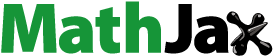
Abstract
Dissolved organic carbon (DOC) plays an important role in the carbon cycle, radiative forcing, and biogeochemistry in cryospheric regions. In this study, concentrations, light-absorption properties and bioavailability of DOC from snow cover in Alaska were characterized. Results indicated that average concentrations of DOC in snow of Alaska (0.17 − 0.30 mg L−1) were lower than that found in Asian mountainous glaciers, but higher that found from polar regions snow. No significant trend of DOC was observed during April to May in 2017 due to the short term study; while the vertical variations generally showed a little higher values in the surface snow than that in the sub-surface snow. An obvious characteristic of DOC light-absorbance in snow between the wavelength of 300 and 700 nm indicated the mass absorption cross section of DOC at 365 nm (MACDOC365) was 0.32 ± 0.24 and 0.37 ± 0.32 m2 g−1 for the snow cover at Barrow site (Arctic Ocean coast) and the other Alaskan regions, respectively. The MACDOC365 values increased especially during snow melting, indicating the DOC with high MAC values were prone to retain in snow. The proportion of radiative forcing caused by DOC relative to that by black carbon in snow was approximately 2.3%, indicating that DOC in snow should be considered during the accelerated melt of snow cover. The bioavailability experiment of DOC in snow indicated that DOC may be an important bioavailable source for proglacial and coastal ecosystems in Alaskan Arctic regions. Using backward air mass trajectory analysis, we suggested that DOC deposited in snow at Barrow primarily originates from marine or terrestrial air mass, but the specific contribution of different sources cannot be quantified without data related to the DOC’s chemical composition and carbon isotopic signatures. This study highlighted the climatic implications of DOC in snow in the Arctic regions.
1. Introduction
The effect of climatic and cryospheric change on carbon cycling is of great research interest due to carbon potential feedback on temperature and ecosystems (Hood et al., Citation2009; Singer et al., Citation2012; Lawson et al., Citation2014; Hood et al., Citation2015; Musilova et al., Citation2017). The reservoir of dissolved organic carbon (DOC) in snow and ice is small (4.48 ± 2.79 PgC) in comparison to that stored in gross primary productivity and respiration. However, it is important because DOC in snow and ice is much more bioavailable compared to the net changes in terrestrial carbon storage (Ping et al., Citation2008; Anesio et al., Citation2009; Legrand et al., Citation2013; Fellman et al., Citation2015; Hood et al., Citation2015; Liu et al., Citation2016; Yan et al., Citation2016), which may impact the carbon cycle of downstream ecosystems (Hood et al., Citation2009; Spencer et al., Citation2014). Snow and ice constitute an important link between the terrestrial and aquatic carbon flux due to snow meltwater transportation and is of increasing importance in land-to-ocean fluxes of organic carbon in cryospheric regions (Antony et al., Citation2014; Lawson et al., Citation2014; D’Amore et al., Citation2015; Fellman et al., Citation2015; Hood et al., Citation2015; Huntington et al., Citation2016; Gao et al., Citation2019, Citation2020).
Snow cover, a critically important component the Arctic, is greatly affected by climate change (Callaghan et al., Citation2011; Bokhorst et al., Citation2016). The duration of the presence of the Arctic snow cover is decreasing rapidly (about 3–5 days per decade) as a result of a delay in the onset of snow cover and the earlier onset of the spring melt (Derksen et al., Citation2015). This, in turn, affects Arctic sea ice, ecosystems, and societies (Goldenson et al., Citation2012; Cooper, Citation2014). In addition to a warmer Arctic climate (Callaghan et al., Citation2011; Pithan & Mauritsen, Citation2014; Huang et al., Citation2017), the presence of light-absorbing organic carbon and black carbon in snow plays a pivotal role in accelerating snow aging and the speed of the snowmelt (Warren & Wiscombe, Citation1980; Flanner et al., Citation2007; Doherty et al., Citation2010; Dumont et al., Citation2014).
Many studies have shown that DOC concentrations in snow of the polar regions varied considerably across the snowmelt season, both temporally and spatially (Antony et al., Citation2011; Legrand et al., Citation2013; Antony et al., Citation2014; Fellman et al., Citation2015), with a range of 0.01 to 43.2 mg L−1 (Hood et al., Citation2015). The highest DOC concentrations were found in basal ice and cryoconite holes, reflecting localized accumulation of organic matter from overrun organic carbon (OC) (Stibal et al., Citation2012) and in-situ production (Stibal et al., Citation2010; Telling et al., Citation2012), respectively. This variability may be the result of the different sources of glacier-derived DOC as mentioned in previous studies (Kim et al., Citation2005; Hood et al., Citation2009; Singer et al., Citation2012; Stubbins et al., Citation2012; Spencer et al., Citation2014, Citation2016), including deposition of aerosols containing soil organic matter and fossil fuel combustion byproducts.
A fraction of DOC known as water-soluble brown carbon is considered to be light-absorber, akin to coloured dissolved organic matter (CDOM) in the atmosphere. Water-soluble CDOM exhibits strong light-absorbing properties in the ultraviolet wavelength (Andreae & Gelencser, Citation2006; Chen & Bond, Citation2010; Yan et al., Citation2016). The radiative forcing (RF) attributed to DOC relative to black carbon (BC) in aerosols has been estimated at 2–10% in a typical polluted area in North China (Kirillova et al., Citation2014), and approximately 1% in a remote island in the Indian Ocean (Bosch et al., Citation2014). Using a model simulation, Lin et al. (Citation2014) reported that the RF caused by organic carbon deposited on terrestrial snow and sea ice ranged from +0.0011 to +0.0031 W m−2.
Previous studies have shown that the organic carbon in snow contributes to a decreased albedo and has a high bioavailability (Lin et al., Citation2014; Fellman et al., Citation2015). However, data published is still sparse. Here, we focus on documenting the properties of DOC in snow cover at Barrow and across the Alaska, which will enhance our understanding on its characteristics, bioavailability, light-absorbing properties, and potential impact on snowmelt. This study will provide insights into the DOC in the Arctic snow.
2. Methodology
2.1. Study area and sampling
From April 6, 2017 to May 18, 2017, surface snow samples were collected from snow cover on sea ice in the Chukchi Sea, about 12 km northeast of Barrow, Alaska (Fig. S1), and stored in pre-cleaned polycarbonate bottles. Vertical profile samples throughout the snowpack depth were collected at a typical resolution of 3–5 cm, and seven snowpack profiles were collected on the Chukchi sea ice and two at the Barrow Environmental Observatory (BEO) site, for comparison. To describe the spatial variation in DOC in the Alaskan snow cover, 27 surface snow samples were also collected across central Alaska between April 19 and 23, 2017 (Fig. S1). Detailed information is provided in the Supplementary Table S1 and Text S1.
2.2. Laboratory analyses
2.2.1. Measurements of DOC, BC, and major ions
DOC concentrations were determined using a total organic carbon (TOC) analyzer (Shimadzu Corp, Kyoto, Japan), after filtering samples through a 0.45 μm pore-sized polytetrafluoroethylene membrane filter. The detection limit of the TOC analyzer was 15 μg L−1. The TOC analyzer can also measure total nitrogen content (TN) with a standard deviation of less than 3%.
BC concentrations in the snow were measured using a single particle soot photometer (SP2, Droplet Measurement Technology, Boulder, USA), with the detection limit of 0.3 fg/particle. The major cations (Ca2+, Mg2+, Na+, K+, NH4+) and anions (Cl–, SO42–, NO3–) were measured using the Dionex-600 and the Dionex-2500 Ion Chromatograph Systems (Dionex, USA), respectively. The detection limit was 1 µg L−1 and the precision errors was better than 5%. Detailed information is provided in Supplementary Text S1. Blanks of DOC and TN measurement in this study is listed in Table S2.
2.2.2. Light absorption measurements
The light-absorbing properties of DOC were analyzed using the SpectraMax M5, an ultraviolet/visible absorption spectrophotometer that measures wavelengths between 200–800 nm with a resolution of 5 nm. During sampling, pure water of MilliQ is used as the blank sample. The mass absorption cross section of DOC (MACDOC365) was calculated using the Lambert-Beer law (Bosch et al., Citation2014; Kirillova et al., Citation2014; Yan et al., Citation2016):
(1)
(1)
where I0 and Ii represent the light intensities of the transmitted light and incident light, respectively; A are the absorbances derived directly from the spectrophotometer at a wavelength of 365 nm; (C) stands for the concentration of DOC (mg L−1); and L is the absorbing path length (0.01 m).
The absorption Ångström exponent (AAE) was calculated using the equation (EquationEq. 2(2)
(2) ) (Kirillova et al., Citation2014):
(2)
(2)
where λ (nm) represents the wavelength; and A(λ) can be derived directly from the spectrophotometer at λ nm. Measurements of AAE can be obtained at wavelengths (λ) between 330 to 400 nm (Chen & Bond, Citation2010; Kirillova et al., Citation2014).
Studies show that BC in snow produces surface warming in the Arctic, further accelerating the snow and ice melting process (Flanner et al., Citation2007; Dou et al., Citation2012; Bond et al., Citation2013; Lin et al., Citation2014; Qian et al., Citation2015). Using a simplistic model (EquationEq. 3(3)
(3) ), we estimated the RF caused by DOC relative to BC in snow. The fraction (f) can be calculated as:
(3)
(3)
where λ (nm) stands for the wavelength; I (λ) is the clear sky Air Mass 1 Global Horizontal (AM1GH) irradiance spectrum on the Earth’s surface, which includes light-absorptive effects of the ozone and water, among others (Levinson et al., Citation2010); MACDOC365 and MACBC550 represent MAC of DOC at 365 nm and MAC of BC at 550 nm, respectively; hABL is the vertical height of the atmospheric boundary layer; and AAEDOC and AAEBC are the AAE of DOC and BC, respectively. In this model, MACBC550 = 7.5 ± 1.2 m2 g−1 (Bond and Bergstrom, Citation2006), AAEBC is set to be 1 (Bond and Bergstrom, Citation2006; Doherty et al., Citation2010; Kirillova et al., Citation2014), and hABL is set at 1000 m (Bosch et al., Citation2014; Kirillova et al., Citation2014).
Specific ultraviolet absorbance (SUVA254 with unit of L mg C−1 m−1) was defined by dividing the decadal ultraviolet-visible absorption coefficient per meter at the wavelength (λ) of 254 nm by the DOC concentration. SUVA254 can reflect the general absorptivity at λ = 254 nm for DOC, which typically represents an index of DOC aromaticity (Weishaar et al., Citation2003; O’Donnell et al., Citation2016).
2.3. In-situ DOC bioavailability experiment
The DOC bioavailability (BDOC) experiment was conducted during the fieldwork between May 7 and 17, 2017, at the sea ice surface near Barrow. In brief, surface snow samples were collected in pre-combusted (550° C for 6 h) aluminum basins. The samples were melted in the field and filtered through pre-combusted glass fiber filters (GF/F 0.7 µm) into 12 pre-cleaned 60 mL polycarbonate bottles that were wrapped in three layers of aluminum foil to avoid solar irradiation. Two samples were refrigerated immediately after filtering to obtain initial DOC concentrations and the others were placed outside at the sampling site A.
2.4. Principal component analysis and backward air mass trajectory analysis
Principal component analysis (PCA) is a statistical procedure that uses an orthogonal transformation to convert a set of observations of possibly correlated variables into a set of values of linearly uncorrelated variables called principal components. The resulting vectors are an uncorrelated orthogonal basis set. Zhang et al. (Citation2016) used PCA in discussions of snow chemistry.
Back trajectories were calculated using the Hybrid Single Particle Lagrangian Integrated Trajectory (HYSPLIT) model (Draxler and Rolph, Citation2010), for the days with recorded precipitation, to determine source regions for air masses arriving in Barrow. Additionally, footprint analyses were also performed using the European Centre for Medium-Range Weather Forecasts (ECMWF) analysis fields, with the Lagrangian analysis tool LAGRANTO (Wernli and Davies, Citation1997; Sprenger and Wernli, Citation2015) launched every six hours during the study period.
3. Results and discussion
3.1. DOC, TN, BC, and concentrations of major ions
3.1.1. Spatial distribution
The spatial distribution of DOC, TN, BC, and major ions is shown in . We found lower concentrations of DOC in Barrow (∼ 0.17 mg L−1) and southern Alaska (near Denali National Park and Preserve) (∼0.24 mg L−1), and higher values occurring in middle Alaska (near Fairbanks) (∼0.33 mg L−1). In terms of TN, higher values were observed in northern Alaska (near Prudhoe Bay) (∼0.15 mg L−1), near the coastal regions; average TN in snow of Barrow is ∼0.13 mg L−1. The concentration of BC in snow was variable across Alaska and exhibited different spatial patterns, showing higher values in Barrow (∼1.55 μg L−1) and central Alaska (∼4.63 μg L−1), and lower values in eastern Alaska (∼0.27 μg L−1). Because of the impact of sea salt aerosols and mineral dust, larger concentrations of major ions appeared in the snow in northern Alaska, especially Cl− (∼187 μg L−1), Ca2+ (1753 μg L−1), and Mg2+ (64 μg L−1). The proportion of major ions exhibited differences from the south to the north. In Barrow and southern Alaska, Na+ and Cl- dominated the total ionic concentrations; while in the central to northern Alaska, Ca2+ is the predominant ion (). These different percentage of major ions indicated the different sources (natural or anthropogenic origins) of these major ions across the study area.
Fig. 1. Distribution of (a) DOC (mg L−1), (b) TN (mg L−1), (c) BC (μg L−1), and (d) major ions (μg L−1) in Alaskan snow cover in the spring of 2017. (Detailed sampling information can be referred to supplementary Table S1.).

Compared to other studies, DOC concentrations showed a wide range from the values reported in different areas (). Spatially, the lowest DOC content (about one order of magnitude lower) in snow was reported from the Greenland Ice Sheet (0.06 mg L−1) and Antarctica (Legrabd et al., Citation2013; Musilova et al., Citation2017). Our results were comparable to those from glacial surface snow in other Alaskan regions (Fellman et al., Citation2015), but lower than those reported for snow cover and mountain glaciers in central Asia (Liu et al., Citation2016; Yan et al., Citation2016; Li et al., Citation2018; Zhang et al., Citation2018, Citation2019; Gao et al., Citation2020). This indicated that the DOC in the snow of central Asia was more likely to be influenced by anthropogenic pollutant inputs and desert-sourced mineral dust deposition (Yan et al., Citation2016). However, the DOC concentration in a glacier in the French Alps was reported to be a little lower than that in central Asia (Legrand et al., Citation2013), which was comparable to that in our study. Ice core samples indicated that DOC in snow were extremely low Antarctic Ice Sheet (Legrand et al., Citation2013). However, DOC in dry valley glaciers in the Antarctica, as reported by Hood et al. (Citation2015), was more abundant; this value may have been affected by in situ productivities (Stibal et al., Citation2012) and may not be representative of the Antarctic Ice Sheet as a whole.
Table 1. DOC concentrations measured in snow of Alaska and Barrow and comparison with previous studies.
3.1.2. Temporal and vertical variations
Results from early April to late May, 2017, showed fluctuations in DOC, TN, and BC concentrations in snow cover on the sea ice of Barrow, but exhibited no significant trends (Fig. S2a). The parameters appeared to show consistent variations, except on April 27, when DOC showed a higher value, indicating different sources, transport, or deposition processes for DOC, TN, and BC. The lower values (e.g., on April 14 and May 5) were associated with the occurrence of fresh snow. Variations in DOC content differed from the variations in the types of major ions in the snow (Fig. S2b). Especially in late May, when the snow began to melt, DOC concentrations tended to accumulate while the major ions decreased due to elution. Nitrate (NO3−) in snow strongly differed compared to the other ions, implying the effects of a post-deposition process. Due to the short sampling term of this study, the seasonality of DOC in the snow at Barrow cannot be discussed further.
The snowpack profiles on the sea ice at Barrow indicated a generally consistent variations (). Overall, the DOC content of the surface snow (0.17 mg L−1) was higher than the subsurface snow (0.14 mg L−1) (). However, the profiles from SP4 (April 17, 2017) were anomalous, with higher values of DOC occurring in the subsurface snow as a result of snowdrift deposition during sampling. For SP1, SP3, and SP10, the highest values of DOC were observed at the bottom of the snowpack (). Profiles of TN in snow were mostly uniform, with higher values in the surface snow (). The BC profiles showed different variations across snowpacks (). Distinct differences in TN and BC were found in SP5 and SP9, showing lower TN and higher BC values in the surface snow. Similar vertical characteristics of BC were also found in Russian Arctic snow (Dou et al., Citation2012). Ca2+ in snow was usually considered to be an indicator of crustal dust in the mid-latitude regions (Zhang et al., Citation2015), while in the Polar Regions, Ca2+ may be affected by sea salt from coastal areas (Lambert et al., Citation2012). Thus, nssCa2+ (non-sea salt Ca2+) were calculated in this study based on method from Lambert et al. (Citation2012), which also showed similar variations with Ca2+ with a coefficient of 0.84 (p < 0.01) (Fig. S3). In Barrow, nssCa2+ were mainly affected by mineral dust. Both Cl– and Ca2+ had higher values in the surface snow at Barrow (), indicating that the impact of dust and sea salt aerosol on Cl– in snow of the study area. Besides, Cl– in snow can be affected by photochemistry within snow (Grannas et al., Citation2007). The NO3– and NH4+ levels in snow of coastal regions did not exhibit any systematic variations with depth (), maybe due to the multi sources from primary production in coastal and open ocean waters and anthropogenic input by long range transport. In general, concentrations of DOC and other proxies (BC, TN, and major ions) showed higher values in the surface snow rather than in the subsurface snow (). We assumed that most particulate snow impurities resulted from wet deposition, as suggested through global modeling in studies by Flanner et al. (Citation2007) and Koch et al. (Citation2009). The increasing concentration towards the surface indicated that the mixing ratio of insoluble light-absorbing particulates to snow mass was higher during late spring, when we sampled, compared to earlier in the accumulation season (Doherty et al., Citation2013). This could be the result of an increase in dry deposition of aerosols in the snow surface at this time.
3.2. Light-absorption properties of DOC
3.2.1. AAE
The AAE is a critical parameter in determining the impact of absorbing aerosols on the Earth’s radiation balance (Pokhrel et al., Citation2016), which can indicate the wavelength dependence of DOC’s light-absorbing properties. The AAE cannot be directly parameterized based on fuel type, because fuel type depends strongly on the burn conditions and there are no large datasets available to compare AAE to fuel type. Using EquationEq. 2(2)
(2) , we calculated the AAE of light-absorbing DOC in snow, providing important input data for the RF calculations. The fitted AAE330 − 400 values ranged from 1.16 to 4.22 for snow samples across Alaska, and from 0.23 to 7.21 for the snow at Barrow. The average AAE330 − 440 values were comparable to those of the Arctic snow (Doherty et al., Citation2010) (). However, AAE330 − 440 values measured in this study were lower than those reported by Voisin et al. (Citation2012) of HULIS in Arctic snow, and DOC in Tibetan glacial snow (Yan et al., Citation2016), and much lower than the values for the BrC aerosol (Kirchstetter et al., Citation2004; Sun et al., Citation2007; Chen & Bond, Citation2010; Lambe et al., Citation2013; Liu et al., Citation2015; Srinivas et al., Citation2016). The diversity in the AAE values in snow and aerosols may suggest differences in the chemical composition of DOC as a result of different sources or different degrees of atmospheric processing (Kirillova et al., Citation2014).
Table 2. Absorption Ångström Exponent (AAE330 − 400) and mass absorption cross section of DOC in snow across Alaska and at Barrow, as well as a comparison with other studies on snow and aerosols from other regions.
Typically, urban and industrial fossil fuel emissions have AAE values of 1.0 − 1.5 (Bergstrom et al., Citation2007), depending on the material being burned and the burn temperature, among other conditions. For example, the AAE value can be larger than 2 for aerosols from inefficient coal burning (Bond, Citation2001), and tends to be higher (1.5 − 2.5) for biomass-burning aerosols (Kirchstetter et al., Citation2004; Bergstrom et al., Citation2007). Mineral dust was common in Arctic snow (Doherty et al., Citation2010) and the AAE value for dust was poorly constrained and higher (2 − 5) than that for fossil fuel emissions (Bergstrom et al., Citation2007; Müller et al., Citation2009). The AAE values that we observed for snow indicated that they were heavily influenced by biomass-burning pollutants or dust.
3.2.2. Macdoc365 and SUVA254
The mass absorption cross section at 365 nm for DOC (MACDOC365) was selected in order to avoid interference from non-organic compounds such as nitrate and to be consistent with previous studies (Kirillova et al., Citation2014; Cheng et al., Citation2016; Yan et al., Citation2016). The average MACDOC365 value was 0.32 ± 0.24 m2 g−1 for the surface snow at Barrow, and 0.37 ± 0.32 m2 g−1 across Alaska (). Both values were lower than those for water-soluble organic carbon in an outflow from northern China (Kirillova et al., Citation2014) and for a receptor in the Indian Ocean (Bosch et al., Citation2014), and comparable to that for precipitation in the Tibetan Plateau (Li et al., Citation2017) (). These values were also lower than the MAC reported by Voisin et al. (Citation2012) for Arctic snow, and the DOC in glacial snow of the Tibetan Plateau (Yan et al., Citation2016), as well as those for typical urban aerosol in Beijing (Cheng et al., Citation2016) or biomass burning (Kirchstetter et al., Citation2004).
The MACDOC365 values from different sources, secondary contributions, or photobleaching processes vary widely (Kirillova et al., Citation2014). Generally, MACDOC365 from biomass smoke can reach 5.0 m2 g−1 (Kirchstetter et al., Citation2004), while that from secondary organic carbon ranges from 0.001 to 0.088 m2 g−1 (Lambe et al., Citation2013). This indicates that, at Barrow, DOC in the snow may be affected both by biomass burning and secondary organic carbon, as evidenced by the interval MACDOC365 values. In addition, mineral dust-sourced DOC may contribute to higher MACDOC365 values in snow samples as compared to the MAC values associated with secondary organic aerosols (Yan et al., Citation2016).
In general, MACDOC365 values observed in the Arctic snow had a negative correlation with DOC concentrations (), indicating that hydrophobic DOC attached to particles (Meyer et al., Citation2009). The negative relationship between the MACDOC365 and the AAE330 − 400 values () also suggested that the presence of more strongly absorbing DOC might contribute to lower AAE values, as observed in previous studies (Bosch et al., Citation2014; Kirillova et al., Citation2014; Yan et al., Citation2016). Temporal variations in MACDOC365 showed a rough increase during the sampling period, especially in late May when the snow began to melt (), which may indicate that snow melting can cause an increase of light-absorbing DOC. The above phenomenon had also been detected in glaciers in the Tibetan Plateau (Hu et al., Citation2018) and in snow cover of the Chinese Altai Mountains (Zhang et al., Citation2019).
Fig. 4. Relationship between (a) MACDOC365 values and DOC concentrations, (b) MACDOC365 values and AAE330-400, and (c) SUVA254 and DOC concentrations for Alaskan snow samples, and temporal variation in MACDOC365 values (d), and SUVA254 Values (e) in snow cover on the sea ice at Barrow during the study period of April to May in 2017.

SUVA254 was mainly affected by the percent aromaticity of dissolved organic matter (Weishaar et al., Citation2003), which can be used to obtain information on organic matter in water, in terms of aromaticity (Assaad et al., Citation2017). SUVA254 for the snow at Barrow ranged from 1.1 to 6.8 L mg C−1 m−1, with an average of 3.5 ± 1.3 L mg C−1 m−1, which showed a negative exponent with DOC concentrations (). There was no significant trend for SUVA254 during the study period (), indicating the consistent presence of aromaticity in snow.
3.2.3. Fraction of RF by DOC relative to BC
Based on EquationEq. 3(3)
(3) , the fractions of RF by DOC relative to that by BC were calculated. Ratios of RF by DOC to BC ranged from 0.86% to 9.08% (average at 2.28% ± 1.53%) for Arctic snow (Supplementary Table S3). Temporal variations in the ratios showed no obvious trends (), which was negatively correlated with MACDOC365 in the snow at Barrow (). This indicated that, during snow melting when high values of MACDOC365 were observed, the RF caused by enriched BC in the snow enhanced much more than that of the DOC. The high RF ratio may be a result of the high ratio of DOC to BC concentration (DOC/BC) (), which has also been noted in glacial snow in the Tibetan Plateau (Yan et al., Citation2016).
Fig. 5. Variation for ratios (a) of RF by DOC to BC, and relationships between ratios of RF by DOC to BC and (b) MACDOC365 and (c) ratios of DOC/BC in the snow at Barrow, Alaska.

Recently, the role of absorbance of light-absorbing OC in snow has been highlighted. Doherty et al. (Citation2010) noted that OC in Arctic snow was typically responsible for ∼40% of the absorption of visible and ultraviolet light. RF by DOC relative to that of BC ranged from 2.1% to 30.4% (average at 9.5% ± 8.4%) in glacial snow in the northern Tibetan Plateau (Yan et al., Citation2016). Simulations by Lin et al. (Citation2014) indicated that the RF of OC deposited in terrestrial snow and sea ice accounted for 24% of the forcing by BC. In this study, the RF in snow by DOC relative to BC was lower than the observations made by other researchers; this may be due to the low DOC concentrations and the high latitude. Further investigation is needed to understand the post-deposition processes that may reduce the light-absorption of OC, including mixing with other non-absorbing constituents, scavenging efficiency, sublimation, or photochemical reactions.
3.3. Bioavailability of DOC
BDOC experiments in the snow found that DOC levels decreased exponentially over time (r2 = 0.81) (), with approximately 60% of DOC degrading within 15 days. Taking into consideration the different experiment conditions, our results showed higher BDOC consumption compared to studies in European Alpine glaciers (59% within 50 days, at 4° C) (Singer et al., Citation2012) and Tibetan glaciers (26.7% within 15 days at 3.8° C) (Spencer et al., Citation2014; Yan et al., Citation2016). A study by Lawson et al. (Citation2014), in a large Greenland Ice Sheet catchment during contrasting melt seasons, indicated a 46 − 64% decline in DOC during glacial incubation (glacier samples only) and exceeded the average DOC decline (17%) in the glacial controls over the same time period. These results suggest the utilization of DOC by microbial heterotrophs in snow and glacier regions (Hood et al., Citation2009; Yan et al., Citation2016). For Alpine glaciers, Singer et al. (Citation2012) found that the percentage of BDOC increased with Δ14C DOC, and showed that radiocarbon age explained only 23% of the variation in BDOC across the studied glaciers. This indicated that complementary biogeochemical factors (for example, chemical composition and sources) can influence the bioavailability of snow-linked DOC. Currently, we did not analyze the Δ14C DOC and the biogeochemistry of DOC, thus limiting further discussion.
3.4. Potential sources of DOC
Backward trajectory analyses indicated that the trajectories arriving at Barrow spread quite a lot, but essentially remain in the northern and southern latitudes (), especially from the Arctic Ocean and Alaskan regions, which may have some distinctly different characteristics. For example, backward air mass trajectories showed that the air masses arriving at Barrow on April 17, 2017 had passed over the Canadian Arctic and Alaska (), while air masses arriving at Barrow on May 2, 2017 came from the Arctic Ocean (). This evidenced strong marine and terrestrial sources for organic carbon in Barrow. Distant aerosol transport and the deposition of terrestrial carbon have also been reported in snowpacks and ice cores in other remote locations, such as Greenland (Grannas et al., Citation2004) and the Russian Arctic (Grannas et al., Citation2006). Terrestrial DOM in a glacial ecosystem was found in a highly bioavailable form (Lawson et al., Citation2014; Fellman et al., Citation2015) and was also a significant driver of bacterial production in aquatic systems (Pollard & Ducklow, Citation2011).
Fig. 7. Footprint analyses of the backward trajectories launched over the snow sampling site at Barrow, Alaska, during April and May in 2017 for pass by (a) 48 h and (b) 120 h, respectively, and HYSPLIT model seven day back trajectories for air masses arriving in Barrow on (c) April 17, 2017 and (d) May 2, 2017. (Different colors of backward trajectories in c and d represent the air mass arrived at the different date.).

PCA analysis of chemical impurities in the snow at Barrow revealed that the first component (PCA1), which accounted for 34.41% of the variance, was dominated by Cl−, Na+, and K+ (), indicating that they arise mostly from marine sources (i.e., natural sources). PCA2 accounted for 24.04% of the total variability and consisted primarily of anthropogenic impurities (SO42−, NO3−, NH4+, and BC). DOC was largely loaded in PCA3 with Ca2+ and TN. When we consider the snow across Alaska, PCA1 (35.48%, mainly loaded with Ca2+, Mg2+, SO42−) and PCA2 (18.92%, mainly loaded with DOC, Na+, K+, and Cl−) represented the crustal origins and impacts of marine aerosols/biomass burning, respectively. Beside the impact of sea salt and anthropogenic impact, sulfate in snow may be affected by mineral dust input (Zhang et al., Citation2016). In this study, nssSO42 was positively correlated with sulfate concentrations (Fig. S4a). There was almost no relationship between DOC and SO42- and nssSO42- (Fig. S4b,c). While, positive correlation was also found between nssSO42- and Ca+, and Mg2+, further indicating the important impact of mineral dust. Previous study of 87Sr/86Sr and εNd(0) data from the sea ice snow samples from the Arctic Ocean and snow samples from Barrow (Alaska) also suggested potential long-range dust transport to the Arctic region (Du et al., Citation2019).
Table 3. Principal component analysis of DOC with other parameters in snow over across the Alaska and on sea ice at Barrow.
Fellman et al. (Citation2015) found that DOC in snow collected across Alaska contained high amounts of K+, indicating biomass burning sources, as evidenced by the source-diagnostic dual-isotope composition of the DOC. Dou et al. (Citation2017) observed that polyaromatic hydrocarbons and hopanes were below the detection limits, indicating that fossil fuel combustion (anthropogenic sources) contributes little to the OC in the Barrow snow. Biomass burning is widespread in Alaska (Kelly et al., Citation2013; Mouteva et al., Citation2015), and appears to contribute to the OC in the Barrow snow (Dou et al., Citation2017). Biplot analysis indicated that DOC in the snow in Barrow had a negative relationship with Na+, K+, Cl−, Ca2+, Mg2+, and SO42− (). In terms of snow across Alaska, DOC had a positive correlation with Na+, K+, and Cl-. Since the occurrence of fires in Alaska was quite low (Fig. S5) and many trajectories pass over the Arctic Ocean (), the impact of biomass burning on DOC deposited in the snow at Barrow may not be significant. However, the lack of data related to the chemical composition of the DOM in snow and its carbon isotopic signatures has confined this study to quantifying the different contributions to carbon aerosols.
Fig. 8. Biplot analyses of DOC with other parameters for (a) snow samples in Barrow, and (b) snow samples across Alaska.

Snowpack cycling of DOC can be important on the local, regional, and global scales (McNeill et al., Citation2012). OC depositions in snow and sea ice may originate from plant material, microorganisms and their excretions, aerosols, or volatile organic compounds deposited from the atmosphere (Pautler et al., Citation2011). Fellman et al. (Citation2015) used carbon isotopic signatures (δ13C and Δ14C) combined with a three-source mixing model and found that the DOC deposited in snow across the icefields of southeast Alaska came mainly from fossil fuel combustion products (43 − 73%), and to a smaller extent from marine (21–41%) and terrestrial (1 − 26%) sources. In Antarctica, OC in snow was consistent with materials from microbial sources as well as terrestrial inputs of vascular plant-derived materials (Antony et al., Citation2014).Carbonaceous isotopes in the snow should be further investigated. Simulations coupled with an emission inventory should also be considered in the future.
3.5. Climatic implications of DOC for the Arctic region
The Arctic is of special importance to the world due to its amplification of temperature, consequently changes in atmospheric and oceanic circulation, and as well as reductions in snow cover and sea ice (ACIA, Citation2004; Derksen et al., Citation2015). Such changes can affect carbon and nitrogen cycling, as well as methane emissions (AMAP, Citation2015; Bokhorst et al., Citation2016), and are thus significant effects for impact assessments and developing adaptation strategies.
Sea ice and snow cover contain BC and other light-absorbing impurities (Flanner et al., Citation2009; Doherty et al., Citation2010; Dou et al., Citation2012; Legrand et al., Citation2013; Dou et al., Citation2017; Marks et al., Citation2017). Light-absorbing impurities in snow can dominate the absorption of solar radiation, reduce the albedo relative to clean snow, contribute to the surface energy budget, and lead to earlier snowmelt (Flanner et al., Citation2007; Doherty et al., Citation2010; Derksen et al., Citation2015; Skiles et al., Citation2018). In a model simulation by Lin et al. (Citation2014), the OC reduced the snow albedo, resulting in RF of organic aerosols deposited in land snow and sea ice ranging from +0.0011 to +0.0031 W m−2, which could potentially enhance the snowmelt (Flanner et al., Citation2009; Dumont et al., Citation2014).
Our findings indicated that light-absorbing DOC has the potential ability to absorb more solar energy and accelerate snow melting. Schmale et al. (Citation2017) reported that snow darkening caused by BC can enhance summer snowmelt rises of up to 6.3% (7 cm w.e. a−1) on the glaciers of central Asia, based on an albedo reduction and snowmelt model. Zhang et al. (Citation2017) also calculated that BC in snow enhanced glacial melt by approximately 15% in the south-eastern Tibetan Plateau. In the Arctic region, a simulation by the Community Earth System Model reported that the deposition of light-absorbing impurities caused 1 − 2° C of surface warming over a large area of the Arctic Ocean and subarctic seas in autumn and winter (Goldenson et al., Citation2012). Light-absorbing impurities contributed between 1.6%−5.1% to the reduction of albedo with snowmelt during April and May, 2015 (Dou et al., Citation2017). Changing snow cover and sea ice in the Arctic region has been linked to cold extremes in the mid-latitude and northern continents/subarctic areas (Cohen et al., Citation2013). The amplified Arctic warming over the past decade has significantly contributed to a continual global warming trend (Huang et al., Citation2017; IPCC, Citation2019). Quantifying the impact of light-absorbing impurities on changes in snow cover and sea ice, and understanding the mitigation strategies for these effects are immediate concerns. Improved understanding of processes, additional Arctic observations, and further modeling efforts based on observed data are required to elucidate the connections with the Arctic (Cohen et al., Citation2014).
4. Conclusions
This study investigated the characteristics of DOC in snow cover across the Alaska and on sea ice at Barrow, further confirming that DOC in snow can significantly affect light-absorption in the Arctic regions. Average DOC concentrations in snow cover at Barrow (∼0.17 mg L−1) and in southern Alaska were lower than those measured in central Alaska (0.33 mg L−1). DOC levels in snow cover (0.17 − 0.30 mg L−1) were comparable to those in glacial surface snow in Alaska, but lower than those in mountain glaciers in Central Asia. No significant temporal variation in DOC concentrations was noted at Barrow, due to the observations were recorded over a short time period. TN and BC in snow of Alaska showed spatial distribution with lower concentrations in the eastern Alaska (with 0.05 mg L−1 and 0.24 mg L−1 for TN and BC respectively). While major ions in snow show higher total concentrations in the northern Alaska, with Na+ and Cl- dominated in Barrow and southern Alaska and Ca2+ dominated in the central to northern Alaska due to different source impacts. For snowpack, vertical variations of DOC and other related proxies generally showed higher values in the surface snow than that in the sub-surface snow.
The light-absorption results showed that AAE330 − 400 values of DOC in snow were 2.11 and 2.34 at Barrow and across Alaska, respectively, which were comparable to those in the Arctic snow, but lower than the values of HULIS in Arctic snow, DOC in Tibetan glaciers, and BrC aerosols. MACDOC365 values of DOC were lower than those of aerosol, which was comparable to those for precipitation in the Tibetan Plateau. MACDOC365 was generally negatively correlated with DOC concentrations, indicating hydrophobic DOC attached to particles in the snow. The results show that the ratios of RF by DOC relative to BC ranged from 0.86% to 9.08% for Arctic snow.
DOC concentrations decreased exponentially over time, with approximately 60% of DOC degrading within 15 days. This highlighted the need to further quantify and determine the source of organic matter input to snow meltwater, impacted ecosystems, and heterotrophic activity. Based on the PCA analysis and the modeled back trajectories, we found that marine and terrestrial sources contributed strongly to DOC deposition on the Alaskan snow cover. Our findings also emphasized the importance of the light-absorbing properties of DOC in snow and its climatic implications for the Arctic.
This study focused on the characteristics of DOC in Arctic snow and highlighted its potential roles on RF. Further studies need to be carried out to understand these roles more thoroughly, including expanded sampling across seasons and process studies. Monitoring the snowmelt process at Barrow will help us better understand the redistribution and scavenging of DOC in snow, and a controlled experiment will help verify the radiative-transfer modeling of albedo reduction attributed to DOC.
Disclosure statement
There was no potential conflict of interest.
Additional information
Funding
References
- ACIA (Arctic Climate Impact Assessment). 2004. Impacts of a WarmingArctic: Arctic Climate Impact Assessment. Cambridge University Press, Cambridge, UK.
- AMAP (Arctic Monitoring and Assessment Programme). 2015. AMAP Assessment 2015: Black Carbon and Ozone as Arctic Climate Forcers. Arctic Monitoring and Assessment Programme (AMAP): Oslo, Norway.
- Andreae, M. O. and Gelencser, A. 2006. Black carbon or brown carbon? The nature of light-absorbing carbonaceous aerosols. Atmos. Chem. Phys. 6, 3131–3148. doi:10.5194/acp-6-3131-2006
- Anesio, A. M., Hodson, A. J., Fritz, A., Psenner, R. and Sattler, B. 2009. High microbial activity on glaciers: importance to the global carbon cycle. Global Change Biol. 15, 955–960. doi:10.1111/j.1365-2486.2008.01758.x
- Antony, R., Grannas, A. M., Willoughby, A. S., Sleighter, R. L., Thamban, M. and co-authors. 2014. Origin and sources of dissolved organic matter in snow on the East Antarctic ice sheet. Environ. Sci. Technol. 48, 6151–6159. doi:10.1021/es405246a
- Antony, R., Mahalinganathan, K., Thamban, M. and Nair, S. 2011. Organic carbon in Antarctic snow: spatial trends and possible sources. Environ. Sci. Technol. 45, 9944–9950. doi:10.1021/es203512t
- Assaad, A., Pontvianne, S. and Pons, M.-N. 2017. Assessment of organic pollution of an industrial river by synchronous fluorescence and UV-vis spectroscopy: the Fensch River (NE France)). Environ. Monit. Assess. 189, 229. doi:10.1007/s10661-017-5933-3
- Bergstrom, R. W., Pilewskie, P., Russell, P. B., Redemann, J., Bond, T. C. and co-authors. 2007. Spectral absorption properties of atmospheric aerosols. Atmos. Chem. Phys. 7, 5937–5943. doi:10.5194/acp-7-5937-2007
- Bokhorst, S., Pedersen, S. H., Brucker, L., Anisimov, O., Bjerke, J. W. and co-authors. 2016. Changing Arctic snow cover: a review of recent developments and assessment of future needs for observations, modelling, and impacts. AMBIO 45, 516–537. doi:10.1007/s13280-016-0770-0
- Bond, T. C. 2001. Spectral dependence of visible light absorption by carbonaceous particles emitted from coal combustion. Geophys. Res. Lett. 28, 4075–4078. doi:10.1029/2001GL013652
- Bond, T. C. and Bergstrom, R. W. 2006. Light absorption by carbonaceous particles: an investigative review. Aerosol Sci. Technol. 40, 27–67. doi:10.1080/02786820500421521
- Bond, T. C., Doherty, S. J., Fahey, D., Forster, P., Berntsen, T. and co-authors. 2013. Bounding the role of black carbon in the climate system: a scientific assessment. J. Geophys. Res. Atmos. 118, 5380–5552. doi:10.1002/jgrd.50171
- Bosch, C., Andersson, A., Kirillova, E., Budhavant, K., Tiwari, S. and co-authors. 2014. Source-diagnostic dual-isotope composition and optical properties of water-soluble organic carbon and elemental carbon in the South Asian outflow intercepted over the Indian Ocean. J. Geophys. Res. Atmos. 119, 11,743–11,759. doi:10.1002/2014JD022127
- Callaghan, T. V., Johansson, M., Brown, R., Groisman, P. Y., Labba, N. and co-authors. 2011. The changing face of arctic snow cover: a synthesis of observed and projected changes. AMBIO 40, 17–31. doi:10.1007/s13280-011-0212-y
- Chen, Y. and Bond, T. C. 2010. Light absorption by organic carbon from wood combustion. Atmos. Chem. Phys. 10, 1773–1787. doi:10.5194/acp-10-1773-2010
- Cheng, Y., He, K., Du, Z., Engling, G., Liu, J. and co-authors. 2016. The characteristics of brown carbon aerosol during winter in Beijing. Atmos. Environ. 127, 355–364. doi:10.1016/j.atmosenv.2015.12.035
- Cohen, J., Furtado, J. C., Jones, J., Barlow, M., Whittleston, D. and co-authors. 2014. Linking Siberian snow cover to precursors of stratospheric variability. J. Clim. 27, 5422–5432. doi:10.1175/JCLI-D-13-00779.1
- Cohen, J., Jones, J., Furtado, J. C. and Tziperman, E. 2013. Warm Arctic, cold continents: A common pattern related to Arctic sea ice melt, snow advance, and extreme winter weather. Oceanography 26, 150–160. https://dx.doi.org/10.5670/oceanog.2013.70
- Cooper, E. J. 2014. Warmer shorter winters disrupt Arctic terrestrial ecosystems. Annu. Rev. Ecol. Evol. Syst. 45, 271–295. doi:10.1146/annurev-ecolsys-120213-091620
- D’Amore, D. V., Edwards, R. T., Herendeen, P. A., Hood, E. and Fellman, J. B. 2015. Dissolved organic carbon fluxes from hydropedologic units in Alaskan coastal temperate rainforest watersheds. Soil Sci. Soc. Am. J. 79, 378–388. doi:10.2136/sssaj2014.09.0380
- Derksen, C., Brown, R., Mudryk, L. and Luojus, K. 2015. Arctic: terrestrial snow cover (in State of the Climate in 2014). Bull. Am. Meteorol. Soc. 96, S133–S135.
- Doherty, S. J., Grenfell, T. C., Forsström, S., Hegg, D. L., Brandt, R. E. and co-authors. 2013. Observed vertical redistribution of black carbon and other insoluble light-absorbing particles in melting snow. J. Geophys. Res. Atmos. 118, 5553–5569. doi:10.1002/jgrd.50235
- Doherty, S. J., Warren, S. G., Grenfell, T. C., Clarke, A. D. and Brandt, R. E. 2010. Light-absorbing impurities in Arctic snow. Atmos. Chem. Phys. 10, 11647–11680. doi:10.5194/acp-10-11647-2010
- Dou, T., Xiao, C., Du, Z., Schauer, J. J., Ren, H. and co-authors. 2017. Sources, evolution and impacts of EC and OC in snow on sea ice: a measurement study in Barrow, Alaska. Sci. Bull 62, 1547–1554. doi:10.1016/j.scib.2017.10.014
- Dou, T., Xiao, C., Shindell, D. T., Liu, J., Eleftheriadis, K. and co-authors. 2012. The distribution of snow black carbon observed in the Arctic and compared to the GISS-PUCCINI model. Atmos. Chem. Phys. 12, 7995–8007. doi:10.5194/acp-12-7995-2012
- Draxler, R. R. and Rolph, G. D. 2010. Hysplit (hybrid single-particle lagrangian integrated trajectory) model access via NOAA ARL ready website. Online at: http://ready.arl.noaa.gov/HYSPLIT.php
- Du, Z., Xiao, C., Dou, T., Li, S., An, H. and co-authors. 2019. Comparison of Sr-Nd-Pb isotopes in insoluble dust between northwestern China and high-latitude regions in the Northern Hemisphere. Atmos. Environ. 214, 116837. doi:10.1016/j.atmosenv.2019.116837
- Dumont, M., Brun, E., Picard, G., Michou, M., Libois, Q. and co-authors. 2014. Contribution of light-absorbing impurities in snow to Greenland’s darkening since 2009. Nature Geosci. 7, 509–512. doi:10.1038/ngeo2180
- Fellman, J. B., Hood, E., Raymond, P. A., Stubbins, A. and Spencer, R. G. M. 2015. Spatial variation in the origin of dissolved organic carbon in snow on the Juneau icefield, Southeast Alaska. Environ. Sci. Technol. 49, 11492–11499. doi:10.1021/acs.est.5b02685
- Flanner, M. G., Zender, C. S., Hess, P. G., Mahowald, N. M., Painter, T. H. and co-authors. 2009. Springtime warming and reduced snow cover from carbonaceous particles. Atmos. Chem. Phys. 9, 2481–2497. doi:10.5194/acp-9-2481-2009
- Flanner, M. G., Zender, C. S., Randerson, J. T. and Rasch, P. J. 2007. Present-day climate forcing and response from black carbon in snow. J. Geophys. Res 112, D11202. doi:10.1029/2006JD008003
- Gao, T., Kang, S., Chen, R., Zhang, T., Zhang, T. and co-authors. 2019. Riverine dissolved organic carbon and its optical properties in a permafrost region of the Upper Heihe River basin in the northern Tibetan Plateau. Sci. Total Environ. 686, 370–381. doi:10.1016/j.scitotenv.2019.05.478
- Gao, T., Kang, S., Zhang, Y., Sprenger, M., Wang, F. and co-authors. 2020. Characterization, sources and transport of dissolved organic carbon and nitrogen from a glacier in the Central Asia. Sci. Total Environ. 725, 138346. doi:10.1016/j.scitotenv.2020.138346
- Goldenson, N., Doherty, S. J., Bitz, C. M., Holland, M. M., Light, B. and co-authors. 2012. Arctic climate response to forcing from light-absorbing particles in snow and sea ice in CESM. Atmos. Chem. Phys. 12, 7903–7920. doi:10.5194/acp-12-7903-2012
- Grannas, A. M., Hockaday, W. C., Hatcher, P. G., Thompson, L. G. and Mosley‐Thompson, E. 2006. New revelations on the nature of organic matter in ice cores. J. Geophys. Res. 111, D04304.
- Grannas, A. M., Jones, A. E., Dibb, J. E., Ammann, M., Anastasio, C. and co-authors. 2007. An overview of snow photochemistry: evidence, mechanisms and impacts. Atmos. Chem. Phys. 7, 4329–4373. doi:10.5194/acp-7-4329-2007
- Grannas, A. M., Shepson, P. B. and Filley, T. R. 2004. Photochemistry and nature of organic matter in Arctic and Antarctic snow. Global Biogeochem. Cycle 18, GB1006.
- Hood, E., Battin, T. J., Fellman, J., O'Neel, S. and Spencer, R. G. M. 2015. Storage and release of organic carbon from glaciers and ice sheets. Nat. Geosci. 8, 91–96. doi:10.1038/ngeo2331
- Hood, E., Fellman, J. B., Spencer, R. G. M., Hernes, P. J., Edwards, R. T. and co-authors. 2009. Glaciers as a source of ancient and labile organic matter to the marine environment. Nature 462, 1044–1047. doi:10.1038/nature08580
- Hu, Z., Kang, S., Yan, F., Zhang, Y., Li, Y. and co-authors. 2018. Dissolved organic carbon fractionation accelerates glacier-melting: a case study in the northern Tibetan Plateau. Sci. Total Environ. 627, 579–585. doi:10.1016/j.scitotenv.2018.01.265
- Huang, J., Zhang, X., Zhang, Q., Lin, Y., Hao, M. and co-authors. 2017. Recently amplified arctic warming has contributed to a continual global warming trend. Nat. Clim. Change 7, 875–879. doi:10.1038/s41558-017-0009-5
- Huntington, T. G., Balch, W. M., Aiken, G. R., Sheffield, J., Luo, L. and co-authors. 2016. Climate change and dissolved organic carbon export to the Gulf of Maine. J. Geophys. Res. Biogeosci. 121, 2700–2716. doi:10.1002/2015JG003314
- IPCC. 2019. IPCC Special Report on the Ocean and Cryosphere in a Changing Climate (eds. H.-O. Pörtner, D. C. Roberts, V. Masson-Delmotte, P. Zhai, M. Tignor, E. Poloczanska, K. Mintenbeck, M. Nicolai, A. Okem, J. Petzold, B. Rama, N. Weyer]. https://www.ipcc.ch/srocc/.
- Kelly, R., Chipman, M. L., Higuera, P. E., Stefanova, I., Brubaker, L. B. and co-authors. 2013. Recent burning of boreal forests exceeds fire regime limits of the past 10,000 years. Proc. Natl. Acad. Sci. USA. 110, 13055–13060. doi:10.1073/pnas.1305069110
- Kim, Y., Hatsushika, H., Muskett, R. R. and Yamazaki, K. 2005. Possible effect of boreal wildfire soot on Arctic sea ice and Alaska glaciers. Atmos. Environ. 39, 3513–3520. doi:10.1016/j.atmosenv.2005.02.050
- Kirchstetter, T. W., Novakov, T. and Hobbs, P. V. 2004. Evidence that the spectral dependence of light absorption by aerosols is affected by organic carbon. J. Geophys. Res. 109, D21208. doi:10.1029/2004JD004999
- Kirillova, E. N., Andersson, A., Han, J., Lee, M. and Gustafsson, Ö. 2014. Sources and light absorption of water-soluble organic carbon aerosols in the outflow from northern China. Atmos. Chem. Phys. 14, 1413–1422. doi:10.5194/acp-14-1413-2014
- Koch, D., Menon, S., Del Genio, A., Ruedy, R., Alienov, I. and co-authors. 2009. Distinguishing aerosol impacts on climate over the past century. J. Clim. 22, 2659–2677. doi:10.1175/2008JCLI2573.1
- Lambe, A. T., Cappa, C. D., Massoli, P., Onasch, T. B., Forestieri, S. and co-authors. 2013. Relationship between oxidation level and optical properties of secondary organic aerosol. Environ. Sci. Technol. 47, 6349–6357. doi:10.1021/es401043j
- Lambert, F., Bigler, M., Steffensen, J. P., Hutterli, M. and Fischer, H. 2012. Centennial mineral dust variability in high-resolution ice core data from Dome C, Antarctica. Clim. Past 8, 609–623. doi:10.5194/cp-8-609-2012
- Lawson, E. C., Wadham, J. L., Tranter, M., Stibal, M., Lis, G. P. and co-authors. 2014. Greenland Ice Sheet exports labile organic carbon to the Arctic oceans. Biogeosciences 11, 4015–4028. doi:10.5194/bg-11-4015-2014
- Legrand, M., Preunkert, S., Jourdain, B., Guilhermet, J., Fa{Ï}N, X. and co-authors. 2013. Water-soluble organic carbon in snow and ice deposited at Alpine, Greenland, and Antarctic sites: a critical review of available data and their atmospheric relevance. Clim. Past 9, 2195–2211. doi:10.5194/cp-9-2195-2013
- Levinson, R., Akbari, H. and Berdahl, P. 2010. Measuring solar reflectance–Part I: defining a metric that accurately predicts solar heat gain. Sol. Energy 84, 1717–1744. doi:10.1016/j.solener.2010.04.018
- Li, C., Yan, F., Kang, S., Chen, P., Han, X. and co-authors. 2017. Re-evaluating black carbon in the Himalayas and the Tibetan Plateau: concentrations and deposition. Atmos. Chem. Phys. 17, 11899–11912. doi:10.5194/acp-17-11899-2017
- Li, X., Ding, Y., Xu, J., He, X., Han, T. and co-authors. 2018. Importance of mountain glaciers as a source of dissolved organic carbon. J. Geophys. Res. Earth Surf. 123, 2123–2134.
- Lin, G., Penner, J. E., Flanner, M. G., Sillman, S., Xu, L. and co-authors. 2014. Radiative forcing of organic aerosol in the atmosphere and on snow: effects of SOA and brown carbon. J. Geophys. Res. Atmos. 119, 7453–7476. doi:10.1002/2013JD021186
- Liu, J., Scheuer, E., Dibb, J. E., Diskin, G. S., Ziemba, L. D. and co-authors. 2015. Brown carbon aerosol in the North American continental troposphere: sources, abundance, and radiative forcing. Atmos. Chem. Phys. 15, 7841–7858. doi:10.5194/acp-15-7841-2015
- Liu, Y. M., Xu, J., Kang, S., Li, X. and Li, Y. 2016. Storage of dissolved organic carbon in Chinese glaciers. J. Glaciol. 62, 402–406. doi:10.1017/jog.2016.47
- Marks, A. A., Lamare, M. L. and King, M. D. 2017. Optical properties of sea ice doped with black carbon–an experimental and radiative-transfer modelling comparison. Cryosphere 11, 2867–2881. doi:10.5194/tc-11-2867-2017
- McNeill, V. F., Grannas, A. M., Abbatt, J. P. D., Ammann, M., Ariya, P. A. and co-authors. 2012. Organics in environmental ices: sources, chemistry, and impacts. Atmos. Chem. Phys. 12, 9653–9678. doi:10.5194/acp-12-9653-2012
- Meyer, T., Lei, Y. D., Muradi, I. and Wania, F. 2009. Organic contaminant release from melting snow. 1. Influence of chemical partitioning. Environ. Sci. Technol. 43, 657–662. doi:10.1021/es8020217
- Mouteva, G. O., Czimczik, C. I., Fahrni, S. M., Wiggins, E. B., Rogers, B. M. and co-authors. 2015. Black carbon aerosol dynamics and isotopic composition in Alaska linked with boreal fire emissions and depth of burn in organic soils. Glob. Biogeochem. Cycles 29, 1977–2000. doi:10.1002/2015GB005247
- Müller, T., Nowak, A., Wiedensohler, A., Sheridan, P., Laborde, M. and co-authors. 2009. Angular illumination and truncation of three different integrating nephelometers: implications for empirical, size-based corrections. Aerosol Sci. Technol. 43, 581–586. https://doi.org/ doi:10.1080/02786820902798484
- Musilova, M., Tranter, M., Wadham, J., Telling, J., Tedstone, A. and co-authors. 2017. Microbially driven export of labile organic carbon from the Greenland ice sheet. Nat. Geosci. 10, 360–365. https://doi.org/ doi:10.1038/ngeo2920
- O’Donnell, J. A., Aiken, G. R., Swanson, D. K., Panda, S., Butler, K. D. and co-authors. 2016. Dissolved organic matter composition of Arctic rivers: linking permafrost and parent material to riverine carbon. Glob. Biogeochem. Cycles 30, 1811–1826. doi:10.1002/2016GB005482
- Pautler, B. G., Simpson, A. J., Simpson, M. J., Tseng, L.-H., Spraul, M. and co-authors. 2011. Detection and structural identification of dissolved organic matter in Antarctic glacial ice at natural abundance by SPR-W5-WATERGATE 1H NMR spectroscopy. Environ. Sci. Technol. 45, 4710–4717. doi:10.1021/es200697c
- Ping, C., Michaelson, G. J., Jorgenson, M. T., Kimble, J. M., Epstein, H. E. and co-authors. 2008. High stocks of soil organic carbon in the North American Arctic region. Nat. Geosci. 1, 615–619. doi:10.1038/ngeo284
- Pithan, F. and Mauritsen, T. 2014. Arctic amplification dominated by temperature feedbacks in contemporary climate models. Nat. Geosci. 7, 181–184. doi:10.1038/ngeo2071
- Pokhrel, R. P., Wagner, N. L., Langridge, J. M., Lack, D., Jayarathne, T. and co-authors. 2016. Parameterization of single scattering albedo (SSA) and absorption Ångström exponent (AAE) with EC/OC for aerosol emissions from biomass burning. Atmos. Chem. Phys. 16, 9549–9561. doi:10.5194/acp-16-9549-2016
- Pollard, P. C. and Ducklow, H. 2011. Ultrahigh bacterial production in a eutrophic subtropical Australian river: Does viral lysis short‐circuit the microbial loop? Limnol. Oceanogr. 56, 1115–1129. doi:10.4319/lo.2011.56.3.1115
- Qian, Y., Yasunari, T. J., Doherty, S. J., Flanner, M. G., Lau, W. K. M. and co-authors. 2015. Light-absorbing particles in snow and ice: measurement and modeling of climatic and hydrological impact. Adv. Atmos. Sci. 32, 64–91. doi:10.1007/s00376-014-0010-0
- Schmale, J., Flanner, M., Kang, S., Sprenger, M., Zhang, Q. and co-authors. 2017. Modulation of snow reflectance and snowmelt from Central Asian glaciers by anthropogenic black carbon. Sci. Rep. 7, 40501 doi:10.1038/srep40501
- Singer, G., Fasching, C., Wilhelm, L., Niggemann, J., Steier, P. and co-authors. 2012. Biogeochemically diverse organic matter in Alpine glaciers and its downstream fate. Nat. Geosci. 5, 710–714. doi:10.1038/ngeo1581
- Skiles, S. M., Flanner, M., Cook, J. M., Dumont, M. and Painter, T. H. 2018. Radiative forcing by light-absorbing particles in snow. Nat. Clim Change 8, 964–917. doi:10.1038/s41558-018-0296-5
- Spencer, R. G. M., Hernes, P. J., Dinga, B., Wabakanghanzi, J. N., Drake, T. W. and co-authors. 2016. Origins, seasonality, and fluxes of organic matter in the Congo River. Glob. Biogeochem. Cycles 30, 1105–1121. doi:10.1002/2016GB005427
- Spencer, R. G. M., Vermilyea, A. W., Fellman, J. B., Raymond, P. A., Stubbins, A. and co-authors. 2014. Seasonal variability of organic matter composition in an Alaskan glacier outflow: insights into glacier carbon sources. Environ. Res. Lett. 9, 055005. doi:10.1088/1748-9326/9/5/055005
- Sprenger, M. and Wernli, H. 2015. The LAGRANTO Lagrangian analysis tool – version 2.0. Geosci. Model Dev. 8, 2569–2586. doi:10.5194/gmd-8-2569-2015
- Srinivas, B., Rastogi, N., Sarin, M. M., Singh, A. and Singh, D. 2016. Mass absorption efficiency of light absorbing organic aerosols from source region of paddy-residue burning emissions in the Indo-Gangetic Plain. Atmos. Environ 125, 360–370. doi:10.1016/j.atmosenv.2015.07.017
- Stibal, M., Lawson, E. C., Lis, G. P., Mak, K. M., Wadham, J. L. and co-authors. 2010. Organic matter content and quality in supraglacial debris across the ablation zone of the Greenland ice sheet. Ann. Glaciol. 51, 1–8. https://doi.org/ doi:10.3189/172756411795931958
- Stibal, M., Wadham, J. L., Lis, G. P., Telling, J., Pancost, R. D. and co-authors. 2012. Methanogenic potential of Arctic and Antarctic subglacial environments with contrasting organic carbon sources. Glob. Change Biol. 18, 3332–3345. doi:10.1111/j.1365-2486.2012.02763.x
- Stubbins, A. and Dittmar, T. 2012. Low volume quantification of dissolved organic carbon and dissolved nitrogen. Limnol. Oceanogr. Methods 10, 347–352. doi:10.4319/lom.2012.10.347
- Stubbins, A., Hood, E., Raymond, P. A., Aiken, G. R., Sleighter, R. L. and co-authors. 2012. Anthropogenic aerosols as a source of ancient dissolved organic matter in glaciers. Nat. Geosci. 5, 198–201. doi:10.1038/ngeo1403
- Sun, H., Biedermann, L. and Bond, T. C. 2007. Color of brown carbon: a model for ultraviolet and visible light absorption by organic carbon aerosol. Geophys. Res. Lett. 34, L17813. doi:10.1029/2007GL029797
- Telling, J., Anesio, A. M., Tranter, M., Stibal, M., Hawkings, J. R. and co-authors. 2012. Controls on the autochthonous production and respiration of organic matter in cryoconite holes on high Arctic glaciers. J. Geophys. Res 117, G01017.
- Voisin, D., Jaffrezo, J. L., Houdier, S., Barret, M., Cozic, J. and co-authors. 2012. Carbonaceous species and humic like substances (HULIS) in Arctic snowpack during OASIS field campaign in Barrow. J. Geophys. Res. 117, D00R19. doi:10.1029/2011JD016612.
- Warren, S. G. and Wiscombe, W. J. 1980. A model for the spectral albedo of snow. II: snow containing atmospheric aerosols. J. Atmos. Sci. 37, 2734–2745. doi:10.1175/1520-0469(1980)037<2734:AMFTSA>2.0.CO;2
- Weishaar, J. L., Aiken, G. R., Bergamaschi, B. A., Fram, M. S., Fujii, R. and co-authors. 2003. Evaluation of specific ultraviolet absorbance as an indicator of the chemical composition and reactivity of dissolved organic carbon. Environ. Sci. Technol. 37, 4702–4708. doi:10.1021/es030360x
- Wernli, H. and Davies, H. C. 1997. A Lagrangian-based analysis of extratropical cyclones. I: the method and some applications. Q. J. Royal Met. Soc. 123, 467–489. doi:10.1002/qj.49712353811
- Yan, F., Kang, S., Li, C., Zhang, Y., Qin, X. and co-authors. 2016. Concentration, sources and light absorption characteristics of dissolved organic carbon on a medium-sized valley glacier, northern Tibetan Plateau. Cryosphere 10, 2611–2621. doi:10.5194/tc-10-2611-2016
- Zhang, Y., Kang, S., Cong, Z., Schmale, J., Sprenger, M. and co-authors. 2017. Light‐absorbing impurities enhance glacier albedo reduction in the southeastern Tibetan plateau. J. Geophys. Res. Atmos. 122, 6915–6933. doi:10.1002/2016JD026397
- Zhang, Y., Kang, S., Gao, T., Schmale, J., Liu, Y. and co-authors. 2019. Dissolved organic carbon in snow cover of the Chinese Altai Mountains, Central Asia: concentrations, sources and light-absorption properties. Sci. Total Environ. 647, 1385–1397. doi:10.1016/j.scitotenv.2018.07.417
- Zhang, Y., Kang, S., Li, G., Gao, T., Chen, P. and co-authors. 2018. Dissolved organic carbon in glaciers of the southeastern Tibetan Plateau: insights into concentrations and possible sources. PLoS One 13, e0205414. doi:10.1371/journal.pone.0205414
- Zhang, Y., Kang, S., Zhang, Q., Gao, T., Guo, J. and co-authors. 2016. Chemical records in snowpits from high altitude glaciers in the Tibetan Plateau and its surroundings. PLoS One. 11, e0155232. doi:10.1371/journal.pone.0155232
- Zhang, Y., Kang, S., Zhang, Q., Grigholm, B., Kaspari, S. and co-authors. 2015. A 500 year atmospheric dust deposition retrieved from a Mt. Geladaindong ice core in the central Tibetan Plateau. Atmos. Res. 166, 1–9. doi:10.1016/j.atmosres.2015.06.007