Abstract
Organotin compounds are known to cause thymic atrophy and an accompanying deficiency of cell-mediated immunity. The study reported here focused on cell death in the thymus as a contributing factor in the induction of thymic atrophy following exposure to dibutyltin (DBTC) and tributyltin (TBTC). In an in vivo study, a reversible thymic atrophy was induced in rats by a single intraperitoneal administration (2.0 mg/kg) of DBTC or TBTC; the magnitude of this effect over a 4-d post-treatment period differed between the two agents. In in vitro studies, T-lymphocytes were isolated from thymuses of naïve rats and then exposed to 1 μM DBTC or TBTC for varying periods of time. Analysis by flow cytometry showed that DBTC induced primarily necrosis, while TBTC induced apoptosis, of the cells. Activities of caspase-8, -9, and -3 were also measured; TBTC exposure caused marked increases in the activities, while DBTC exposure did not cause any significant change. TBTC exposure also appeared to induce expression of CAD (which fragments DNA), but had minimal effect on levels of the CAD inhibitor, ICAD. In contrast, DBTC exposure resulted in a larger level of ICAD expression. WST-8 and JC-1 assays were used to evaluate mitochondrial function, since a strong activation of caspase-9 by TBTC suggested mitochondrial involvement. The involvement of caspase in the activation was examined using cytochrome c expression; cytochrome expression and the loss of mitochondrial function occurred within 10 min of TBTC exposure. DBTC exposure affected the mitochondria less. These results indicated that effects on mitochondria likely played an important role in the induction of apoptosis by TBTC. The results of this study show that DBTC and TBTC induce necrosis and apoptosis of T-lymphocytes, respectively, by apparently indicating different mechanisms of cell death. It follows that these increases in cell death induced by these organotin compounds likely contributed to the thymic atrophy observed in the rats here.
Introduction
Organotin compounds are used widely as a stabilizer of polyvinyl chloride, insecticides, herbicides, and antiseptics for fishing nets and paints on the bottoms of ships. Such widespread use of organotin compounds has caused serious environmental pollution (Piver, Citation1973; Fent, Citation1998). Past cases of organotin poisoning in humans were caused mostly by occupational exposure or by acute over-exposure to organotin-containing pharmaceutical products (such as in the Stalinon incident; FAO/WHO Monographs, Citation1971). Poisoning from organotin contamination in foodstuffs have not been as well documented, but have occurred. In light of these potential toxic events, the manufacture, importation, and use of tri-organotin compounds are presently prohibited in Japan. Nonetheless, marine pollution along the coast of Japan still continues from tri-organotins such as tributyltin and triphenyltin derived from extra-national sources. Thus, surveillance by national regulatory agencies continues in order to prevent the consumption of bioaccumulated organotin by humans (and other mammals) who routinely eat marine products.
The effects of organotin compounds on the endocrine (Ogata et al., Citation2001; Suzuki et al., Citation2002), immune, and nervous systems (Arakawa, Citation2000) in rats and/or mice are beginning to be clarified. Immunotoxicity caused by organotin is characterized by the selective induction of thymic atrophy and an accompanying deficiency in host cell-mediated immunity. In general, thymic atrophy depends on a marked depletion of lymphocytes; although the depletion is most often due to increases in cell death (Arakawa, Citation1983, Citation2000; Arakawa and Wada, Citation1988), other toxic manifestations (such as inhibition of lymphocyte maturation/proliferation, increases in cell efflux) cannot be dismissed out of hand. The alkyltins have the strongest immunosuppressive effect among organotin compounds, with dibutyltin causing more immunosuppression than tributyltin (Arakawa, Citation1995, Citation1997; Arakawa et al., 1998). During the course of a long-term (6-8 weeks) exposure regimen, dibutyltin induced an atrophy that was reversible, whereas the atrophy induced by tributyltin was irreversible (Murata et al., Citation1994; Suzuki et al., Citation2000). In contrast, following a single oral dose of either alklytin, induction of a reversible thymic atrophy was observed (Snoeij et al., Citation1988). Based on these findings, it seemed apparent that the mechanisms of thymic atrophy induced by exposure to the dialkyltin and trialkyltin were different.
The study here examined the potential mechanism(s) of cell death of thymic lymphocytes, an effect that almost assuredly contributes to the onset of thymic atrophy, induced by these two alkyltins. Of particular interest to study were the effects of each agent on lymphocyte mitochondria since damage at these organelles can ultimately lead to development of apoptosis. It has been known for ≈40 years that both di- and trialkyltins can cause mitochondrial respiratory dysfunction and inhibition of oxidative phosphorylation (Aldridge and Street, Citation1970; Selwyn et al., 1970; Penninks et al., Citation1983). Only more recently has it been hypothesized that this dysfunction could serve as a starting point in cell death induction, since mitochondria appear to play a key role in the regulation of apoptotic processes. Specifically, it has been shown that when there is a collapse of the mitochondrial membrane potential and an accompanying release of cytochrome c; (ie. when these both occur), there is an activation of cellular caspase-9 (Tsujimoto and Shimizu, Citation2000). In turn, caspase-9 eventually activates caspase-3 (Gabriel et al., Citation1998), which then leads to activation of a cytoplasmic DNA-fragmenting enzyme, CAD (caspase activity DNase) (Nagata et al., 1998), that partakes in the internucleosomal degradation of chromosomal DNA, a step that precedes cell death. Interestingly, these investigators also showed that an inhibitor (i.e., ICAD) of CAD was present in the cytoplasm and its function was to act as a counterbalance to this entire process.
In the study reported here, mechanisms of dibutyltin (DBTC)- and tributyltin (TBTC)- induced cell death in thymic lymphocytes (including, potentially, the mitochondrial-dependent apoptosis route described above) were investigated. In addition, any differences in the use of this/other mechanisms by each alkyltin were also assessed to potentially help explain why the two agents seem to differ in their effects on the thymuses of exposed hosts.
Materials and methods
Animals
Male Wistar rats (3-weeks-old, weighing ≈ 40 g,) were obtained from Japan SLC (Shizuoka, Japan) for use in these experiments. The animal studies here were conducted according to the principles in the Guidelines for the Care and Use of Laboratory Animals of the University of Shizuoka, under the supervision (and with the approval) of the Japanese Association of Laboratory Animal Facilities of Public and Private Universities (JALAP).
All rats were maintained individually under strict hygienic conditions in polycarbonate cages inside rooms having a controlled temperature (24 ± 1°C), humidity (55 ± 5%), and light-dark cycle (12 hr:12 hr). Food and water were provided ad libitum. All rats were acclimated for 1 week, and then randomly assigned (in groups of 5) to various treatment groups (for in vivo study; 25/regimen, with 5 per regimen to be analyzed at each indicated timepoint) or left ungrouped if they were to be used as the sources of thymic lymphocytes for all in vitro studies.
In vivo treatment with DBTC or TBTC
For the single intraperitoneal dosing (400 μl/rat), dibutyltin chloride (DBTC) or tributyltin chloride (TBTC) (Wako Pure Chemicals Co. Ltd., Tokyo, Japan) was dissolved in corn oil and delivered as 2.0 mg DBTC or TBTC/kg body weight. Rats were then euthanized 1, 2, 3, and 4 days thereafter. Each rat was weighed prior to removal of its thymus. The isolated organ was then weighed on an analytical balance, and the thymic index calculated.
In vitro studies
Isolation of thymic lymphocytes and treatment with organotin agents
An in vitro experimental system to investigate the effects of DBTC and TBTC was constructed using thymic lymphocytes isolated from naïve rats. The thymus was excised from each rat and its lymphocytes prepared by pressing the tissue through a stainless steel mesh. The liberated thymic lymphocytes were collected and washed three times with PBS (phosphate-buffered saline, pH 7.2) by centrifugation at 800 × g for 5 min each wash. The cells were then suspended in RPMI-1640 medium (Gibco-Invitrogen, Paisley, UK) at 8.0 × 106 cells/ml, and viability was measured by trypan blue staining (survival rate was ≥ 90%).
For all in vitro studies, aliquots of stock solutions of DBTC and TBTC (each at 20 μM) were adjusted (using absolute ethanol) to a concentration that would yield a final level of 1 μM for each in any given treatment well. The final ethanol concentration present over the course of these studies was always ≤ 0.1%, a level seen in preliminary studies to have no effect on cell viability (data not shown). Lengths of incubation of the lymphocytes with either alkyltin depended on the particular assay (timespans are indicated in respective sections below).
Analysis of apoptotic vs. necrotic cell death
The type of cell death that occurred among the cells treated for either 30 or 60 min was analyzed by double staining with fluorescein-labeled inhibitor of caspase-3 (FLICA; Immunochemistry Technologies, LLC, Bloomington, MN) and propidium iodide (PI). FLICA bound to the early apoptotic cells, and PI labeled the late apoptotic and necrotic cells with membrane damage. After exposure to either organotin compound, the T-lymphocytes were adjusted to a density of 1.0 × 106 cells/ml and washed in PBS. Staining was then performed according to manufacturer’s instructions. After labeling with FLICA, all samples were analyzed directly using a BD FACSCant flow cytometer (BD Biosciences Immunocytometry Systems, San Jose, CA) to determine the type of cell death. The percentages of necrotic (FLICA-/PI+) and of apoptotic (FLICA+/PI±) cells were determined using BD FACSDiVa software. Specifically, these values were calculated, respectively, as 100% × [the number of cells (events) in Quadrant 1 (Q1)]/sum total of all cells in Q1+Q2+Q3+Q4, and as 100% × [the number of cells (events) in Q2+Q4]/sum total of all cells.
Assay of activation of Caspase-3, Caspase-8, and Caspase-9
To assess the activities of the three caspases, T-lymphocytes (seeded at 8.0 × 106 cells/ml in culture flasks; total volume/flask = 25 ml) were treated with 1 μM DBTC or 1 μM TBTC, and then incubated at 37°C for 0 (untreated controls that received vehicle [i.e., medium containing ≤ 0.1% ethanol]), 10, 30, 60, or 120 min. In some cases, cells were pre-treated for 30 min with 50 μM caspase-8 inhibitor Z-IETD-FMK, caspase-9 inhibitor Z-LEHD-FMK, or caspase-3 inhibitor Z-DEVD-FMK (Santa Cruz Biotechnology, Inc., Santa Cruz, CA).
Following the treatments (± caspase inhibitors), the cytosolic fractions were isolated from the lymphocytes in a buffer containing 10 mM Tris-HCl (pH 7.4), 10 mM KCl, 2 mM MgCl2, 5 mM 2-mercaptoethanol, 1% Triton X-100, 10% glycerol, and 2% Protease Inhibitor Cocktail (Nacalai Tesque, Inc., Kyoto, Japan). The samples were placed on ice for 15 min and then cell membranes disrupted by homogenization. The nuclei and cell membranes were then removed by centrifugation at 800 × g for 5 min at 4°C. Total protein concentrations in each isolate were then determined using a BCA™ Protein assay kit with an albumin standard (Pierce, Rockford, IL).
The activation states of caspase-8, -9, and -3 in the samples were then evaluated by using caspase specific substrates. To perform each activity assay, an aliquot of cytosolic fraction (delivering equivalent of 5 μg) was placed in the well of a 96-well plate along with 50 μl reaction buffer (25 mM HEPES [pH 7.5], 10% sucrose, 0.1% CHAPS, and 10 mM dithiothreitol). One of the following caspase substrates (at 50 μl) was then added to each well: fluorometric caspase-8 substrate (IETD-AFC), caspase-9 substrate (LEHD-AFC), or caspase-3 substrate (DEVD-AFC). The plates were then incubated at 37°C for 2 hr and caspase activity then measured by assessing the levels of free AFC with a plate reader (Molecular Devices, Sunnyvale, CA) employing a 400 nm excitation filter and a 505 nm emission filter.
Mitochondrial function assay
The activity of mitochondrial dehydrogenase in the isolated T-lymphocytes was determined using a WST-8 assay kit (Dojindo Laboratories, Kumamoto, Japan), as described previously (Chen et al., Citation2006), and following manufacturer instructions. Briefly, the assay is based on cleavage of a tetrazolium salt to formazan dye by functioning cellular mitochondrial dehydrogenase. The amount of the dye generated is directly proportional to the number of living cells, and is directly related to the overall functionality of their mitochondria. In the study here, the lymphocytes were placed at 3.0 × 105 cells/well in 96-well plates and then cultured with 1 μM DBTC or TBTC at 37°C for 0 (untreated controls that received medium containing ≤ 0.1% ethanol), 10, 30, 60, 120, or 180 min. The absorbance in each well was then measured at 450 nm using a SPECTRAMAX 190 plate reader (Molecular Devices).
The reduction of mitochondrial membrane potential (Δψm) in the isolated T-lymphocytes was analyzed using JC-1 dye (Sigma-Aldrich, Inc., St. Louis, MO), according to the method of Reers et al (Citation1991). JC-1 was chosen for use here as this dye selectively enters the mitochondria and, depending on the membrane potential, forms J-aggregates that are associated with a large shift in emission spectra. In particular, a loss of mitochondrial membrane potential is shifted in fluorescence from red to green. In this study, the lymphocytes were plated at 1.0 × 106 cells/ml in culture flasks (total volume/flask = 25 ml) and then cultured with 1 μM DBTC or TBTC at 37°C for 0 (untreated controls that received medium containing ≤ 0.1% ethanol), 5 or 10 min. The cells were then resuspended in PBS and incubated with 10 μg JC-1 dye/ml at 37°C for 15 min. Both red and green fluorescence emissions were then analyzed during flow cytometry using an excitation wavelength of 488 nm and emission wavelength of 530 nm (for green fluorescence) or 585 nm (for red fluorescence).
Western blot analyses
Proteins related to apoptosis were examined using Western blot analyses of suitable subcellular fractions. Isolated lymphocytes were plated at 8.0 × 106 cells/ml in flasks (total volume/flask = 25 ml) and then cultured with 1 μM DBTC or TBTC at 37°C for 0 (untreated controls that received medium containing ≤ 0.1% ethanol), 10, 30, 60, or 120 min. The cells were then collected, washed with PBS, and cytosolic fractions isolated in a buffer containing 20 mM Tris (pH 7.5), 10 mM KCl, 1.5 mM MgCl2, 1 mM EDTA, 1 mM dithiothreitol, and 2% Protease Inhibitor Mixture (Nacalai Tesque, Inc.). The samples were then processed as described above. Cytosolic fractions without mitochondria were then extracted using a Mitochondria-Cytosol Fraction Kit (BioVision, Mountain View, CA), according to manufacturer’s instructions. Protein concentrations in the resultant extracts were assessed using the BCA™ assay kit (Pierce).
For the detection of cytochrome c in each sample, 10 μg of cytosolic fraction without mitochondria was separated over 15% SDS-PAGE gels. For detection of CAD (caspase activity DNase) and ICAD (inhibitor of CAD), 10 μg of cytosolic proteins was resolved over 12% SDS-PAGE gels. In all cases, electrophoresed proteins were then electrotransferred onto PVDF membranes (Amersham-Pharmacia Biotech, Arlington Heights, IL) that were then blocked with Block Ace (Dainippon Sumitomo Pharma Co., Ltd., Osaka, Japan). The membranes were then incubated with rabbit polyclonal anti-CAD (1:1000; Merck, Ltd), anti-ICAD (1:1000; Stressgen, San Diego, CA), anti-cytochrome c (1:500; Santa Cruz.), or anti-β-actin (1:5000; Sigma). After washing away unbound antibody and subsequent incubation with horseradish peroxidase-bearing anti-rabbit secondary antibody (Amersham) for 50 min at room temperature, all antibodies present were detected using an ECL signal enhancement kit (Amersham). Analysis of the β-actin was used to verify uniformity of protein loading and transfer. The fluorometric intensity of each immunoreactive band was ultimately estimated using Imaging Software (ImageQuant; Amersham Place, Little Chalfont, Buckinghamshire).
Statistics
All statistical analyses were performed using one-way analysis of variance (ANOVA) followed by post-hoc testing when appropriate using Fisher’s LSD or Kruskal–Wallis test followed by the Mann–Whitney U-test using SPSS 11.5J for Windows (SPSS Japan Inc., Tokyo). Differences were considered statistically significant at p-values < 0.05.
Results
Thymic atrophy after single alkyltin exposure
Over the 4-day period after the intraperitoneal (IP) administration of 2 mg/kg DBTC or TBTC, the thymus glands of rats at designated (daily) timepoints were excised and their relative weights/indices calculated (). The relative weights decreased the most between 24 and 48 hr after the single exposure. The thymic indices were decreased ≈ 20 and 10% (relative to control rat values) by 24 hr after IP DBTC and TBTC administrations, respectively; these values decreased to ≈40 and 30% of the time-matched control rats’ values by 48 hr, respectively (). These results showed that thymic atrophy in rats occurred as a result of the single IP administration of each organotin compound, and that the induced atrophy was greater with DBTC than TBTC. As had been seen in other studies using a single exposure to alkyltin agents (see Snoeij et al., Citation1988), the relative weight of the thymus began to improve after reaching a nadir (the timepoint defined by dose/route of exposure). In the study here, this reversal started to occur after the first 48 hr period post-exposure. Thus, with DBTC and TBTC, the period of peak thymic atrophy occurred at 24–48 hr after the single IP administration.
Table 1. Effect of organotin agents on thymus relative weight.
Figure 1. Thymic atrophy following organotin exposure. Relative thymus weight of rats given a single intraperitoneal injection of dibutyltin or tributyltin chloride at 2 mg/kg body weight. Treated and untreated (control) rat were euthanized at each post-exposure timepoint indicated and the thymus was removed and weighed. Results shown are the indices as percent of time-matched control rat values; mean ±SD (n=5). **p < 0.01 compared to control host values.
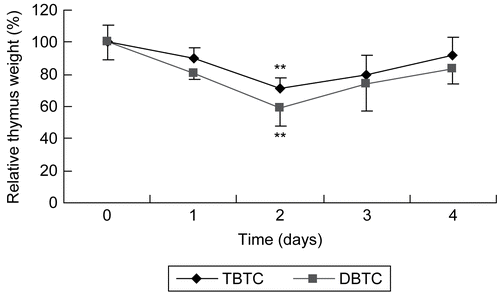
Flow cytometric analyses of cell death
The morphology of cell death caused in vitro by DBTC and TBTC was analyzed using flow cytometry with PI and FAM-DEVD-FMK, which binds covalently to active caspase-3 (Ekert et al., Citation1999). The vertical axis of each cytogram () represents PI expression and the horizontal axis that for active caspase-3. The region of PI (-) and caspase (-) is the area of viable cells (i.e., Q3), and the region of PI (+) and caspase (-) is the area of necrosis (i.e., Q1). The regions of PI (-) and caspase (+), and PI (+) and caspase (+) (i.e., Q4 and Q2, respectively) are the areas of apoptosis. When the percentages of viable, apoptotic, and necrotic cells were calculated (), the results showed that a significant amount (28%) of the cell populations “moved” into the region indicative of necrosis within 30 min of the start of the DBTC exposure; this value only increased a further 10.7% (to 31%) with an additional 30 min of incubation. Only ≈ 9.5% of the cells sorted to the regions indicative of apoptosis (PI [±] and caspase activity [+]); this value decreased slightly to ≈7% as the exposure continued for an additional 30 min. Concurrently, the numbers of viable cells were decreased to ≈63% of the total population examined.
Figure 2. Morphological analysis of cell death using flow cytometry. Five different cytograms of thymocytes were analyzed for viability, apoptosis, and necrosis. Thymocytes were treated with 1 μM DBTC or TBTC for the indicated times. FAM-VAD-FMK (X-axis; green fluorescence) and PI (Y-axis; red fluorescence) were then analyzed by flow cytometry. A: Control cells. B-1: Cells were exposed to DBTC for 30 min. B-2: DBTC for 60 min. C-1: TBTC for 30 min. C-2: TBTC for 60 min. Cell status was revealed by green vs. red fluorescence. Numbers shown indicate cell fractions gated in regions Q-1: necrosis, Q-2: late apoptotic cells, Q-3: live cells, and Q-4: early apoptotic cells. Relative amounts of viable, early apoptotic, late apoptotic, and necrotic thymocytes are shown. Both axes are in log scale. While the images shown are a representative cytogram from these assays, the percentage results shown in each are the mean ± SD (n=5 separate cell populations examined per treatment shown).
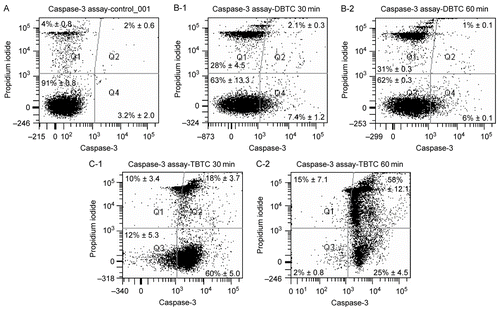
Table 2. Relative amounts of viable, early apoptotic, late apoptotic, and necrotic T-lymphocytes after treatments.
In contrast to the effects seen with DBTC, the overwhelming majority (78%) of the cell population “moved” into the caspase-3 active region (indicative of apoptosis) after just 30 min of TBTC exposure; this only increased by ≈6% (to 83%) with an additional 30 min of treatment. In this case, the extent of cells that appeared as necrotic was only 10% after 30 min of TBTC, but this value increased by half (to 15%) with a further 30 min of exposure. Unlike what was noted with the DBTC-treated cells, the number of viable cells was quite low (i.e., 12%) after just 30 min of TBTC exposure and fell all the way to 2% by the end of just the 1 hour of incubation with the alkyltin.
Measurement of caspase activity
Effects of the alkyltin agents on the activities of caspase-9 and -3 (which are involved with mitochondria) and of caspase-8 (which is independent of mitochondria) were examined. As shown in , weak activation of caspase-8 was observed in the DBTC-exposed lymphocytes. There appeared to be no significant effects on caspases-3 or -9 regardless of the length of treatment with DBTC. In contrast, strong activation of caspases-8 and -3 was observed in TBTC-exposed lymphocytes. In contrast, caspase-9 was activated, but the effect was short-lived (i.e., only occurred at the 30-min exposure point and not thereafter). With caspase-8, activation occurred within ≈10 min after TBTC exposure and was still being induced (albeit to a lesser degree) even after 120 min of treatment. With caspase-3, a similar consistent activation was induced starting ≈30 min after the start of the exposure.
Figure 3. Activation of caspases during alkyltin-induced cell death. Whole cell lysates (5.0 μg) were prepared from thymocytes exposed to 1 μM DBTC or TBTC for the indicated times. Activation of (A) caspase-8, (B) caspase-9, and (C) caspase-3 was determined using specific fluorescent substrates Ac-IETD-MCA, Ac-LEHD-MCA, and Ac-DMQD-MCA, respectively. Fluorescence was detected using a plate reader. Date are expressed as percentage of values found at 0 hr (control). Results shown are the mean ± SD (n=5 separate cell populations examined per treatment).
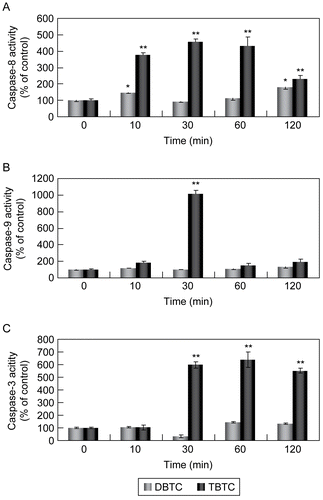
Analysis of mitochondrial function
The effect of exposure to TBTC or DBTC on thymic lymphocyte mitochondrial function was evaluated. The results here () indicated that activity of mitochondrial dehydrogenase 10 min after the start of the TBTC exposure was markedly reduced ≈70% compared to normal. Further incubation (i.e., out to 120 min) with this alkyltin resulted in a dehydrogenase activity level that was only further reduced to >80% of normal. In contrast, the effect of DBTC was generally less than that of TBTC. Though there was a progressive length of treatment-related decrease in the activity of the enzyme, the maximal decrease due to DBTC was only to a level of ≈70% of normal; however, of note, this change in activity was steep (as opposed to plateaued with the TBTC) over the period from 10–120 min of incubation.
Figure 4. Evaluation of time-dependent mitochondrial function. Mitochondrial dehydrogenase activity measured with WST-8 assay after T-lymphocyte exposure to 1 μM DBTC or TBTC for the indicated times. Absorbance was detected using a plate reader. Date are expressed as percentage of values found at 0 hr. Results shown are the mean ± SD (n=5 separate cell populations examined per treatment). Value statistically different (at **p < 0.01or *p < 0.05) compared to the control (0 min; vehicle-treated cells).
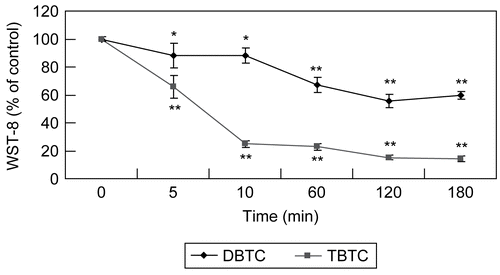
There appeared to be a strong relationship between the induced changes in the activity of mitochondrial dehydrogenase and changes in the ability of the treated lymphocytes to maintain membrane potential(s). As shown in representative flow cytograms (), the cell populations in the region designated “A” represented cells that had maintained normal potentials while those in the region “B” had a decrease in membrane potential(s). It was readily seen that there was a significant decrease in mitochondrial membrane potential in cells exposed to TBTC. While the initial drop was to a level of ≈30% of all the cells within the first 5 min, this reached a value of ≈60% in the period between 5 and 10 min after the start of the TBTC exposure. These changes appear to mirror the period in which the maximal drops in mitochondrial dehydrogenase activity occurred (see above). In contrast, there was little-to-no marked change in the membrane potential(s) after either period of DBTC exposure.
Figure 5. Analysis of mitochondrial membrane potential. Mitochondrial membrane potential was analyzed using a flow cytometry with JC-1. Red fluorescence intensity indicated cells with stable Δψm, while green fluorescence intensity indicated cells with low Δψm. A shift from red to green denotes dissipation of potential. The cell populations in region designated “A” represented cells that had maintained normal potentials; those in the region designated “B” had a decrease in membrane potential(s).The percentage of mitochondrial membrane potential depolarized cells in region (B) is indicated. While the images shown are a representative cytogram from these assays, the percentage results shown in each are the mean ± SD (n=5 separate cell populations examined per treatment shown).
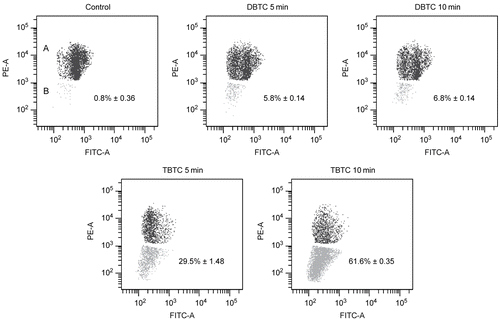
Expression of apoptosis-inducing factors
Western blotting was used to analyze the expression of ICAD, CAD, and cytochrome c, proteins that have each been shown to be associated with apoptosis. As shown in , there was a marked increase in expression of cytochrome c between 10 and 60 min after the start of the TBTC exposure. When the fluorescent intensities of the bands were analyzed, the expression level at 10–30 min was four times that of control (t = 0 min) levels. In contrast, the expression of cytochrome c was not as markedly affected after DBTC exposure.
Figure 6. Western blot analyses of time-dependent changes in cytochrome c. Cytosolic fractions without mitochondria (10 μg/ml) from organotin-exposed T-lymphocytes were separated over an 15% SDS-PAGE gel, and then transferred to PVDF membranes. The membranes were immunoblotted with rabbit polyclonal anti-cytochrome c (1:500), and the signals were detected by use of an ECL system. β-Actin was employed as an internal standard to verify uniformity of protein loading and transfer. (A) Representative immunoblot; each band indicates cytochrome c protein and β-actin protein. (B) Band intensity as determined using Image QuaNT. Results shown are the mean ± SD (n=5 separate cell populations examined per treatment shown).
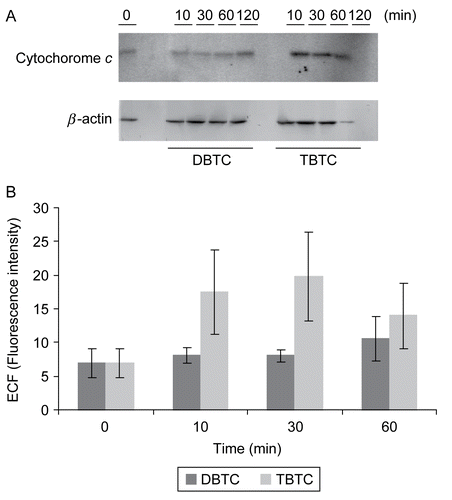
Recalling (see ) that the strongest activation of caspase-3 was found among the thymic lymphocytes treated with TBTC for 60 min, and that ICAD is cleaved (and activated) by caspase-3, expression of CAD and ICAD were compared in samples obtained after a 60 min exposure to the test agents. Expression of CAD did not appear to largely differ among the controls, DBTC-exposed, and TBTC-exposed lymphocytes (). On the other hand, while ICAD was expressed to approximately the same extent in the controls and DBTC-exposed cells, levels of ICAD in the TBTC-exposed lymphocytes were decreased to one-third of those in the controls and DBTC-exposed cells.
Figure 7. Expression of CAD and ICAD. Cytosolic fractions (10 μg/ml) from organotin-exposed T-lymphocytes were resolved over a 12% SDS-PAGE gel, then transferred to PVDF membranes. The membranes were then immunoblotted with rabbit polyclonal anti-CAD (1:1000) and anti-ICAD (1:1000), and the signals later detected by use of an ECL system. β-Actin was employed as an internal standard to verify uniformity of protein loading and transfer. (A) Representative immunoblot; each band indicates CAD protein and ICAD protein, respectively. (B) Intensity of each immunoreactive band was estimated using fluorometric quantification. Results shown are the mean ± SD (n=5 separate cell populations examined per treatment shown).
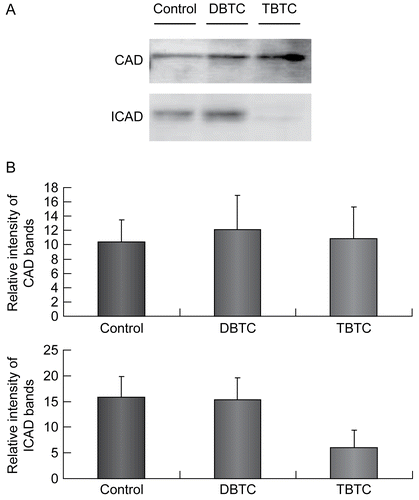
Effects of caspase inhibitor
To clarify whether any TBTC-induced caspase-9 activation, in turn, caused caspase-3 activation, caspase-3 activity was measured after a 60-min TBTC exposure of cells that did or did not receive a pre-treatment with caspase-9 inhibitor Z-LEHD-FMK. At this timepoint, caspase-3 activity was ≈ 6-times greater than that in the control cultures (). However, when caspase-9 activity was inhibited, caspase-3 activity was suppressed to control levels. Interestingly, a similar effect was observed when the caspase-8 inhibitor Z-IETD-FMK was employed. With respect to caspase-8 activation by TBTC, the results indicated that caspase-8 activity (after 60 min of cell exposure to TBTC) was ≈ 4.5-times that of the controls. However, this activity was almost completely inhibited in cells in which caspase-3 activity had been inhibited (). In contrast, only one-third of the TBTC-stimulated activity of caspase-8 was inhibited when the cells had been pre-treated with Z-LEHD-FMK. As previously seen in , treatments with the DBTC alone once again had only nominal effects on the activities of either caspase-3 or -8.
Figure 8. Effect of caspase-8, -9, and/or -3 inhibitors on alkyltin-induced activation of caspases-3 and -8. Cells were pre-treated with vehicle, 50 μM Z-DEVD-FMK (caspase-3 inhibitor), Z-IETD-FMK (caspase-8 inhibitor), or Z-VAD-FMK (caspase-9 inhibitor) 30 min prior to the challenge with 1 μM TBTC. One hour after the treatment with TBTC (or DBTC for comparative purposes) was begun, (A) caspase-3 and (B) caspase-8 activities were determined using a plate reader. Results shown are the activities as percent of time-matched control values; mean ±SD (n=5 separate cell populations examined per treatment shown). Value statistically different at **p < 0.01 compared to control and/or at ##p < 0.01 compared to TBTC- only treated cells.
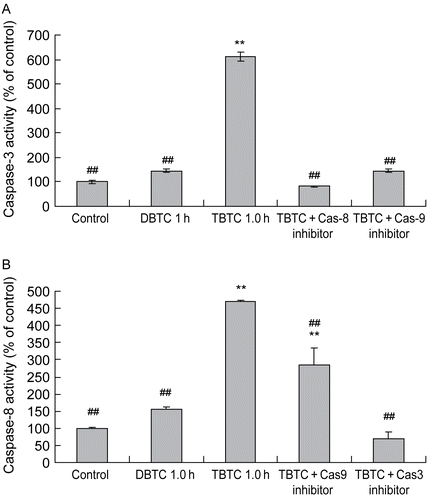
Discussion
A self-reversible thymic atrophy was induced by a single intraperitoneal administration of the organotins DBTC or TBTC. In this study, these alkyltins were believed to distribute to the thymus with minimal metabolism because of the nature of how they were administered (Arakawa, Citation1983, Citation2000; Arakawa and Wada, Citation1988). Oddly, though the extent of atrophy induced by either agent was at its maximum 48 hr after administration to the hosts, the magnitude of atrophy was greater in rats administered DBTC than TBTC. This was somewhat odd in that the concentration of organotin in the thymus was on the order of 10−6 M for both compounds. Because the thymic atrophy induced was likely dependent on the local tin concentration, it would not be unexpected that each agent was being eliminated from the thymus over the course of the 48 hr period and then reached a level that made it possible for its lymphocytes to again increase in number (and the weight of thymus to rise, accordingly).
Because of these differences in atrophy induction, in vitro studies were performed to determine if there were alkyltin compound-related differences in mechanisms that could lead to thymic lymphocyte death, one likely significant factor contributing to the thymic atrophy in the exposed hosts. The results of one of the assays here indicated that while there was caspase-3 activity (indicative of apoptosis) in nearly 80% of the cells after 30–60 min of TBTC exposure. In contrast, caspase activation/apoptotic events were minimal with DBTC exposure and, instead, necrosis had occurred in nearly 30% of all cells. These “patterns” of cell death seen with the in vitro DBTC and TBTC exposures are consistent with the results of histopathologic analyses described by Arakawa (Citation2003) using alkyltin-exposed rodents.
While we believe that these differences in how DBTC and TBTC appear to induce the death of thymic lymphocytes in vitro might underlie their differential effects on induction of thymic atrophy in vivo, we are keenly aware that tin-induced cell death is only a single means by which that pathology could have arisen in vivo. For example, in general, even a low concentration of organotin can cause inhibition of cell growth; thus, this toxic outcome could also have been a likely contributor to the onset of thymic atrophy in our rats (as it was in other studies that examined alkyltin-exposed hosts; Snoeij et al., Citation1988; Pieters et al., Citation1992; Gennari et al., Citation1997). Clearly, a combined alykyltin-induced inhibition of cell growth and simultaneous depletion of lymphocytes due to enhanced cell death could be sufficient to give rise to thymic atrophy in vivo.
Accordingly, differences in how DBTC and TBTC impact on BOTH of these parameters could explain our in vivo observations here. Further, there are other toxic manifestations (for example, an increase in cell efflux from the thymus) that could have been induced by either/both alkyltins, and cannot yet be dismissed out of hand. Ongoing investigations in our laboratory will help to determine if/the extent to which these endpoints (i.e., cell proliferation, cell efflux) are affected using the experimental conditions employed in the studies here.
The results of our in vitro studies here indicated that the following were involved in the mechanism of apoptosis induction by TBTC: a reduction of mitochondrial function and membrane potential; the release of cytochrome c into the cytoplasm; activation of caspases; and, activation of CAD due to an ICAD decrease (as originally hypothesized in Tsujimoto and Shimizu, Citation2000). In addition, selective activation of caspase-8 (as predicted based on studies performed by Nagata [Citation1997] as well as Ashkenazi and Dixit [Citation1998]) was observed. Interestingly, when caspase-3 activity was inhibited, this caspase-8 activation was inhibited. This outcome supports the claims by others (i.e., Zou et al., Citation2003) that caspase-3 was thought to activate not only endonucleases, but also intracellular caspases that were in an inactive state.
In addition, it was noted here that when caspase-9 activation was suppressed, the alkyltin (specifically, TBTC)-triggered caspase-8 activation was somewhat suppressed. Because both caspase-9 and cytochrome c have been reported to activate caspase-8 (Tang et al., Citation2000; McDonnell et al., Citation2003), these results reflect the fact that at least some of the TBTC-induced caspase-8 activity must have evolved indirectly from a parallel activation of caspase-9. These activations of inactive caspases by TBTC likely enhanced a death signal response - leading to the execution of apoptotic processes. On the other hand, any caspase-dependent apoptosis response appeared to be weak for DBTC.
One reason for these variations in ability to induce caspases/apoptotic pathways in the lymphocytes may be due to the difference in the intracellular point of action between DBTC and TBTC (i.e., differences in reactivities at the site of toxic action/effect due to inherent differences in each alkyltin’s chemical structure/properties). These differences in properties have been suggested to give rise to critical variations in the intracellular distribution of each agent (Arakawa et al., Citation1991; Iizuka et al., Citation1991; Iizuka and Arakawa, Citation1992a, Citation1992b; Arakawa, Citation2000). Several organotin compounds are known to cause inhibition of ATP synthesis and inhibition of oxidative phosphorylation in mitochondria (Aldridge and Street, Citation1970; Selwyn et al., 1970; Dawson and Selwyn, Citation1975; Cain et al., Citation1977; Penninks et al., Citation1983).
With respect to potential effects on cellular membranes by the agents analyzed here, DBTC does not directly affect the membrane but instead rapidly passes through; in contrast, TBTC directly affects membranes and destroys their structure and function (White and Tobin, Citation2004). In fact, certain physicochemical properties of TBTC are strikingly different from that of DBTC. For example, the logarithms of the n-octanol-water partition coefficients (log P) for DBTC and TBTC are 0.05 and 2.2, respectively (Tsuda et al., Citation1988); this indicates that TBTC is more lipophilic than DBTC. These differences in effect on phospholipid bilayers could be one explanation for the different predominant mechanism of cell death induction apparently being employed by these two alkyltin agents in this current study.
These studies have shown that, in vitro, there are significant differences in the predominant mechanism of thymic lymphocyte death induced by DBTC and TBTC. DBTC exposure caused a necrosis that was not dependent on the activation of caspase(s). In contrast, TBTC exposure primarily resulted in induction of apoptosis of the lymphocytes. These TBTC-induced outcomes appeared to be mediated, in part, through effects on the cells’ mitochondria and induction of caspases. In general, this TBTC-triggered apoptosis seemed to evolve through a series of reactions, i.e., a decrease in the membrane potential, followed by a release of cytochrome c, and, ultimately, an activation of caspase(s). Our ongoing studies will build upon results here to ascertain if the alkyltin-specific preferred “in vitro’ mechanisms of toxicity by DBTC and TBTC among thymic lymphocytes are also the bases for their apparent differential toxicities (re: thymic atrophy) in vivo.
Acknowledgements
Declaration of interest: The authors report no conflicts of interest. The authors alone are responsible for the content and writing of the paper.
References
- Aldridge, W. N., and Street, B. W. 1970. The specific binding of trimethyltin and triethyltin to rat liver mitochondria. Biochem. J. 118:171–179.
- Arakawa, Y. 1983. Organotin compounds and lymphocytes. J. Pharmacol. Dyn. 6:S23.
- Arakawa, Y. 1995. Tin and Immunity—Review. Biol. Res. Trace Elem. 6:1–34.
- Arakawa, Y. 1997. Recent studies on the model of biological action of di- and trialkyltin compounds. Chem. Tin 10:388–422.
- Arakawa, Y. 2000. Invasion of biofunctions by organotins. Biol. Res. Trace Elem. 11:259–286.
- Arakawa, Y. 2003. Morbid aging of thymus immunity by metals. Biol. Res. Trace Elem. 14:249–258.
- Arakawa, Y., and Wada, O. 1988. Suppression of cell proliferation by certain organotin compounds. In: Tin and Malignant Cell Growth, Chapter 9. Zuckerman J.J. (Ed.), CRC Press, Boca Raton, Florida, USA, pp. 83–106.
- Arakawa, Y., Iizuka, T., and Matsumoto, C. 1991. Intracellular distribution of organotin compounds and its effects on the structure and function of organellae. Biol. Res. Trace Elem. 2:321–326.
- Ashkenazi, A., and Dixit, V. M. 1998. Death receptors: Signalling and modulation. Science 281:1305–1308.
- Cain, K., Hymas, R. L., and Griffiths, D. E. 1977. Studies on energy-linked reactions: Inhibiton of oxidative phosphorylation and energy-linked reactions by dibutyltin dichloride. FEBS Lett. 82:23–28.
- Chen, C. L., Lin, C. F., Chiang, C. W., Jan, M. S., and Lin, Y. 2006. Lithium inhibits ceramide- and etoposide-induced protein phosphatase 2A methylation, Bcl-2 dephosphorylation, caspase-2 activation, and apoptosis. Am. Soc. Pharmacol. Exp. Ther. 70:510–517.
- Dawson, A. P., and Selwyn, M. J. 1975. The action of tributyltin on energy coupling in coupling-factor-deficient sub-mitochondrial particles. Biochem. J. 152:333–339.
- Ekert, P. G., Silke, J., and Vaux, D. L. 1999. Caspase inhibitors. Cell Death Differ. 6:1081–1086.
- FAO/WHO Monographs, 1971. Evaluations of Some Pesticide Residues in Food. Rome: Food Agricultural Organization, UN/WHO, 327.
- Fent, K. 1998. Effect of organotin compounds in fish: From the molecular to the population level. Fish Ecotoxicol. 86:259–302.
- Gabriel, N., Benedict, M. A., Hu. Y., and Inohara, N. 1998. Caspases: The proteases of the apoptotic pathway. Oncogene 17:3237–3245.
- Gennari, A., Potters, M., Seinen, W., and Pieters, R. 1997. Organotin-induced apoptosis as observed in vitro is not relevant for induction of thymus atrophy at anti-proliferative doses. Toxicol. Appl. Pharmacol. 147:259–266.
- Iizuka, T., and Arakawa, Y. 1992a. Effect of organotin compounds on the structure and function of endoplasmic reticulum. Japan J. Hyg. 47:249.
- Iizuka, T., and Arakawa, Y. 1992b. Effect of organotin compounds on the structure and function of Golgi apparatus. Japan. J. Hyg. 46:302.
- Iizuka, T., Matsumoto, C., and Arakawa, Y. 1991. Inhibition of calcium mobilization by certain organotin compounds. Japan J. Hyg. 46:303.
- McDonnell, M. A., Wang, D., Khan, S. M., Vander Heiden, M.G., and Kelekar, A. 2003. Caspase-9 is activated in a cytochrome c-independent manner early during TNFalpha-induced apoptosis in murine cells. Cell Death Differ. 10:1005–1015.
- Murata, J., Sano, Y., Arakawa, Y., Nakanishi, H., Hori, S., and Wada, O. 1994. Organotin-induced thymus atrophy and its tolerance manifestation. Japan. J. Hyg. 49:140.
- Nagata, S. 1997. Apoptosis by death factor. Cell 88:355–365.
- Ogata, R., Omura, M., Shimasaki, Y., Kubo, K., Oshima, Y., Aou, S., and Inoue, N. 2001. Two-generation reproductive toxicity study of tributyltin chloride. J. Toxicol. Environ. Health 63:127–144.
- Penninks, A. H., Verschuren, P. M., and Seinen, W. 1983. Di-n- butyltin-dichloride uncouples oxidative phosphorylation in rat liver mitochondria. Toxicol. Appl. Pharmacol. 70:115–120.
- Pieters, R. H., Bol, M., Lam, B. W., Seinen, W., and Penninks, A. H. 1992. The organotin-induced thymus atrophy, characterized by depletion of CD4+CD8+ thymocytes, is preceded by a reduction of the immature CD4−CD8+ TcRαβlow CD2high thymoblast subset. Immunology 76:203–208.
- Piver, W. T. 1973. Organotin compounds: Industrial application and biological investigations. Environ. Health Perspect. 4:61–79.
- Reers, M., Smith, T. W., and Chen, L. B. 1991. J-Aggregate formation of a carbocyanine as a quantitative fluorescent indicator of membrane potential. Biochemistry 30:4480–4486.
- Sakahira, H., Enari, M., and Nagata, S. 1998. Cleavage of CAD inhibitor in CAD activation and DNA degradation during apoptosis. Nature 391:96–99.
- Snoeij, N. J., Penninks, A. H., and Seinen, W. 1988. Dibutyltin and tributyltin compounds induce thymus atrophy in rats due to a selective action on thymic lymphoblasts. Int. J. Immunopharmacol. 10:891–899.
- Stockdale, M., Dawson, A. P., and Selwyn, M. J. 1970. Effects of trialkytin and triphenyltin compounds on mitochondrial respiration. Eur. J. Biochem. 15:342–351.
- Suzuki, H., Murayama, N., Yamaguchi, C., Ono, C., Ohba, R., Kuroda, M. Ohtani, Y., Nakano, Y., Takeuchi. T., and Arakawa, Y. 2002. Calcium overload and apoptosis in the thymus induced by tributyltin exposure. KURRI Progress Report 2001, p. 136.
- Suzuki, H., Otani, Y., Ono, C., Murayama, N., Yamaguchi, C., Kuroda, M., Oba, R., and Arakawa, Y. 2002. The reproductive toxicity of organotin on the mammalian species. Trace Nutr. Res. 19:35–38.
- Suzuki, H., Otomo, Y., Arakawa, Y., Takeuchi, T., Nakano, Y., and Nakashima, H. 2000. Manifestation of tolerance and cell death suppressive factors in the organotin-induced thymus atrophy. Biol. Res. Trace Elem. 11:423–424.
- Tang, D., Lahti, J. M., and Kidd, V. J. 2000. Caspase-8 activation and bid cleavage contribute to MCF7 cellular execution in a caspase-3-dependent manner during staurosporine-mediated apoptosis. J. Biol. Chem. 275:9303–9307.
- Tsujimoto, Y., and Shimizu, S. 2000. Bcl-2 family: Life-or-death switch. FEBS Lett. 466:6–10.
- Tsuda, T., Nakanishi., H, Aoki., S., and Takebayashi, J. 1988. Bioconcentration and metabolism of butyltin compounds in carp. Water Res. 22:647–651.
- White. J. S., and Tobin, J. M. 2004. Inorganic tin-organotin interactions with Candida maltosa. Appl. Microbiol. Biotechnol. 63:445–451.
- Zou, H., Yang, R., Hao, J., Wang, J., Sun, C., Fesik, S. W., Wu, J. C., Tomaselli, K. J., and Armstrong, R. C. 2003. Regulation of the Apaf-1/caspase-9 apoptosome by caspase-3 and XIAP. J. Biochem. 278:8091–8098.