Citation79,Citation80] implies that a compensatory CB-independent telomerase assembly pathway might exist. The mechanisms of telomerase assembly require further investigation.
Interaction between hTR and hTERT regulates telomerase activity
hTR contains the necessary template for synthesizing telomeric DNA by reverse transcription, therefore its interaction with hTERT is important for telomerase activity. Regions in hTR mediating interaction with hTERT have been reviewed previously [Citation6,Citation102]. In brief, the pseudoknot and CR4/5 interact with hTERT independently [Citation103,Citation104], and both of them are required for maintaining telomerase activity [Citation104]. Recent advances have been made towards identifying the factors mediating hTR-hTERT interaction. The RNA-binding protein HuR, which is implicated in post-transcriptional modification of mRNA, is found to associate with hTR and promote methylation of C106 by a yet to be identified RNA methyltransferase, possibly by altering the secondary structure of hTR. This modification enhances the hTR-hTERT interaction as well as telomerase activity [Citation105].
A small RNA arising from nucleotide 425–447 of hTR (hTR-sRNA) is associated with AGO2, one of the AGO proteins that is involved in microRNA-induced silencing of mRNA [Citation106]. hTR-sRNA recruits AGO2 to hTR through base pairing, which is predicted to occur at positions 12–31 and 313–340 of hTR. AGO2 enhances the hTR-hTERT interaction and telomerase activity without affecting hTR levels. Furthermore, overexpression of hTR-sRNA increases telomerase activity even in the absence of AGO2, implying that other AGO proteins might compensate for the functions of AGO2 in telomerase regulation [Citation107]. However, the biogenesis pathway of hTR-sRNA remains elusive.
Earlier reports applying incomplete depletion of TCAB1 through RNA interference-based methods led to the observation that TCAB1 knockdown does not reduce telomerase activity [Citation79]. In a more recent study, TCAB1 knockout using a CRISPR-Cas9 system was found to impair telomerase activity. Further analysis uncovered a novel function of TCAB1 in mediating the folding of P6b and P6.1 loops () of hTR, thereby optimizing the interaction between the CR4/5 region and hTERT [Citation80]. Note that telomerase activity is not completely abolished upon TCAB1 knockout, which might explain why only hTERT and hTR are needed for telomerase activity in vitro [Citation4], and why a minimal hTR containing only the pseudoknot region and CR4/5 but without the CAB box can maintain telomere homeostasis when hTERT is overexpressed [Citation108]. In agreement with the important role of the P6.1 loop in mediating the interaction with hTERT, several nucleotides in this region are highly conserved and their substitution reduces telomerase activity [Citation109]. Two of these conserved nucleotides, U306 and U307, are found to be pseudouridylated (Ψ) [Citation110,Citation111], a modification that has been shown to promote tertiary interactions in tRNA [Citation112]. The presence of Ψ306 and Ψ307 rearranges the P6.1 loop conformation as is revealed by solution structure analysis [Citation110]. It will be interesting to explore the possibility that pseudouridylation of nucleotides in P6.1 mediates the interaction between hTR and hTERT.
Mutations in hTR or hTR regulatory proteins lead to telomere maintenance disorders
Defects in hTR accumulation, interaction with hTERT, or recruitment to telomeres can cause telomere maintenance defects, manifested as premature ageing and accompanied by other symptoms. Mutations in the promoter, the template region, pseudoknot, CR4/5, and the H/ACA motif of hTR have previously been identified in patients with dyskeratosis congenita (DC), Hoyeraal-Hreidarsson syndrome (HHS), familial idiopathic pulmonary fibrosis (IPF), and aplastic anaemia [Citation113–117]. Mutations in dyskerin, NOP10, NHP2, and NAF1 that affect either subcellular localization or protein–protein/protein–RNA interactions disrupt the assembly of the pre-H/ACA complex on hTR and account for hTR accumulation defects [Citation33–37,Citation118,Citation119]. TCAB1, the CB localization factor, is mutated in an autosomal recessive form of DC [Citation81]. Mutations in PARN, the nuclease essential for deadenylation, 3ʹ processing, and accumulation of hTR, are linked to DC and IPF [Citation120–122]. In addition, a mutant form of ZCCHC8, a component of the exosome adaptor NEXT, was recently discovered in IPF patients along with its novel functions in hTR maturation [Citation60]. TOE1, the nuclease recently shown to have deadenylation and 3ʹ processing activity towards hTR [Citation47,Citation65], is associated with pontocerebellar hypoplasia, a rare neurodegenerative disorder that is also present in HHS [Citation123].
Non-canonical functions of hTR
Other than the previously described hTERT-independent function in regulating the ATR DNA damage response signalling pathway [Citation124], hTR is found to translocate to the mitochondria, where it is processed into a shorter form containing nucleotide 53 to 247, which is subsequently exported to the cytoplasm and serves as an indicator of mitochondrial functions [Citation125]. Overexpression of the processed form of hTR causes accelerated senescence without altering telomerase activity [Citation126], but the underlying mechanism requires further investigation.
An open reading frame encoding a small peptide is predicted to exist in vertebrate telomerase RNA, including hTR [Citation127]. In human cells, the presence of this peptide (hTERP) is detected by immunofluorescence, Western blot, and mass spectrometry in telomerase positive cells but not in hTR-negative VA13 cells. Overexpression of WT hTR but not mutants defective in producing hTERP confers resistance against drug-induced apoptosis [Citation127], consistent with a documented telomerase-independent function of hTR in promoting cell survival [Citation128].
Conclusion and future direction
hTR is sophisticatedly regulated at different steps of its transcription, maturation, assembly into the telomerase complex, and recruitment to telomeres. The current knowledge of hTR maturation and localization is summarized in . hTR precursors are first processed in the nucleolus by PARN [Citation43–45,Citation48,Citation122] and/or possibly in the nucleoplasm in a ZCCHC8- and/or RRP6-dependent manner [Citation43,Citation60]. In addition, the recently discovered deadenylation and 3ʹ processing activity of the CB-specific exonuclease TOE1 towards hTR indicates an extra step of removing residual poly(A) tail and 3ʹ extension in CBs [Citation47,Citation65]. Finally, mature hTR either resides in the nucleoplasm or is translocated to CBs, until recruitment to telomeres in S phase.
Despite the valuable insights provided by some of the latest discoveries, the mechanisms underlying hTR subcellular localization are still not fully understood. A recent study suggests that CRM1 might cooperate with TGS1 to regulate hTR levels and intranuclear transport but not nuclear export [Citation95]. Previously, CRM1 inhibition has been shown to increase the interaction between hTR and TGS1-LF [Citation88], a protein that sequesters C/D box snoRNP in CBs and needs to be dissociated by CRM1 to allow nucleolar import. Although it has been shown that 3ʹ extended hTR precursors accumulate in the nucleolus or nucleoplasm when processing defects are induced [Citation48,Citation129], further studies are required to investigate whether they also undergo CB to nucleolus transport during biogenesis, as is the case in C/D box snoRNA [Citation88]. In addition, the previously overlooked cytoplasmic presence of hTR has been suggested by recent studies. Mitochondria-related functions of hTR indicate that it is exported from the nucleus, contrary to a previous observation that hTR is excluded from the cytoplasm [Citation88]. However, it is also possible that the probe used previously is not sufficiently sensitive to detect low levels of cytoplasmic hTR. Indeed, hTR has been detected in the cytoplasm by fractionation [Citation95,Citation127], although possible leakage of hTR from the nuclear fraction should also be considered. It is likely that further questions can be answered by hTR live cell imaging in the future.
It has long been known that binding of the H/ACA complex to the H/ACA box promotes hTR accumulation. It is now clear that it attenuates excessive 3ʹ processing that will lead to degradation, and correctly defines the hTR 3ʹ end [Citation43]. Intriguingly, upon PARN depletion, hTR accumulation is compromised even in the presence of the H/ACA complex [Citation44,Citation45,Citation48,Citation49,Citation127], which is unlikely due to defects in recruiting the H/ACA complex, since this process is co-transcriptional [Citation29] and upstream of PARN function. In addition, even though the triple helix structure of hTR prior to binding of the H/ACA complex could resist RRP6 activity, mutations that strengthen the triplex helix lead to reduced instead of increased hTR levels [Citation43]. These results together imply the existence of an unidentified factor that can overcome the triple helix structure or the H/ACA complex to degrade hTR.
Finally, understanding the mechanisms regulating hTR degradation and maturation gives rise to a possible treatment option for telomere maintenance disorders that aims to increase hTR levels. PAPD5 depletion rescues the defects in hTR levels, hTR maturation, telomerase activity, telomere length, and haematopoietic potential of human embryonic stem cells carrying a mutation in dyskerin [Citation130]. More importantly, a PAPD5 inhibitor achieves similar restoration in cultured patient cells or xenografted human cells in mice [Citation131], demonstrating the potential to treat patients suffering from premature ageing telomere maintenance syndromes by targeting hTR degradation/maturation.
Abbreviations
Acknowledgments
We thank Deanna MacNeil, Patrick Lambert-Lanteigne, and Adrian Young for discussion and feedback. Financial support was provided from a Canadian Institute for Health Research Grant to C.A. Support for J.Q. was provided by a McGill University Faculty of Medicine Internal Studentship.
Disclosure statement
The authors declare no conflict of interest.
Additional information
Funding
References
- Harley CB, Futcher AB, Greider CW. Telomeres shorten during ageing of human fibroblasts. Nature. 1990;345:458–460.
- Armanios M, Blackburn EH. The telomere syndromes. Nat Rev Genet. 2012;13:693–704.
- Nguyen THD, Tam J, Wu RA, et al. Cryo-EM structure of substrate-bound human telomerase holoenzyme. Nature. 2018;557:190–195.
- Weinrich SL, Pruzan R, Ma L, et al. Reconstitution of human telomerase with the template RNA component hTR and the catalytic protein subunit hTRT. Nat Genet. 1997;17:498–502.
- Stewart SA, Weinberg RA. Telomeres: cancer to Human Aging. Annu Rev Cell Dev Biol. 2006;22:531–557.
- Schmidt JC, Cech TR. Human telomerase: biogenesis, trafficking, recruitment, and activation. Genes Dev. 2015;29:1095–1105.
- Roake CM, Artandi SE. Regulation of human telomerase in homeostasis and disease. Nat Rev Mol Cell Biol. 2020;21:384–397.
- Theimer CA, Feigon J. Structure and function of telomerase RNA. Curr Opin Struct Biol. 2006;16:307–318.
- Chen JL, Greider CW. An emerging consensus for telomerase RNA structure. Proc Natl Acad Sci U S A. 2004;101:14683–14684.
- Webb CJ, Zakian VA. Identification and characterization of the Schizosaccharomyces pombe TER1 telomerase RNA. Nat Struct Mol Biol. 2008;15:34–42.
- Leonardi J, Box JA, Bunch JT, et al. TER1, the RNA subunit of fission yeast telomerase. Nat Struct Mol Biol. 2008;15:26–33.
- Seto AG, Zaug AJ, Sobel SG, et al. Saccharomyces cerevisiae telomerase is an Sm small nuclear ribonucleoprotein particle. Nature. 1999;401:177–180.
- Mitchell JR, Cheng J, Collins KA, et al. ACA small nucleolar RNA-like domain at the human telomerase RNA 3′ end. Mol Cell Biol. 1999;19:567–576.
- Matera AG, Terns RM, Terns MP. Non-coding RNAs: lessons from the small nuclear and small nucleolar RNAs. Nat Rev Mol Cell Biol. 2007;8:209–220.
- Chen JL, Blasco MA, Greider CW. Secondary structure of vertebrate telomerase RNA. Cell. 2000;100:503–514.
- Richard P, Darzacq X, Bertrand E, et al. A common sequence motif determines the Cajal body-specific localization of box H/ACA scaRNAs. Embo J. 2003;22:4283–4293.
- Egan ED, Collins K. An enhanced H/ACA RNP assembly mechanism for human telomerase RNA. Mol Cell Biol. 2012;32:2428–2439.
- Feng J, Funk W, Wang S, et al. The RNA component of human telomerase. Science. 1995;269:1236–1241.
- Fu D, Collins K. Distinct biogenesis pathways for human telomerase RNA and H/ACA small nucleolar RNAs. Mol Cell. 2003;11:1361–1372.
- Soutourina J. Transcription regulation by the Mediator complex. Nat Rev Mol Cell Biol. 2018;19:262–274.
- Skaar JR, Ferris AL, Wu X, et al. The Integrator complex controls the termination of transcription at diverse classes of gene targets. Cell Res. 2015;25:288–305.
- Rubtsova MP, Vasilkova DP, Moshareva MA, et al. Integrator is a key component of human telomerase RNA biogenesis. Sci Rep. 2019;9:1–10.
- Sexton AN, Collins K. The 5ʹ guanosine tracts of human telomerase RNA are recognized by the G-quadruplex binding domain of the RNA helicase DHX36 and function to increase RNA accumulation. Mol Cell Biol. 2011;31:736–743.
- Lattmann S, Stadler MB, Vaughn JP, et al. The DEAH-box RNA helicase RHAU binds an intramolecular RNA G-quadruplex in TERC and associates with telomerase holoenzyme. Nucleic Acids Res. 2011;39:9390–9404.
- Booy EP, Meier M, Okun N, et al. The RNA helicase RHAU (DHX36) unwinds a G4-quadruplex in human telomerase RNA and promotes the formation of the P1 helix template boundary. Nucleic Acids Res. 2012;40:4110–4124.
- Garneau D, Revil T, Fisette JF, et al. Heterogeneous nuclear ribonucleoprotein F/H proteins modulate the alternative splicing of the apoptotic mediator Bcl-x. J Biol Chem. 2005;280:22641–22650.
- Dominguez C, Fisette JF, Chabot B, et al. Structural basis of G-tract recognition and encaging by hnRNP F quasi-RRMs. Nat Struct Mol Biol. 2010;17:853–861.
- Xu C, Xie N, Su Y, et al. HnRNP F/H associate with hTERC and telomerase holoenzyme to modulate telomerase function and promote cell proliferation. Cell Death Differ. 2019;27:1998–2013.
- Darzacq X, Kittur N, Roy S, et al. Stepwise RNP assembly at the site of H/ACA RNA transcription in human cells. J Cell Biol. 2006;173:207–218.
- Kroustallaki P, Lirussi L, Carracedo S, et al. SMUG1 promotes telomere maintenance through telomerase RNA processing. Cell Rep. 2019;28:1690–1702.e10.
- Leulliot N, Godin KS, Hoareau-Aveilla C, et al. The box H/ACA RNP assembly factor Naf1p contains a domain homologous to Gar1p mediating its interaction with Cbf5p. J Mol Biol. 2007;371:1338–1353.
- Egan ED, Collins K. Specificity and stoichiometry of subunit interactions in the human telomerase holoenzyme assembled in vivo. Mol Cell Biol. 2010;30:2775–2786.
- Stanley SE, Gable DL, Wagner CL, et al. Loss-of-function mutations in the RNA biogenesis factor NAF1 predispose to pulmonary fibrosis–emphysema. Sci Transl Med. 2016;8:351ra107–351ra107.
- Vulliamy T, Beswick R, Kirwan M, et al. Mutations in the telomerase component NHP2 cause the premature ageing syndrome dyskeratosis congenita. Proc Natl Acad Sci U S A. 2008;105:8073–8078.
- Walne AJ, Vulliamy T, Marrone A, et al. Genetic heterogeneity in autosomal recessive dyskeratosis congenita with one subtype due to mutations in the telomerase-associated protein NOP10. Hum Mol Genet. 2007;16:1619–1629.
- Zeng XL, Thumati NR, Fleisig HB, et al. The accumulation and not the specific activity of telomerase ribonucleoprotein determines telomere maintenance deficiency in X-linked dyskeratosis congenita. Hum Mol Genet. 2012;21:721–729.
- MacNeil DE, Lambert-Lanteigne P, Autexier C. N-terminal residues of human dyskerin are required for interactions with telomerase RNA that prevent RNA degradation. Nucleic Acids Res. 2019;47:5368–5380.
- Wang P, Yang L, Gao YQ, et al. Accurate placement of substrate RNA by Gar1 in H/ACA RNA-guided pseudouridylation. Nucleic Acids Res. 2015;43:7207–7216.
- Walbott H, Machado-Pinilla R, Liger D, et al. The H/ACA RNP assembly factor SHQ1 functions as an RNA mimic. Genes Dev. 2011;25:23982408.
- Machado-Pinilla R, Liger D, Leulliot N, et al. Mechanism of the AAA+ ATPases pontin and reptin in the biogenesis of H/ACA RNPs. RNA. 2012;18:1833–1845.
- Kakihara Y, Houry WA. The R2TP complex: discovery and functions. Biochim Biophys Acta Mol Cell Res. 2012;1823:101–107.
- Grozdanov PN, Roy S, Kittur N, et al. SHQ1 is required prior to NAF1 for assembly of H/ACA small nucleolar and telomerase RNPs. RNA. 2009;15:1188–1197.
- Tseng CK, Wang HF, Schroeder MR, et al. The H/ACA complex disrupts triplex in hTR precursor to permit processing by RRP6 and PARN. Nat Commun. 2018;9:1–12.
- Tseng CK, Wang H-F, Burns A, et al. Human telomerase RNA processing and quality control. Cell Rep. 2015;13:2232–2243.
- Roake CM, Chen L, Chakravarthy AL, et al. Disruption of telomerase RNA maturation kinetics precipitates disease. Mol Cell. 2019;74:688–700.e3.
- Goldfarb KC, Cech TR. 3′ terminal diversity of MRP RNA and other human noncoding RNAs revealed by deep sequencing. BMC Mol Biol. 2013;14:23.
- Deng T, Huang Y, Weng K, et al. TOE1 acts as a 3′ exonuclease for telomerase RNA and regulates telomere maintenance. Nucleic Acids Res. 2019;47:391–405.
- Nguyen D, Grenier St-Sauveur V, Bergeron D, et al. A polyadenylation-dependent 3′ end maturation pathway is required for the synthesis of the human telomerase RNA. Cell Rep. 2015;13:2244–2257.
- Shukla S, Schmidt JC, Goldfarb KC, et al. Inhibition of telomerase RNA decay rescues telomerase deficiency caused by dyskerin or PARN defects. Nat Struct Mol Biol. 2016;23:286–292.
- Meola N, Domanski M, Karadoulama E, et al. Identification of a nuclear exosome decay pathway for processed transcripts. Mol Cell. 2016;64:520–533.
- Lubas M, Christensen M, Kristiansen M, et al. Interaction profiling identifies the human nuclear exosome targeting complex. Mol Cell. 2011;43:624–637.
- Schmid M, Jensen TH. The nuclear RNA exosome and its cofactors. Adv Exp Med Biol. 2019;1203:113–132.
- Zinder JC, Lima CD. Targeting RNA for processing or destruction by the eukaryotic RNA exosome and its cofactors. Genes Dev. 2017;31:88–100.
- Kilchert C, Wittmann S, Vasiljeva L. The regulation and functions of the nuclear RNA exosome complex. Nat Rev Mol Cell Biol. 2016;17:227–239.
- Macias S, Cordiner RA, Gautier P, et al. DGCR8 acts as an adaptor for the exosome complex to degrade double-stranded structured RNAs. Mol Cell. 2015;60:873–885.
- Wu G, Schmid M, Rib L, et al. A two-layered targeting mechanism underlies nuclear RNA sorting by the human exosome. Cell Rep. 2020;30:2387–2401.e5.
- Lubas M, Andersen P, Schein A, et al. The human nuclear exosome targeting complex is loaded onto newly synthesized RNA to direct early ribonucleolysis. Cell Rep. 2015;10:178–192.
- Andersson R, Gebhard C, Miguel-Escalada I, et al. An atlas of active enhancers across human cell types and tissues. Nature. 2014;507:455–461.
- Andersen PR, Domanski M, Kristiansen MS, et al. The human cap-binding complex is functionally connected to the nuclear RNA exosome. Nat Struct Mol Biol. 2013;20:1367–1376.
- Gable DL, Gaysinskaya V, Atik CC, et al. ZCCHC8, the nuclear exosome targeting component, is mutated in familial pulmonary fibrosis and is required for telomerase RNA maturation. Genes Dev. 2019;33:1381–1396.
- Berndt H, Harnisch C, Rammelt C, et al. Maturation of mammalian H/ACA box snoRNAs: PAPD5-dependent adenylation and PARN-dependent trimming. RNA. 2012;18:958–972.
- Aström J, Aström A, Virtanen A. In vitro deadenylation of mammalian mRNA by a HeLa cell 3′ exonuclease. Embo J. 1991;10:3067–3071.
- Henriksson N, Nilsson P, Wu M, et al. Recognition of adenosine residues by the active site of poly(A)-specific ribonuclease. J Biol Chem. 2010;285:163–170.
- Virtanen A, Henriksson N, Nilsson P, et al. Poly(A)-specific ribonuclease (PARN): an allosterically regulated, processive and mRNA cap-interacting deadenylase. Crit Rev Biochem Mol Biol. 2013;48:192–209.
- Son A, Park JE, Kim VN. PARN and TOE1 constitute a 3′ end maturation module for nuclear non-coding RNAs. Cell Rep. 2018;23:888–898.
- Bresson SM, Hunter OV, Hunter AC, et al. Canonical Poly(A) polymerase activity promotes the decay of a wide variety of mammalian nuclear RNAs. PLoS Genet. 2015;11:e1005610.
- Bresson SM, Conrad NK. The human nuclear Poly(A)-binding protein promotes RNA hyperadenylation and decay. PLoS Genet. 2013;9:e1003893.
- Beaulieu YB, Kleinman CL, Landry-Voyer AM, et al. Polyadenylation-dependent control of long noncoding RNA expression by the Poly(A)-binding protein nuclear 1. PLoS Genet. 2012;8:e1003078.
- Meola N, Jensen TH. Targeting the nuclear RNA exosome: poly(A) binding proteins enter the stage. RNA Biol. 2017;14:820–826.
- Bengoechea R, Tapia O, Casafont I, et al. Nuclear speckles are involved in nuclear aggregation of PABPN1 and in the pathophysiology of oculopharyngeal muscular dystrophy. Neurobiol Dis. 2012;46:118–129.
- Rammelt C, Bilen B, Zavolan M, et al. PAPD5, a noncanonical poly(A) polymerase with an unusual RNA-binding motif. RNA. 2011;17:1737–1746.
- Ogami K, Cho R, Hoshino S. ichi. Molecular cloning and characterization of a novel isoform of the non-canonical poly(A) polymerase PAPD7. Biochem Biophys Res Commun. 2013;432:135–140.
- Balatsos NAA, Nilsson P, Mazza C, et al. Inhibition of mRNA deadenylation by the nuclear cap binding complex (CBC. J Biol Chem. 2006;281:4517–4522.
- Zhu Y, Tomlinson RL, Lukowiak AA, et al. Telomerase RNA accumulates in cajal bodies in human cancer cells. Mol Biol Cell. 2004;15:81–90.
- Jády BE, Bertrand E, Kiss T. Human telomerase RNA and box H/ACA scaRNAs share a common Cajal body-specific localization signal. J Cell Biol. 2004;164:647–652.
- Cristofari G, Adolf E, Reichenbach P, et al. Human telomerase RNA accumulation in cajal bodies facilitates telomerase recruitment to telomeres and telomere elongation. Mol Cell. 2007;27:882–889.
- Jády BE, Richard P, Bertrand E, et al. Cell cycle-dependent recruitment of telomerase RNA and cajal bodies to human telomeres. Mol Biol Cell. 2006;17:944–954.
- Chen Y, Deng Z, Jiang S, et al. Human cells lacking coilin and Cajal bodies are proficient in telomerase assembly, trafficking and telomere maintenance. Nucleic Acids Res. 2015;43:385–395.
- Venteicher AS, Abreu EB, Meng Z, et al. A human telomerase holoenzyme protein required for Cajal body localization and telomere synthesis. Science. 2009;323:644–648.
- Chen L, Roake CM, Freund A, et al. An activity switch in human telomerase based on RNA conformation and shaped by TCAB1. Cell. 2018;174:218–230.e13.
- Zhong F, Savage SA, Shkreli M, et al. Disruption of telomerase trafficking by TCAB1 mutation causes dyskeratosis congenita. Genes Dev. 2011;25:11–16.
- Bizarro J, Bhardwaj A, Smith S, et al. Nopp140-mediated concentration of telomerase in Cajal bodies regulates telomere length. Mol Biol Cell. 2019;30:3136–3150.
- Laprade H, Querido E, Smith MJ, et al. Single-molecule imaging of telomerase RNA reveals a recruitment – retention model for telomere elongation. Mol Cell. 2020;79:115–126.
- Tomlinson RL, Ziegler TD, Supakorndej T, et al. Cell cycle-regulated trafficking of human telomerase to telomeres. Mol Biol Cell. 2006;17:955–965.
- Abreu E, Aritonovska E, Reichenbach P, et al. TIN2-tethered TPP1 recruits human telomerase to telomeres in vivo. Mol Cell Biol. 2010;30:2971–2982.
- Xin H, Liu D, Wan M, et al. TPP1 is a homologue of ciliate TEBP-β and interacts with POT1 to recruit telomerase. Nature. 2007;445:559–562.
- Schmidt JC, Zaug AJ, Cech TR. Live cell imaging reveals the dynamics of telomerase recruitment to telomeres. Cell. 2016;166:1188–1197.e9.
- Pradet-Balade B, Girard C, Boulon S, et al. CRM1 controls the composition of nucleoplasmic pre-snoRNA complexes to licence them for nucleolar transport. Embo J. 2011;30:2205–2218.
- Masuda S, Das R, Cheng H, et al. Recruitment of the human TREX complex to mRNA during splicing. Genes Dev. 2005;19:1512–1517.
- Ohno M, Segref A, Bachi A, et al. PHAX, a mediator of U snRNA nuclear export whose activity is regulated by phosphorylation. Cell. 2000;101:187–198.
- Izaurralde E, Lewis J, Gamberi C, et al. A cap-binding protein complex mediating U snRNA export. Nature. 1995;376:709–712.
- Fornerod M, Ohno M, Yoshida M, et al. CRM1 is an export receptor for leucine-rich nuclear export signals. Cell. 1997;90:1051–1060.
- Girard C, Verheggen C, Neel H, et al. Characterization of a short isoform of human Tgs1 hypermethylase associating with small nucleolar ribonucleoprotein core proteins and produced by limited proteolytic processing. J Biol Chem. 2008;283:2060–2069.
- Boulon S, Verheggen C, Jady BE, et al. PHAX and CRM1 are required sequentially to transport U3 snoRNA to nucleoli. Mol Cell. 2004;16:777–787.
- Chen L, Roake CM, Galati A, et al. Loss of human TGS1 hypermethylase promotes increased telomerase RNA and telomere elongation. Cell Rep. 2020;30:1358–1372.e5.
- Meister G, Eggert C, Fischer U. SMN-mediated assembly of RNPs: A complex story. Trends Cell Biol. 2002;12:472–478.
- Kambach C, Walke S, Young R, et al. Crystal structures of two Sm protein complexes and their implications for the assembly of the spliceosomal snRNPs. Cell. 1999;96:375–387.
- Paushkin S, Gubitz AK, Massenet S, et al. The SMN complex, an assemblyosome of ribonucleoproteins. Curr Opin Cell Biol. 2002;14:305–312.
- Fu D, Collins K. Human telomerase and Cajal body ribonucleoproteins share a unique specificity of Sm protein association. Genes Dev. 2006;20:531–536.
- Lee JH, Lee YS, Jeong SA, et al. Catalytically active telomerase holoenzyme is assembled in the dense fibrillar component of the nucleolus during S phase. Histochem Cell Biol. 2014;141:137–152.
- Wong JMY, Kusdra L, Collins K. Subnuclear shuttling of human telomerase induced by transformation and DNA damage. Nat Cell Biol. 2002;4:731–736.
- MacNeil DE, Bensoussan HJ, Autexier C. Telomerase regulation from beginning to the end. Genes (Basel). 2016;7:64.
- Bachand F, Autexier C. Functional regions of human telomerase reverse transcriptase and human telomerase RNA required for telomerase activity and RNA-protein interactions. Mol Cell Biol. 2001;21:1888–1897.
- Mitchell JR, Collins K. Human telomerase activation requires two independent interactions between telomerase RNA and telomerase reverse transcriptase. Mol Cell. 2000;6:361–371.
- Tang H, Huang L, Tang Y, et al. HuR regulates telomerase activity through TERC methylation. Nat Commun. 2018;9. DOI:10.1038/s41467-018-03728-5
- Liu J, Carmell MA, Rivas FV, et al. Argonaute2 is the catalytic engine of mammalian RNAi. Science. 2004;305:1437–1441.
- Laudadio I, Orso F, Azzalin G, et al. AGO 2 promotes telomerase activity and interaction between the telomerase components TERT and TERC. EMBO Rep. 2019;20. DOI:10.15252/embr.201845969
- Vogan JM, Zhang X, Youmans DT, et al. Minimized human telomerase maintains telomeres and resolves endogenous roles of H/ACA proteins, TCAB1, and Cajal bodies. Elife. 2016;5. DOI:10.7554/eLife.18221
- Robart AR, Collins K. Investigation of human telomerase holoenzyme assembly, activity, and processivity using disease-linked subunit variants. J Biol Chem. 2010;285:4375–4386.
- Kim NK, Theimer CA, Mitchell JR, et al. Effect of pseudouridylation on the structure and activity of the catalytically essential P6.1 hairpin in human telomerase RNA. Nucleic Acids Res. 2010;38:6746–6756.
- Zemora G, Handl S, Waldsich C. Human telomerase reverse transcriptase binds to a pre-organized hTR in vivo exposing its template. Nucleic Acids Res. 2016;44:413–425.
- Davis DR. Stabilization of RNA stacking by pseudouridine. Nucleic Acids Res. 1995;23:5020–5026.
- Garcia CK, Wright WE, Shay JW. Human diseases of telomerase dysfunction: insights into tissue aging. Nucleic Acids Res. 2007;35:7406–7416.
- Vulliamy TJ, Kirwan MJ, Beswick R, et al. Differences in disease severity but similar telomere lengths in genetic subgroups of patients with telomerase and shelterin mutations. PLoS One. 2011;6:e24383.
- Yamaguchi H, Sakaguchi H, Yoshida K, et al. Clinical and genetic features of dyskeratosis congenita, cryptic dyskeratosis congenita, and Hoyeraal-Hreidarsson syndrome in Japan. Int J Hematol. 2015;102:544–552.
- Du HY, Pumbo E, Ivanovich J, et al. TERC and TERT gene mutations in patients with bone marrow failure and the significance of telomere length measurements. Blood. 2009;113:309–316.
- Carroll KA, Ly H. Functional characterization of mutations in the promoter proximal region of the telomerase hTERC gene identified in patients with hematological disorders. Int J Clin Exp Med. 2011;4:187–192.
- Trahan C, Dragon F. Dyskeratosis congenita mutations in the H/ACA domain of human telomerase RNA affect its assembly into a pre-RNP. RNA. 2009;15:235–243.
- Trahan C, Martel C, Dragon F. Effects of dyskeratosis congenita mutations in dyskerin, NHP2 and NOP10 on assembly of H/ACA pre-RNPs. Hum Mol Genet. 2010;19:825–836.
- Kropski JA, Reiss S, Markin C, et al. Rare genetic variants in PARN are associated with pulmonary fibrosis in families. Am J Respir Crit Care Med. 2017;196:1481–1484.
- Newton CA, Batra K, Torrealba J, et al. Telomere-related lung fibrosis is diagnostically heterogeneous but uniformly progressive. Eur Respir J. 2016;48:1710–1720.
- Moon DH, Segal M, Boyraz B, et al. Poly(A)-specific ribonuclease (PARN) mediates 3′-end maturation of the telomerase RNA component. Nat Genet. 2015;47:1482–1488.
- Bakar Ö, Işik U, Canpolat C, et al. Hoyeraal-hreidarsson syndrome: an extremely rare dyskeratosis congenita phenotype. Pediatr Dermatol. 2015;32:e263–e266.
- Kedde M, le Sage C, Duursma A, et al. Telomerase-independent regulation of ATR by human telomerase RNA. J Biol Chem. 2006;281:40503–40514.
- Cheng Y, Liu P, Zheng Q, et al. Mitochondrial trafficking and processing of telomerase RNA TERC. Cell Rep. 2018;24:2589–2595.
- Zheng Q, Liu P, Gao G, et al. Mitochondrion-processed TERC regulates senescence without affecting telomerase activities. Protein Cell. 2019;10:631–648.
- Rubtsova M, Naraykina Y, Vasilkova D, et al. Protein encoded in human telomerase RNA is involved in cell protective pathways. Nucleic Acids Res. 2018;46:8966–8977.
- Gazzaniga FS, Blackburn EH. An antiapoptotic role for telomerase RNA in human immune cells independent of telomere integrity or telomerase enzymatic activity. Blood. 2014;124:3675–3684.
- Theimer CA, Jády BE, Chim N, et al. Structural and functional characterization of human telomerase RNA processing and cajal body localization signals. Mol Cell. 2007;27:869–881.
- Fok WC, Shukla S, Vessoni AT, et al. Posttranscriptional modulation of TERC by PAPD5 inhibition rescues hematopoietic development in dyskeratosis congenita. Blood. 2019;133:1308–1312.
- Nagpal N, Wang J, Zeng J, et al. Small-molecule PAPD5 inhibitors restore telomerase activity in patient stem cells. Cell Stem Cell. 2020;26:896–909.e8.